DOI:
10.1039/C5QO00326A
(Research Article)
Org. Chem. Front., 2016,
3, 53-61
Fluorescent nanoassemblies between tetraphenylethenes and sulfonatocalixarenes: a systematic study of calixarene-induced aggregation†
Received
23rd October 2015
, Accepted 11th November 2015
First published on 13th November 2015
Abstract
In recent years, various stimulus-responsive nanoarchitectures have been fabricated by virtue of the calixarene-induced aggregation (CIA) strategy by our group and others, displaying functional applications of controlled release and catalysis. Herein, we aimed to demonstrate a systematic study of CIA that how and to what extent the structures of hosts and guests affect the assembly behavior. Tetraphenylethene (TPE) analogues were employed as model substrates due to their feature of aggregation-induced emission, which converts aggregation to easily observable fluorescence signals. As a result, the complexation-induced aggregation of TPE guests by macrocyclic hosts was conveniently monitored by fluorescence spectroscopy. Three typical macrocyclic hosts, cyclodextrins, cucurbiturils and p-sulfonatocalixarenes, were engaged in the complexation-induced aggregation of TPE guests. The obtained results show that the preorganized cyclic scaffold and the cavity binding capability of p-sulfonatocalixarenes play a crucial role in CIA performance, besides the well-accepted charge compensation. To generalize the feature of CIA, we further studied the complexation-induced aggregation of TPE guests by heparin, a model polyanion that forms polyion complex micelles with cationic substrates. The similarities and differences between CIA assemblies and polyion complex micelles were claimed.
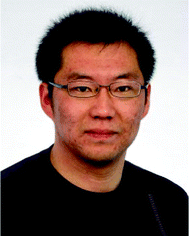 Dong-Sheng Guo | Dong-Sheng Guo was born in Tangshan, China in 1979. He obtained his Ph.D. degree in 2006 from Nankai University under the guidance of Professor Yu Liu and then joined Professor Liu's group as a faculty member. He was promoted as an Associate Professor in 2008, and became full Professor in 2013. His current research interest is calixarene-based supramolecular chemistry. |
Introduction
Self-assembly, in brief, is the science in which things put themselves together via reversible non-covalent interactions.1 Many important and interesting phenomena in nature are related to self-assembly.2 Inspired by nature, scientists in diverse fields have strived for establishing artificial self-assembly systems. Thanks to the dynamic characteristics of non-covalent forces, self-assembling materials3 provide benign performances of reversible response,4–6 self-healing,7–10 self-adaption,11 and error correction.12,13 In this regard, developing novel self-assembling strategies are highly desired to design well-defined supramolecular architectures14,15 and smart functional materials.16,17 For instance, amphiphilic assembly is related to many biological processes and widely used in many areas.18 However, not all amphiphilic molecules form the expected nano-architectures. Zhang and coworkers proposed the concept of “supra-amphiphiles” referring to amphiphiles that are constructed on the basis of non-covalent interactions or dynamic covalent bonds.19 In supra-amphiphiles, the functional groups can be easily attached to the amphiphiles without tedious covalent synthesis. They demonstrated a hierarchical self-assembly of nanoribbons using the strategy of supra-amphiphiles, where the self-assembling nanostructure can be tuned reversibly between single-layer and multilayer nanoribbons by the variation of pH.20 It is worth mentioning that hierarchical self-assembly is also a ubiquitous self-assembling strategy in nature.21 It has been a fascinating way to prepare highly ordered structures with a controllable complexity. Taking the advantage of hierarchical self-assembly, Yang and coworkers made a great contribution on various elegant functional architectures.22–25 On the other hand, orthogonal self-assembly, describing non-covalent interactions that do not interfere with each other directly,26 represents an alternative way to build self-assembling materials, especially supramolecular polymers.27,28 More recently, Liu and coworkers proposed the frame-guided assembly strategy.29 Single-stranded DNA functionalized gold nanoparticles were employed as the foundation of the frame, and then the other assembly units were directed to the corresponding position by DNA hybridization.30
Among all the non-covalent interactions, the electrostatic interaction shows advantages of being relatively strong and with long range, but the disadvantages of being non-specific and having non-directional nature.31 Ionic self-assembly is therefore always involved in a synergistic mechanism by integrating multiple interactions into one system. Shelnutt and coworkers prepared robust nanotubes through ionic self-assembly of two oppositely charged porphyrins, where electrostatic and π-stacking interactions were involved.32 By virtue of charge interactions, a new kind of polyion complex (PIC) micelles was developed by mixing ionic surfactants with oppositely charged polyelectrolytes.33
As part of our ongoing program on self-assembling materials based on macrocyclic hosts, we recently proposed a novel building strategy of calixarene-induced aggregation (CIA), where the complexation of p-sulfonatocalix[n]arenes (SCnAs) results in decreasing the critical aggregation concentrations (CACs) of guest molecules, improving the stability and compactness of aggregates, and regulating the assembly morphology in a well-defined manner.34 Until now, more than 30 guest molecules, including surfactants, fluorescent dyes, drugs, and biomacromolecules, have been employed in CIA by our group and others.35–41 Several kinds of self-assembling architectures were therefore fabricated, directed by diverse applications including drug delivery, catalysis, and optoelectronic materials.42
At a glance, the assembling model of CIA is somehow similar to that of PIC micelles.43 In this regard, García-Río and coworkers took SC6A as a small model molecule of polyanions and investigated the complexation-induced assembly behavior of cationic surfactants in detail, giving a better understanding of PIC micelles.44 We however envisaged that there should be some differences between SCnAs and polyelectrolytes in inducing aggregation. Obviously, the flexible polyelectrolytes show no special pre-organized structure and cavity binding behavior, and the electrostatic attraction is the only driving force to construct PIC micelles. Consequently, we herein investigated comprehensively the complexation-induced aggregation behavior by using different macrocycles (cyclodextrins, calixarenes and cucurbiturils), as well as heparin (a model polyanion) (Scheme 1), to get deep insight into CIA and disclose how and to what extent CIA differs from PIC. Tetraphenylethene (TPE) analogues were employed as model substrates (Scheme 2) due to their feature of aggregation-induced emission (AIE),45–47 which converts aggregation to easily observable fluorescence signals. We can therefore compare the complexation-induced aggregation behavior conveniently by monitoring the corresponding fluorescence.
