DOI:
10.1039/C5PY01022E
(Paper)
Polym. Chem., 2016,
7, 89-100
Rheological behavior and molecular relaxation of polycations with macrocounterions in aqueous solutions†
Received
2nd July 2015
, Accepted 10th October 2015
First published on 12th October 2015
Abstract
Counterions play significant roles in the characteristics of polyelectrolytes, whereas many problems still remain unsolved. Among most previous research studies, counterions were too small to be “seen” by light scattering. In this article, we prepare macrocounterions which are single/double carboxylate ion-terminated polyethylene glycols (Mn ≈ 5000 g mol−1). The solution properties of polycations with macrocounterions are investigated through rheological, conductivity and dynamic light scattering measurement. On the basis of the scaling theory, the static and dynamic parameters of polyelectrolytes are analyzed over wide concentration ranges. In rheological measurement, a critical concentration (
) has been observed. When the concentration is below
, the role of macrocounterions can be ignored; while above
, macrocounterions determine the longest relaxation and prevent entanglement of polycations. Moreover, the same turning point exists in the dependence of conductivity and viscosity on the molar fraction of macrocounterions, which indicates that macrocounterions can't serve as brushes condensed on the polycation until their molar fraction is above the effective charge density of polyelectrolytes. In dynamic light scattering, we investigate the relaxation of polycations with macrocounterions on the length scale of electrostatic blobs, where the size of macrocounterions becomes significant. In comparison with ordinary polyelectrolytes, it is observed that both fast and slow diffusive relaxations of polycations with macrocounterions are significantly slowed down, and the fast mode relaxation becomes dominant. Since no independent relaxation of macrocounterions has been observed, it is supposed that the fast mode relaxation is the coupled relaxation of polycations and macrocounterions.
1. Introduction
Polyelectrolytes, consisted of polyions and multiple counterions, are considered to be among the least understood condensed matters due to the complex long-range electrostatic interaction and the multi-component system. In particular, counterions can influence the static structure and dynamics of polyelectrolytes dramatically.1–5 Interestingly, strong electrostatic interaction between polyions and counterions of high charge density has been observed, including counterions of multivalent ions, oppositely charged polyelectrolytes and polyzwitterions. For example, multivalent counterions can cause like-charged polyelectrolytes to condense into compact ordered states, e.g. DNA toroids and F-actin bundles;6–8 oppositely charged polyelectrolytes can form complexes which can be utilized in layer-by-layer assembly,9 high-performance membranes10 and hydrogels of extremely high mechanical strength;11 polyzwitterions exhibit anti-polyelectrolyte properties because their counterions are covalently bonded with polyions.12,13 In dynamic light scattering (DLS), polyelectrolytes have been observed to exhibit both fast and slow mode relaxations. Concerning the fast mode relaxation, it is supposed to be the coupling movement of the polyion with counterions.4,14,15 Matsuoka et al.4 found that the fast mode follows the order of Li+ < Na+ < Cs+ < H+ which is consistent with the ion mobility and hydration size, although the difference was small. For divalent counterions (Mg2+), the fast mode becomes slower and the slow mode becomes faster, in comparison with the monovalent counterion (Na+).14
Among the research studies in the past, counterions were too small to be “seen” in scattering measurements. Thus, a question arises: if the counterions are as large as visible in light scattering, how will such macrocounterions influence the conformation as well as dynamics of polyions? In this study, we prepared macrocounterions which are carboxylate ion-terminated poly(ethylene glycol) (PEG) of about 5000 g mol−1. As is well-known, PEG is a neutral polymer which exhibits good solubility in water.16 Therefore, the macrocounterions are able to dissolve in water readily, as opposed to ionic surfactants which will form micelles in water. Moreover, PEG bears diverse properties such as crystallization, solubility in organic solvents17 and good solvation of cations which are ideal for lithium ion batteries.18,19 Therefore, the long PEG tails of the macrocounterions could possibly endow polyelectrolytes with some novel properties. Concerning the high molecular weight of macrocounterions, it is supposed that (i) their conformation and relaxation behaviors can be detectable; (ii) their electrophoretic mobility will be much slower than those of small counterions; and (iii) the condensed macrocounterions can be considered as self-assembled brushes on polyions. In this article, both single and double carboxylate ion-terminated PEG were prepared. On the basis of the scaling theory, we first investigated the specific viscosity (ηsp), the longest relaxation time (τ) and the terminal modulus (G) of polycations with macrocounterions over wide concentration ranges through rheological measurement. Then dependence of solution conductivity on the molar fraction of macrocounterions is investigated. In addition, the diffusive relaxation of electrostatic blobs of polycations with macrocounterions in dilute solution is investigated by DLS.