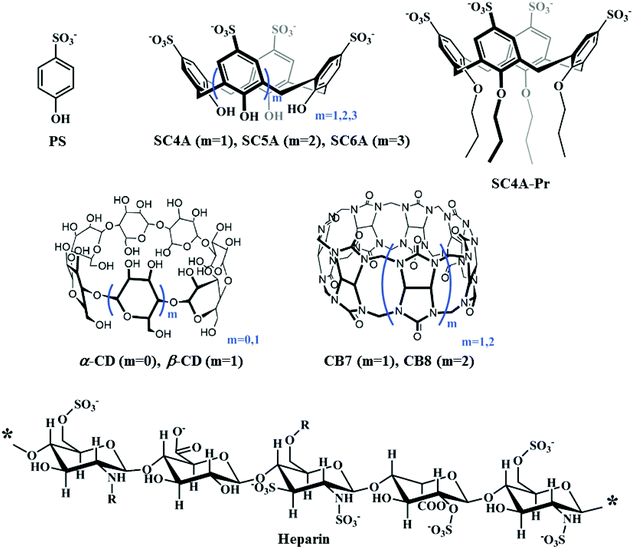 |
| Scheme 1 The chemical structures of 4-phenolsulfonic sodium (PS), SCnAs (n = 4, 5, 6), p-sulfonatocalix[4]arene tetrapropyl ether (SC4A-Pr), α,β-cyclodextrins (α-CD and β-CD), cucurbit[7,8]urils (CB7 and CB8), and heparin. | |
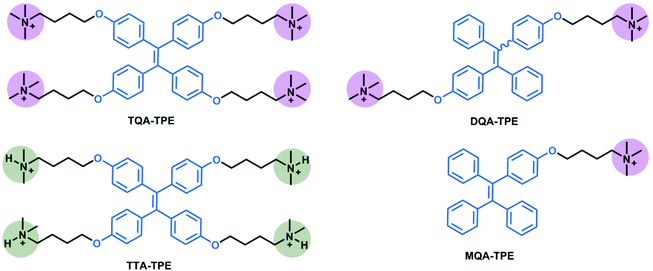 |
| Scheme 2 The chemical structures of TPE guests. | |
Results and discussion
Syntheses of TPE guests and their self-assembly properties
TPE analogues represent an outstanding family of AIE candidates due to their simple synthesis and good optical properties.48 AIE molecules overcome a significant drawback of traditional fluorescence dyes, aggregation-caused quenching. Their fluorescence is quenched by the intramolecular rotation.49 When forming aggregates, their stacking restricts the intramolecular rotation, leading to fluorescence increasing. Once reported by Tang's group,50 AIE has attracted great attention and has been applied in many areas such as in organic light-emitting diodes (OLEDs)51–53 and biosensors.54–56 In our present work, four organic ammonium-modified TPE derivatives were used, including 4,4′,4′′,4′′′-((ethene-1,1,2,2-tetrayltetrakis(benzene-4,1-diyl))tetrakis(oxy))tetrakis(N,N,N-trimethylbutan-1-aminium) (TQA-TPE), 4,4′-(((1,2-diphenylethene-1,2-diyl)bis(4,1-phenylene))bis(oxy))bis(N,N,N-trimethylbutan-1-aminium) (DQA-TPE), N,N,N-trimethyl-4-(4-(1,2,2-triphenylvinyl)phenoxy)butan-1-aminium (MQA-TPE), and 4,4′,4′′,4′′′-((ethene-1,1,2,2-tetrayltetrakis(benzene-4,1-diyl))tetrakis(oxy))tetrakis(N,N-dimethylbutan-1-aminium) (TTA-TPE). DQA-TPE, MQA-TPE and TQA-TPE were prepared according to our previous studies.57,58 TTA-TPE was prepared according to the synthetic route shown in Scheme 3. As a general procedure, tetramethoxy-TPE was prepared by McMurry coupling. After demethylation by BBr3, tetrahydroxy-TPE reacted with 1,4-dibromobutane to form tetrabromobutoxy-TPE. Finally, tetrabromobutoxy-TPE was refluxed with N(CH3)3 or NH(CH3)2 to obtain water-soluble TQA-TPE and TTA-TPE guests. MQA-TPE, DQA-TPE and TQA-TPE possess one, two and four quaternary ammonium end groups, respectively. The different numbers of positively charged end groups enable us to disclose the role of charge compensation in CIA. TQA-TPE and TTA-TPE are with different kinds of end groups, quaternary and ternary ammoniums. SCnAs afford distinguished binding affinities to these two end groups, providing us an opportunity to clarify the effect of binding capability on CIA.
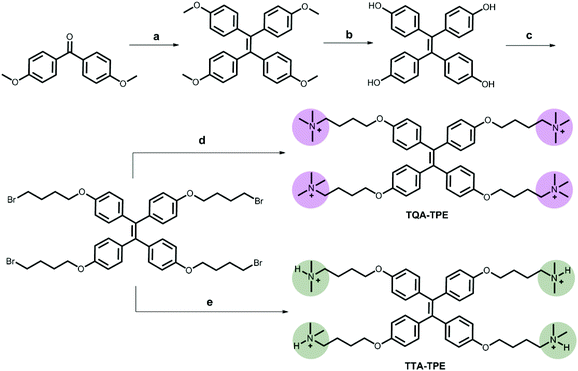 |
| Scheme 3 Synthetic routes of TQA-TPE and TTA-TPE. (a) TiCl4, Zn, THF, reflux, 20 h; (b) BBr3, CH2Cl2, r.t., overnight; (c) 1,4-dibromobutane, K2CO3, CH3CN, reflux, 30 h; (d) 33% N(CH3)3 aqueous solution, EtOH, reflux, 10 h; (e) 30% NH(CH3)2 ethanol solution, EtOH, reflux, 7 h. | |
Before studying the complexation-induced aggregation of TPE guests by macrocyclic hosts, we need to know the self-assembly behaviors of free TPE analogues. Taking TQA-TPE as an example, its CAC was monitored by dynamic light scattering (DLS). The scattering intensity of TQA-TPE solutions increases with the concentration of TQA-TPE (Fig. S4†). An inflection point was observed at 9.0 × 10−5 M, which was deemed as the CAC of TQA-TPE. At a concentration (1.2 × 10−4 M) above its CAC, TQA-TPE forms well-defined aggregates with an averaged hydrodynamic diameter of around 480 nm (Fig. S5†). However, no appreciable AIE fluorescence was observed. The result indicates that the aggregation is not compact enough to restrict the intramolecular rotation of the phenyl rings although free TQA-TPE is capable of forming large-sized aggregates above its CAC.
Self-assembly of TPE guests in the presence of different macrocyclic hosts
CDs, SCAs and CBs are three classical water-soluble macrocycles widely studied with respect to their ability to form host–guest complexes in aqueous media.59–61 As for assembly, CDs and CBs tend to form rotaxanes or catenanes62,63 because guest molecules tend to thread their cavities. As a result, self-aggregation of guest molecules is always prevented by CDs and CBs encapsulation.64–66 In contrast, SCnAs favor a more shallow inclusion. This is because the hydrogen-bonding network formed by the lower-rim phenolic hydroxyls leaves the bottom of calixarene cavity effectively closed, preventing guest threading. Taking the negatively charged feature into account, SCnAs can therefore promote the self-aggregation of positively charged aromatic or amphiphilic guests.34
We first compared the complexation-induced aggregation of the TPE guests by these three kinds of macrocyclic hosts (Fig. 1 and S9†). No appreciable AIE fluorescence was observed upon addition of α-CD, β-CD, CB7, or CB8 into the TPE solution (1.0 × 10−5 M, below the CAC of free TQA-TPE), indicating that CD and CB species do not have the tendency to induce aggregation of the TPE guests. It agrees well with previous reports that CDs and CBs always preclude guests from self-aggregation by threading.67,68 However, when adding SC4A into the TPE solution, pronounced AIE fluorescence which peaked at 475 nm was observed, ascribing to CIA.58 DLS measurement ensures the formation of large-sized aggregates by giving an average diameter of 143 nm (Fig. S7†). The CAC of TQA-TPE in the presence of SC4A was determined as 8.0 × 10−7 M by DLS (Fig. S6†), decreasing over 100 times compared with that of free TQA-TPE. All these results demonstrate obviously the CIA of the TPE guests, with decreased CAC value and enhanced compactness of the aggregate.
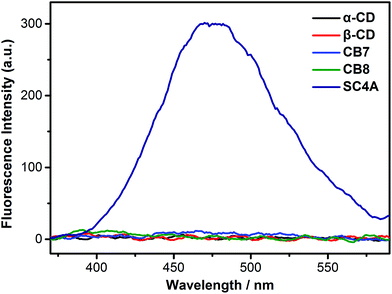 |
| Fig. 1 Fluorescence spectra of TQA-TPE (1.0 × 10−5 M) in the presence of α-CD (4.0 × 10−5 M), β-CD (4.0 × 10−5 M), CB7 (4.0 × 10−5 M), CB8 (4.0 × 10−5 M), SC4A (1.0 × 10−5 M) in water at 25 °C, λex = 320 nm. | |
Effect of the calixarene structures on CIA
With regard to the mechanism of CIA, we proposed a conjecture two-step process.34 First, the host and guest molecules form a simple complex as the transient core driven by the host–guest interaction. Thereafter, additional guest molecules are readily integrated into the core complex by charge, hydrophobic and π-stacking interactions, resulting in the formation of higher-order aggregates. Consequently, CIA is a result of a synergistic effect of diverse non-covalent interactions. Three key factors are generally assumed for high-performance CIA: (1) strong binding affinities between the SCnAs and the polar head groups of the guests, (2) charge compensation between the hosts and guests, and (3) the preorganized cyclic scaffold of the SCnAs. Moreover, addition of excess calixarene would lead to aggregate dispersion and a simple complex formed because the host–guest interaction overwhelms the others.