2. Experimental section
2.1. Materials
Poly(ethylene glycol) (PEG, Mn = 4600 g mol−1) and poly(ethylene glycol) methyl ether (MPEG, Mn = 5000 g mol−1) were purchased from Aldrich, USA. Toluene (AR) and tetrahydrofuran (THF, AR) were purchased from SCRC, China and were distilled over sodium/benzophenone ketyl under a dry N2 atmosphere prior to use. Succinic anhydride (>95%), 4-dimethylaminopyridine (DMAP, >99%), triethylamine (>99%), methyl 2-chloropropionate (MCP, >95%) and (3-acrylamidopropyl)trimethylammonium chloride (ATP–Cl, 74–75% in water) were purchased from TCI, Japan. N,N-Dimethylformamide (DMF, AR), ammonium persulfate ((NH4)2S2O8, AR), sodium bisulfite (NaHSO3, AR) and sodium hydroxide (NaOH, AR) were purchased from SCRC, China and were used as received. Copper(I) chloride (CuCl, >97%) from Fluka, USA was washed with acetic acid followed by methanol to remove the impurities. Tris(2-dimethylaminoethyl)amine (Me6TREN, >99%) was purchased from Alfa Aesar, USA. Deuterium oxide (D2O, 99.9%) was purchased from TenglongWeibo Technology, China. Deuteriochloroform (CDCl3, 99.8%) was purchased from Cambridge Isotope Laboratories, Inc., USA. Ultrapure water with resistivity of 18.25 MΩ cm was produced by using a ULUPURE water purification system, China. Strong anion exchange resins D-201 were purchased from Aladdin, China.
2.2. Synthesis
2.2.1 Synthesis of carboxyl-terminated MPEG (MPC).
MPEG (45.40 g, 8.86 mmol) was dried by azeotropic distillation in 200 mL toluene at 135–140 °C. The dry MPEG, succinic anhydride (1.78 g 17.72 mmol), DMAP (1.08 g, 8.86 mmol) and triethylamine (123 μL, 0.89 mmol) were dissolved in 400 mL THF. The reaction was carried out at 20 °C under vigorous stirring. After 72 h, the solvent was evaporated completely using a rotary evaporator. The solid residue was dissolved in ultrapure water, and the precipitates were removed through pumping filtration. Then filtrate was dialyzed in dialysis tubes (cutoff molecular weight: 3500 g mol−1) against ultrapure water, until the conductivity of the emerging dialyzate is below 2 μs cm−1. Finally, the dialyzed solutions were dehydrated through lyophilization. 1H NMR (CDCl3, 500 MHz): 4.20 (t, COOCH2), 3.60 (m, OCH2CH2O), 3.33 (s, CH3O), 2.59 (m, OCOCH2CH2OCO).
2.2.2 Synthesis of carboxyl-terminated PEG (CPC).
The synthesis and purification of CPC was similar to that of MPC, with dry PEG (29.41 g, 6.31 mmol), succinic anhydride (2.52 g, 25.24 mmol), DMAP (1.54 g, 12.62 mmol) and triethylamine (177 μL, 1.26 mmol) dissolved in 300 mL THF. 1H NMR (CDCl3, 500 MHz): 4.24 (t, COOCH2), 3.66 (m, OCH2CH2O), 2.63 (m, OCOCH2CH2OCO).
2.2.3 Synthesis of PATP–OH.
2.2.3.1 Free radical polymerization.
Complete exchange of chloride ions (Cl−) into hydroxide ions (OH−) for monomers ATP–Cl (29.41 g, 106 mmol) was conducted by utilizing an excess amount of ionic exchange resin. After ion exchange, the elution (ATP–OH) was concentrated by rotary evaporation under vacuum pressure. 1 mmol (NH4)2S2O8 was added to 200 mL of ATP–OH aqueous solution at about 0.5 mol L−1. The mixture was degassed by bubbling nitrogen gas for 1 h. Then 0.1 mmol NaHSO3 were added to initiate the reaction. The reaction was carried out at 25 °C. After 20 h, the products were dialyzed in dialysis tubes (cutoff molecular weight: 14
000 g mol−1) against ultrapure water, until the conductivity of the emerging dialyzate was around 2 μs cm−1. Finally, the dialyzed polyelectrolyte solution (PATP–OH) was concentrated by rotary evaporation under vacuum pressure. The concentrated solution was stored in polyethylene bottles and its monomer concentration was 94.65 mmol L−1 calibrated by neutralization titration.