Although several studies on CIA have been performed by our group and others, it is still unclear how the SCnA structures affect the CIA performance. We herein compared the CIA of TQA-TPE by using SC4A, SC5A, SC6A, and SC4A-Pr. 4-Phenolsulfonic sodium (PS), the building subunit of SCnAs, was also employed as blank control. SC4A, SC5A and SC6A have distinguished conformational flexibilities, cavity sizes and sulfonate charge groups. SC4A-Pr is a lower-rim derivative of SC4A with propyl chains. As a result of lower-rim modification, SC4A-Pr assumes a “pinched-cone” conformation with more rigidification,69 and prefers to encapsulate planar guests instead of spherical guests.71
The fluorescence spectra of TQA-TPE upon addition of PS or various calixarenes are shown in Fig. 2. All calixarene species lead to the occurrence of AIE fluorescence of TPE, but even excess PS does not, which displays that the preorganized cyclic scaffold of a calixarene is prerequisite in CIA. SC4A, the smallest analogue with four negatively charged sulfonate groups, shows better CIA performance than the larger ones, SC5A and SC6A, indicating that there are other factors besides electrostatic interactions to control CIA. Both SC4A and SC5A possess the well-defined cone conformation held by the hydrogen-bonding network of lower-rim phenolic hydroxyls. However, SC4A exhibits much stronger binding capability to ternary ammonium than SC5A,70 and therefore, SC4A is expected to induce aggregation of TQA-TPE better than SC5A. The dominant influence of cavity binding capability on CIA was further validated by comparing the AIE fluorescence of TQA-TPE upon addition of SC4A and SC4A-Pr. SC4A-Pr, with the “pinched-cone” conformation, shows 2–3 orders of magnitudes weaker binding affinity to quaternary ammonium than SC4A,69,71 and consequently, SC4A-Pr cannot induce aggregation of TQA-TPE as well as SC4A.
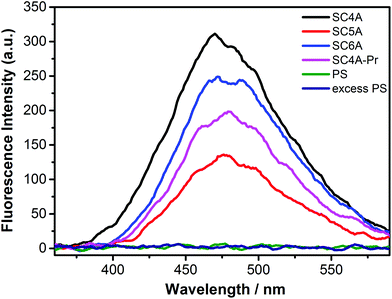 |
| Fig. 2 Fluorescence spectra of TQA-TPE (1.0 × 10−5 M) in the presence of SC4A, SC5A, SC6A, SC4A-Pr, PS at a stoichiometry of charge compensation and 10 times PS in water at 25 °C, λex = 320 nm. | |
As mentioned above, adding excess calixarene could result in the aggregate being dispersed. We further implemented several fluorescence titrations to get more insight into CIA. Upon a stepwise addition of calixarenes to the TQA-TPE solution (Fig. 3), the fluorescence of TQA-TPE increases sharply to a maximum at molar ratios around charge compensation, and then decreases gradually until reaching a plateau. The fluorescence decrease definitely demonstrates the disassembly of fluorescent nanoparticles. The ultimate fluorescence intensity upon addition of excess calixarene is somewhat indicative of the degree of disassembly, and the decreasing slope reflects the capability of excess calixarene to drive disassembly. Excess SC4A cuts down the AIE fluorescence to almost zero which means that vast majority of aggregates were destroyed. That is, in the presence of excess SC4A, the host–guest interaction overwhelms the others, resulting in the higher-order aggregate disassembled and the simple complex formed (Scheme 4). DLS measurements provide us another powerful evidence of the disassembly caused by excess SC4A (Fig. S7†). Mixing TQA-TPE with SC4A in 1
:
1 molar ratio generated a pronounced scattering intensity of large-sized assembly (ca. 143 nm) as mentioned above, whereas with excess SC4A in 1
:
9 ratio, the scattering signal disappeared.
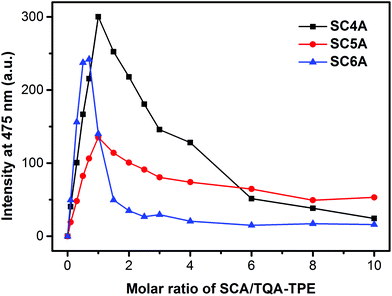 |
| Fig. 3 Fluorescence intensities of TQA-TPE (1.0 × 10−5 M) at 475 nm depended on gradual addition of SC4A, SC5A and SC6A. | |
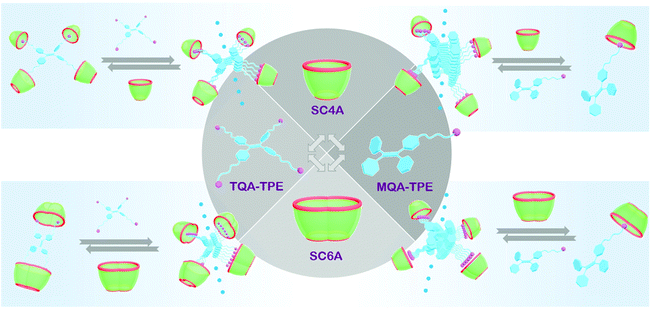 |
| Scheme 4 Schematic illustration of CIA of TQA-TPE/MQA-TPE by SCnAs (n = 4 and 6) and the disassembly by excess SCnAs. | |
The AIE fluorescence of TQA-TPE was merely quenched by half upon addition of excess SC5A, and the quenching slope is much more flat than that of SC4A. Both phenomena indicate the inferior disassembling ability of SC5A, originating from its relatively weak binding affinity to ternary ammonium. And also, anticipating excess SC5A forms the ultimate 4
:
1 complex with TQA-TPE (each quaternary ammonium end group was encapsulated into one SC5A cavity), there would be more steric hindrance and charge repulsion between SC5A molecules, which is unfavorable for the disassembly driven by host–guest interaction.
A dramatically sharp fluorescence quenching was observed upon addition of excess SC6A, and a definite inflection point appears at the SC6A/TQA-TPE molar ratio of 2
:
1. Since the larger analogue SC6A is not the best candidate for binding organic ammonium,59 the best disassembling performance directed by SC6A seems to be strange. The inflection point at the molar ratio of 2
:
1 is informative. That is, 2 equiv. SC6A is efficient enough to destroy the aggregate absolutely. If SC6A assumes the same disassembling model as SC4A, at least 4 equiv. SC6A is required to achieve the complete disassembly. However, the actual result indicates the formation of the ultimate 2
:
1 complex between SC6A and TQA-TPE, and therefore, we inferred the assembling and disassembling model as shown in Scheme 4. To verify the assumption of 2
:
1 complexation between SC6A and TQA-TPE, the aggregation and disassembly of MQA-TPE induced by SC6A were further conducted (Fig. 4). MQA-TPE is with only one quaternary ammonium modified. Upon addition of excess SC6A, the ultimate complex stoichiometry is expected to be 1
:
1 (Scheme 4), which is different from the 2
:
1 stoichiometry of TQA-TPE where two quaternary ammonium groups were encapsulated into one SC6A cavity. It is reasonably acceptable that SC6A forms a more stable complex with TQA-TPE than MQA-TPE owing to the multivalency effect. Consequently, both the induced-aggregation and disassembly performances of MQA-TPE are much lower than those of TQA-TPE.
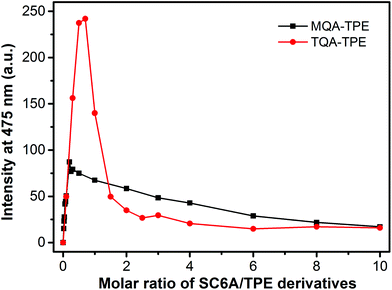 |
| Fig. 4 Fluorescence intensities of TQA-TPE (1.0 × 10−5 M) and MQA-TPE (1.0 × 10−5 M) at 475 nm depended on gradual addition of SC6A. | |
Effect of the end groups of TPE guests on CIA
In this section, we studied the effect of the end groups of TPE guests on CIA. TQA-TPE, DQA-TPE and MQA-TPE were compared as they possess different numbers of quaternary ammoniums. As can be seen in Fig. 5, all three guests display AIE fluorescence induced by SC4A. Their different emission intensities imply the varying degrees of aggregation, descending in the order of TQA-TPE > DQA-TPE > MQA-TPE. This tendency is involved in electrostatic interaction. When free TPE analogues assemble in water, there are two kinds of interactions. On the one hand, hydrophobic and π⋯π stacking interactions between TPE backbones favor the aggregation. On the other hand, electrostatic repulsion between the positively charged end groups prevents the aggregation. Upon complexation with SC4A, electrostatic repulsion between the end groups is replaced by electrostatic attraction between the quaternary ammonium groups and the sulfonate groups of the SC4A, and this attraction facilitates TPE guest aggregation. It is reasonably acceptable that the more electrostatic attraction, the better CIA performance.
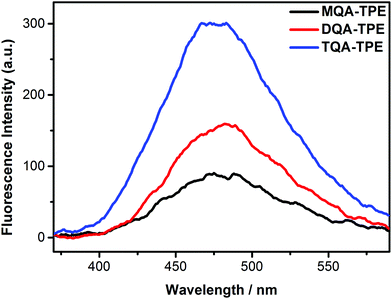 |
| Fig. 5 Fluorescence spectra of TQA-TPE (1.0 × 10−5 M), DQA-TPE (1.0 × 10−5 M), MQA-TPE (1.0 × 10−5 M) in the presence of SC4A at a charge-compensation stoichiometry in water at 25 °C, λex = 320 nm. | |
TQA-TPE and TTA-TPE both bear four positive charges, but with different kinds of end groups (quaternary ammonium and ternary ammonium, respectively). The CIA performances between TQA-TPE and TTA-TPE were further compared. As shown in Fig. 6, TTA-TPE shows comparable AIE fluorescence induced by SC4A than TQA-TPE, but the quenching slope of TTA-TPE is more flat than that of TQA-TPE. The inferior disassembly ascribes to the distinguished binding affinities of ternary and binary ammoniums with SC4A. As is well-known, C–H⋯π interaction is one of the crucial driving forces to generate complexes between calixarenes and guests. In comparison with ternary ammonium, binary ammonium has one less methyl group. SC4A therefore forms a less stable complex with binary ammonium than with ternary ammonium,70 resulting from less C−H⋯π interactions. The weaker cavity binding affinity leads to the poorer disassembling effect upon addition of excess SC4A.
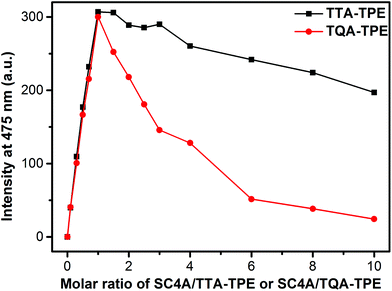 |
| Fig. 6 Fluorescence intensities of TQA-TPE (1.0 × 10−5 M) and TTA-TPE (1.0 × 10−5 M) at 475 nm depended on gradual addition of SC4A. | |
Comparison between CIA and PIC
Heparin, a highly sulfated glycosaminoglycan, has the highest negative charge density compared to any known biological molecule. Herein, the AIE fluorescence of TQA-TPE induced by SC4A and heparin was compared to reveal the similarities and differences between CIA and PIC. The fluorescence spectra of TQA-TPE in the presence of SC4A and heparin were shown in Fig. 7, and pronounced AIE fluorescence was observed in both cases. Heparin is a polymer with average molecular weight of 3–40 kDa,72 its each repetitive disaccharides unit possesses 3–4 negative charges.73 Assuming a PIC model, SC4A should present a much weaker induced-aggregation capability than heparin because of less efficient charge interaction. However, the AIE fluorescence of TQA-TPE induced by SC4A is still slightly stronger than that induced by heparin.