2.2.3.2 Atom transfer radical polymerization (ATRP).
In contrast to free radical polymerization mentioned above, ion exchange was conducted after ATRP synthesis to avoid precipitates of copper(I) hydroxide (CuOH) during the reaction. ATP–Cl (29.97 g, 0.11 mol) was dissolved in DMF/H2O (45 mL/37.5 mL) with [ATP–Cl] = 1.25 mol L−1. The molar ratio of the reagents was followed by [ATP–Cl]
:
[MCP]
:
[CuCl]
:
[Me6TREN] = 200
:
1
:
1
:
1. MCP (0.06 g), and Me6TREN (0.13 g) were added into the solution. Then the mixture was degassed by three freeze–pump–thaw cycles. The reaction was initiated by addition of CuCl (0.054 g) and continued for 1 h at 20 °C. After polymerization, the products underwent ion exchange to convert Cl− into OH− completely. The elution was purified in the same way as products of free radical polymerization. Its monomer concentration was 50.3 mmol L−1 calibrated by neutralization titration.
2.2.4 Preparation of polycations with macrocounterions.
Polycations with macrocounterions were prepared by the neutralization reaction of a PATP–OH storage solution with a certain molar fraction of MPC and CPC. The highest molar fraction of macrocounterions is 90 mol%, and the rest of the counterions are hydroxide ions (OH−) to avoid hydrolysis of carboxylate ions (COO−). Then the concentrated solutions were diluted to a series of concentrations. The storage solution was further concentrated to prepare samples of higher concentrations. All the solutions were stored in polyethylene bottles.
2.3. Characterization
The chemical structures of MPC and CPC were characterized by proton nuclear magnetic resonance (1H NMR) spectroscopy with a 500 MHz Bruker Advance III spectrometer (Germany). The weight-average molecular weight (Mw), number-average molecular weight (Mn) and polydispersity index (Mw/Mn) of polyelectrolytes were determined by gel permeation chromatography (GPC) of Waters 515 equipped with TOSOH BIOSEP G4000SWXL (7.8 × 300 mm, Japan) as hydrophilic columns. Near-monodisperse poly(ethyleneoxide) (PEO) standards were used for calibration. The eluent was 0.1 M NaNO3 aqueous solution at a flow rate of 1 mL min−1 at 40 °C.
2.4. Rheological measurements
An AR-G2 rheometer (TA Co., USA) was utilized for rheological measurement. A steel cone-plate geometry (40 mm diameter, 2° cone angle, gap 50 μm) was used in steady shear. The temperature was controlled at 20 °C by using a Peltier system.
2.5. Conductivity
Conductivity was measured by using a conductivity meter with conductivity cell lnlab@738, Mettler Toledo Co. The cell was calibrated using standard solutions with known conductivities. The temperature was controlled at 20 °C by using a water bath.
2.6. Laser light scattering
Dynamic light scattering was measured by using a commercial laser light scattering spectrometer (Brookhaven Instruments Corporation, USA) equipped with a digital autocorrelator (BI-9000AT) and a He–Ne laser (λ0 = 632.8 nm). All solutions were filtered with Millipore LCR 0.2 μm. The temperature was controlled at 20 °C and angular range for measurement was performed from 30° to 150°. The CONTIN method20 was used to analyze DLS data.
3. Results and discussion
Scheme 1 shows the chemical structures of polyethylene glycol (PEG), double carboxyl-terminated PEG (CPC), polyethylene glycol methyl (MPEG), carboxyl-terminated MPEG (MPC), poly((3-acrylamidopropyl)trimethylammonium hydroxide) (PATP–OH), and poly((3-acrylamidopropyl)trimethylammonium carboxylate ion-terminated MPC) (PATP–MPC). NMR characterization of MPC and CPC is shown in the ESI SI.1 and SI.2.†Table 1 shows the Mn, Mw and Mw/Mn of PATP–OH, (PATP_ATRP)–OH, PEG and MPEG. In particular, PATP–OH is synthesized by free radical polymerization, which presents high molecular weight and broad polydispersity. On the other hand, (PATP_ATRP)–OH is synthesized by an ATRP method, which exhibits much lower molecular weight and narrower polydispersity. The degree of polymerization of (PATP_ATRP)–OH is comparable to an electrostatic blob of PATP–OH, which will be discussed later.