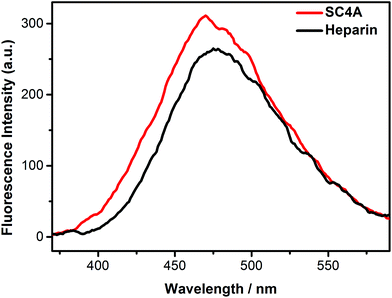 |
| Fig. 7 Fluorescence spectra of TQA-TPE (1.0 × 10−5 M) in the presence of SC4A and heparin at a charge-compensation stoichiometry in water at 25 °C, λex = 320 nm. | |
The definite difference between CIA and PIC is to compare the disassembling process when excess SC4A or heparin was added. As shown in Fig. 8, excess SC4A diminishes the AIE fluorescence drastically, while excess heparin almost does not. Heparin is a flexible polymer without any preorganized structure. As a result, there is no special “host–guest interaction” contributing to the induced aggregation besides electrostatic attraction. In the case of CIA, the aggregation was dispersed by excess SC4A, where the host–guest interaction overwhelms the others. In this regard, in the case of PIC, excess heparin is incapable of disassembling the aggregation. DLS measurements further support our standpoint (Fig. S8†). Mixing heparin with TQA-TPE in both 1
:
1 and 9
:
1 charge stoichiometries generated similarly pronounced scattering intensity of large-sized assembly.
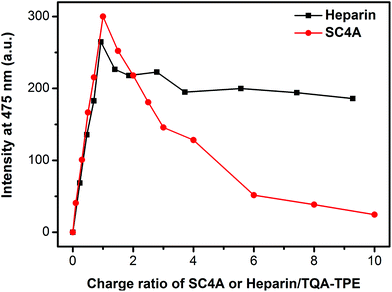 |
| Fig. 8 Fluorescence intensities of TQA-TPE (1.0 × 10−5 M) at 475 nm depended on gradual addition of SC4A and heparin. | |
Conclusion
In summary, we studied the complexation-induced aggregation of TPE guests with various hosts, including CDs, CBs, SCnAs, PS, and heparin, by monitoring the AIE fluorescence. The obtained results give a deep insight into CIA. The preorganized cyclic framework of SCnAs is prerequisite for CIA, whereas excess PS, the building subunit of SCnAs, does not show any tendency of inducing aggregation. Both host–guest and electrostatic interactions contribute synergistically to CIA, which offsets the intrinsic charge repulsion during the course of self-aggregation of cationic substrates, and thereby, leads to the formation of large-sized aggregates. As cavity binding is one of the dominant driving forces, the structures of SCnAs, such as cyclic size, conformation, and flexibility exert extraordinary influence on the performance of CIA. Among various SCnAs employed in this work, SC4A exhibits the best CIA performance benefiting from its strongest binding capability offered by its well-organized cone shape cavity. Furthermore, the similarities and differences between CIA and PIC were declared. Both of them are capable of inducing aggregation, leading to the formation of large-sized aggregates bellowing the CAC of guests. Electrostatic interaction plays a great role in both processes. The definite difference between CIA and PIC is the host–guest interaction. The polyions are flexible and show no special preorganized structure, and therefore, special cavity binding could not be observed in PIC. A clear phenomenon to differentiate CIA from PIC is whether the aggregates disassemble upon addition of excess SCnAs or polyions. In the presence of excess SCnAs, the host–guest interaction overwhelms the electrostatic and π-stacking interactions, which degrades higher-order aggregates to simple complexes. If the host–guest interaction between calixarene and the guest is weak, CIA displays more features of PIC, in which the electrostatic interaction plays the crucial role, and no disassembly could be observed.
Acknowledgements
This work was supported by NSFC (21172119 and 21322207), the Fundamental Research Funds for the Central Universities and National University Student Innovation Program (201410055098), which are gratefully acknowledged.
Notes and references
-
J. A. Pelesko, in Self-assembly: The science of things that put themselves together, Chapman & Hall/CRC, 2007, pp. 1–13 Search PubMed.
- G. M. Whitesides and B. Grzybowski, Science, 2002, 295, 2418 CrossRef CAS PubMed.
- X. Ji, Y. Yao, J. Li, X. Yan and F. Huang, J. Am. Chem. Soc., 2013, 135, 74 CrossRef CAS PubMed.
- R. J. Wojtecki, M. A. Meador and S. J. Rowan, Nat. Mater., 2011, 10, 14 CrossRef CAS PubMed.
- D. J. Ahn, S. Lee and J.-M. Kim, Adv. Funct. Mater., 2009, 19, 1483 CrossRef CAS.
- S. Dong, Y. Luo, X. Yan, B. Zheng, X. Ding, Y. Yu, Z. Ma, Q. Zhao and F. Huang, Angew. Chem., Int. Ed., 2011, 50, 1905 CrossRef CAS PubMed.
- Z. Wei, J. H. Yang, J. Zhou, F. Xu, M. Zrínyi, P. H. Dussault, Y. Osadag and Y. M. Chen, Chem. Soc. Rev., 2014, 43, 8114 RSC.
- X. Yang, H. Yu, L. Wang, R. Tong, M. Akram, Y. Chen and X. Zhai, Soft Matter, 2015, 11, 1242 RSC.
- N. Roy, B. Bruchmann and J.-M. Lehn, Chem. Soc. Rev., 2015, 44, 3786 RSC.
- M. Zhang, D. Xu, X. Yan, J. Chen, S. Dong, B. Zheng and F. Huang, Angew. Chem., Int. Ed., 2012, 51, 7011 CrossRef CAS PubMed.
- J.-M. Lehn, Angew. Chem., Int. Ed., 2013, 52, 2836 CrossRef CAS PubMed.
- P. T. Corbett, J. Leclaire, L. Vial, K. R. West, J.-L. Wietor, J. K. M. Sanders and S. Otto, Chem. Rev., 2006, 106, 3652 CrossRef CAS.
- N. Sreenivasachary and J.-M. Lehn, Proc. Natl. Acad. Sci. U. S. A., 2005, 102, 5938 CrossRef CAS PubMed.
- P. J. Stang and B. Olenyuk, Acc. Chem. Res., 1997, 30, 502 CrossRef CAS.
- G. Wu, P. Verwilst, K. Liu, M. Smet, C. F. J. Faul and X. Hang, Chem. Sci., 2013, 4, 4486 RSC.
- J.-M. Lehn, Angew. Chem., Int. Ed., 2015, 54, 3276 CrossRef CAS PubMed.
- A. Grinthal and J. Aizenberg, Chem. Soc. Rev., 2013, 42, 7072 RSC.
- G. Wang, Y. Kang, B. Tang and X. Zhang, Langmuir, 2015, 31, 120 CrossRef CAS PubMed.
- Y. Kang, K. Liu and X. Zhang, Langmuir, 2014, 30, 5989 CrossRef CAS PubMed.
- C. Wang, Y.-S. Guo, Z.-Q. Wang and X. Zhang, Langmuir, 2010, 26, 14509 CrossRef CAS PubMed.
- P. Jonkheijm, P. Van der Schoot, A. P. H. J. Schenning and E. W. Meijer, Science, 2006, 313, 80 CrossRef CAS PubMed.
- G.-Z. Zhao, L.-J. Chen, W. Wang, J. Zhang, G. Yang, D.-X. Wang, Y. Yu and H.-B. Yang, Chem. – Eur. J., 2013, 19, 10094 CrossRef CAS PubMed.
- L.-J. Chen, Y.-Y. Ren, N.-W. Wu, B. Sun, J.-Q. Ma, L. Zhang, H. Tan, M. Liu, X. Li and H.-B. Yang, J. Am. Chem. Soc., 2015, 137, 11725 CrossRef CAS PubMed.
- J. Zhang, R. Marega, L.-J. Chen, N.-W. Wu, X.-D. Xu, D. C. Muddiman, D. Bonifazi and H.-B. Yang, Chem. – Asian J., 2014, 9, 2928 CrossRef CAS PubMed.
- N.-W. Wu, L.-J. Chen, C. Wang, Y.-Y. Ren, X. Li, L. Xu and H.-B. Yang, Chem. Commun., 2014, 50, 4231 RSC.
- H. Hofmeier and U. S. Schubert, Chem. Commun., 2005, 2423 RSC.
- S.-L. Li, T.-X. Xiao, C. Lin and L.-Y. Wang, Chem. Soc. Rev., 2012, 41, 5950 RSC.
- Q. Wang, M. Cheng, Y. Zhao, L. Wu, J.-L. Jiang, L.-Y. Wang and Y. Pan, Chem. Commun., 2015, 51, 3623 RSC.
- Y. Dong, Y. Sun, L. Wang, D. Wang, T. Zhou, Z. Yang, Z. Chen, Q. Wang, Q. Fan and D. Liu, Angew. Chem., Int. Ed., 2014, 53, 2607 CrossRef CAS PubMed.
- Z. Zhao, C. Chen, Y. Dong, Z. Yang, Q. Fan and D. Liu, Angew. Chem., Int. Ed., 2014, 53, 13468 CrossRef CAS PubMed.
- C. F. J. Faul and M. Antonietti, Adv. Mater., 2003, 15, 673 CrossRef CAS.
- Z.-C. Wang, C. J. Medforth and J. A. Shelnutt, J. Am. Chem. Soc., 2004, 126, 15954 CrossRef CAS PubMed.
- A. Kishimura, Polym. J., 2013, 45, 892 CrossRef CAS.
- D.-S. Guo and Y. Liu, Acc. Chem. Res., 2014, 47, 1925 CrossRef CAS PubMed.
- X.-Y. Hu, Y. Chen and Y. Liu, Chin. Chem. Lett., 2015, 26, 862 CrossRef CAS.
- Y.-X. Wang, D.-S. Guo, Y.-C. Duan, Y.-J. Wang and Y. Liu, Sci. Rep., 2015, 5, 9019 CrossRef CAS PubMed.
- S. Peng, K. Wang, D.-S. Guo and Y. Liu, Soft Matter, 2015, 11, 290 RSC.
- K. Wang, D.-S. Guo, X. Wang and Y. Liu, ACS Nano, 2011, 5, 2880 CrossRef CAS PubMed.
- N. Basilio, V. Francisco and L. García-Río, Int. J. Mol. Sci., 2013, 14, 3140 CrossRef CAS PubMed.
- V. Wintgens, Z. Miskolczy, J.-M. Guigner, C. Amiel, J. G. Harangozó and L. Biczók, Langmuir, 2015, 31, 6655 CrossRef CAS PubMed.
- V. Wintgens, C. L. Coeur, C. Amiel, J.-M. Guigner, J. G. Harangozó, Z. Miskolczy and L. Biczók, Langmuir, 2013, 29, 7682 CrossRef CAS PubMed.
- D.-S. Guo, K. Wang, Y.-X. Wang and Y. Liu, J. Am. Chem. Soc., 2012, 134, 10244 CrossRef CAS PubMed.
- Y. Kang, C. Wang, K. Liu, Z. Wang and X. Zhang, Langmuir, 2012, 28, 14562 CrossRef CAS PubMed.
- N. Basilio, B. Gmez, L. García-Río and V. Francisco, Chem. – Eur. J., 2013, 19, 4570 CrossRef CAS PubMed.
- J. Luo, Z. Xie, J. W. Y. Lam, L. Cheng, H. Chen, C. Qiu, H. S. Kwok, X. Zhan, Y. Liu, D. Zhu and B.-Z. Tang, Chem. Commun., 2001, 1740 RSC . Recently, the underlying light-emitting is ascribed to the restriction of intramolecular motion (rotation and vibration), please also see ref. 46 and 47.
- R. Zhang, R. T. K. Kwok, B. Z. Tang and B. Liu, RSC Adv., 2015, 5, 28332 RSC.
- J. Mei, Y. Hong, J. W. Y. Lam, A. Qin, Y. Tang and B. Z. Tang, Adv. Mater., 2014, 26, 5429 CrossRef CAS PubMed.
- D. Ding, K. Li, B. Liu and B. Z. Tang, Acc. Chem. Res., 2013, 46, 2441 CrossRef CAS PubMed.
- D. A. Shultz and M. A. Fox, J. Am. Chem. Soc., 1989, 111, 6311 CrossRef CAS.
- Y. Dong, J. W. Y. Lam, A. Qin, J. Liu, Z. Li, B.-Z. Tang, J. Sun and H. S. Kwok, Appl. Phys. Lett., 2007, 91, 011111 CrossRef.
- W. Z. Yuan, Y. Gong, S. Chen, X. Y. Shen, J. W. Y. Lam, P. Lu, Y. Lu, Z. Wang, R. Hu, N. Xie, H. S. Kwok, Y. Zhang, J. Z. Sun and B. Z. Tang, Chem. Mater., 2012, 24, 1518 CrossRef CAS.
- M. P. Aldred, C. Li, G.-F. Zhang, W.-L. Gong, A. D. Q. Li, Y. Dai, D. Ma and M.-Q. Zhu, J. Mater. Chem., 2012, 22, 7515 RSC.
- J. Huang, X. Yang, J. Wang, C. Zhong, L. Wang, J. Qin and Z. Li, J. Mater. Chem., 2012, 22, 2478 RSC.
- S. Chen, Y. Hong, Y. Liu, J. Liu, C. W. T. Leung, M. Li, R. T. K. Kwok, E. Zhao, J. W. Y. Lam, Y. Yu and B. Z. Tang, J. Am. Chem. Soc., 2013, 135, 4926 CrossRef CAS PubMed.
- W. Chen, Q. Li, W. Zheng, F. Hu, G. Zhang, Z. Wang, D. Zhang and X. Jiang, Angew. Chem., Int. Ed., 2014, 53, 13734 CrossRef CAS PubMed.
- T. Noguchi, T. Shiraki, A. Dawn, Y. Tsuchiya, L. T. N. Lien, T. Yamamoto and S. Shinkai, Chem. Commun., 2012, 48, 8090 RSC.
- Q. Zhang, Y.-C. Liu, D.-M. Kong and D.-S. Guo, Chem. – Eur. J., 2015, 21, 13253 CrossRef CAS PubMed.
- B.-P. Jiang, D.-S. Guo, Y.-C. Liu, K.-P. Wang and Y. Liu, ACS Nano, 2014, 8, 1609 CrossRef CAS PubMed.
- D.-S. Guo, K. Wang and Yu Liu, J. Incl. Phenom. Macrocycl. Chem., 2008, 62, 1 CrossRef CAS.
- S. O. Fakayode, M. Lowry, K. A. Fletcher, X. D. Huang, A. M. Powe and I. M. Warner, Curr. Anal. Chem., 2007, 3, 171 CrossRef CAS.
- I. W. Wymana and D. H. Macartney, Org. Biomol. Chem., 2010, 8, 253 Search PubMed.
- K. Kim, Chem. Soc. Rev., 2002, 31, 96 RSC.
- A. Harada, Acc. Chem. Res., 2001, 34, 456 CrossRef CAS PubMed.
- C. Hu, Y. Lan, F. Tian, K. R. West and O. A. Scherman, Langmuir, 2014, 30, 10926 CrossRef CAS PubMed.
- S. T. J. Ryan, J. D. Barrio, I. Ghosh, F. Biedermann, A. I. Lazar, Y. Lan, R. J. Coulston, W. M. Nau and O. A. Scherman, J. Am. Chem. Soc., 2014, 136, 9053 CrossRef CAS PubMed.
- L. Jiang, M. Deng, Y. Wang, D. Liang, Y. Yan and J. Huang, J. Phys. Chem. B, 2009, 113, 7498 CrossRef CAS PubMed.
- J. Yin, C. Chi and J. Wu, Chem. – Eur. J., 2009, 15, 6050 CrossRef CAS PubMed.
- T. Girek, J. Inclusion Phenom. Macrocyclic Chem., 2013, 76, 237 CrossRef CAS.
- X.-Y. Hu, S. Peng, D.-S. Guo and Y. Liu, Supramol. Chem., 2015, 27, 336 CrossRef CAS.
- Due to the steric effect of TQA-TPE guest, the ternary ammonium is more appropriate as a binding model to compare the binding affinities of SCnAs, KS of SC4A = 2.94 × 105 M−1 and KS of SC5A = 1.56 × 104 M−1. In the case of TTA-TPE, binary ammonium was chosen as the binding model, KS of SC4A = 5.93 × 104 M−1. These data are quoted from the master thesis of Xiao-Ning Gao, Nankai University, 2015.
- J. Cui, V. D. Uzunova, D.-S. Guo, K. Wang, W. M. Nau and Y. Liu, Eur. J. Org. Chem., 2010, 9, 1704 CrossRef.
- V. Ruiz-Calero, J. Saurina, M. T. Galceran, S. Hernández-Cassoua and L. Puignou, Analyst, 2000, 125, 933 RSC.
- S. Zhang, N. Li, F. Zhao, K. Li and S. Tong, Spctrochim. Acta A, 2002, 58, 273 CrossRef.
Footnote |
† Electronic supplementary information (ESI) available. See DOI: 10.1039/c5qo00326a |
|
This journal is © the Partner Organisations 2016 |
Click here to see how this site uses Cookies. View our privacy policy here.