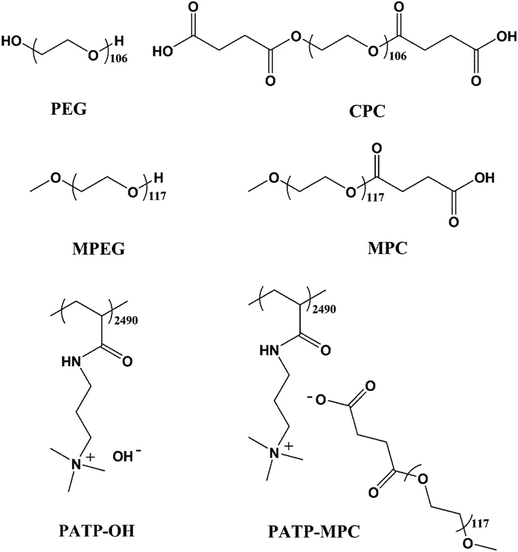 |
| Scheme 1 Chemical structures of PEG, CPC, MPEG, MPC, PATP–OH and PATP–MPC. | |
Table 1 Molecular weights, polydispersity index and degree of polymerization for samples
Sample |
M
n/g mol−1 |
M
w/g mol−1 |
M
w/Mn |
N
|
Calculated by dividing Mn by the monomer molecular weight.
Calculated by the NMR spectrum.
|
PATP–OH |
4.68 × 105 |
7.09 × 105 |
1.52 |
2490a |
(PATP_ATRP)–OH |
5.13 × 103 |
5.41 × 103 |
1.05 |
27a |
MPC |
5.22 × 103 |
— |
— |
117b |
CPC |
4.86 × 103 |
— |
— |
106b |
3.1. Theory background
There are four length scales which describe the molecular structure of polyelectrolytes in solution, including Bjerrum length (lB), electrostatic blob size (ξe), contour length (L) and correlation blob size (ξ).21–23 First, Bjerrum length (lB) is the length scale at which the electrostatic energy of two charges equals the thermal energy 1 kT:where e is the elementary charge, ε is the dielectric constant of the solvent, k is the Boltzmann constant, and T is the absolute temperature. According to Manning theory, the counterions will condense to the polyion when the distance between the nearby charged monomers becomes smaller than lB, as a result, the effective charge density f of the polyion decreases. Generally, for polyelectrolytes in water at 25 °C, lB ≈ 0.71 nm, and the effective charge density f ≈ b/lB ≈ 35%.23
Secondly, an electrostatic blob size (ξe) is the length scale at which the electrostatic energy inside the electrostatic blob equals 1 kT:
where
f is the effective charge density of polyelectrolytes,
ξe is the size of the electrostatic blob and
ge is the number of monomers inside the electrostatic blob. Inside the electrostatic blob, polyion monomers adopt a self-avoiding walk, like neutral polymers in good solvent:
where
b is the monomer size, and
b ≈ 0.25 nm for vinyl monomers in good solvent.
23
Considering the contour length (L) of a polyelectrolyte chain in dilute solution where no multi-chain interaction exists, L is considered as an array of electrostatic blobs:
| 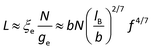 | (4) |
where
N is the degree of polymerization of polyelectrolytes.
Finally, the correlation blob size (ξ) describes the distance between the nearby chains when the concentration is over the critical overlap concentration c*. Based on the definition, c* is calculated by24
|  | (5) |
| 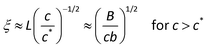 | (6) |
Inside the correlation blob, the polyelectrolyte segments behave like they are in the dilute solution, while outside the correlation blob, electrostatic interactions are screened and the conformation of the polyelectrolyte is a random walk of correlation blobs:
| R ≈ ξ(N/g)1/2 for c > c* | (7) |
where
R is the end-to-end distance of polyelectrolytes,
ξ is the correlation blob size and
g is the number of monomers inside the correlation blob.
Moreover, the stretching parameter B is defined as the ratio of the maximum possible contour length bN to the contour length L of the polyelectrolyte:
| B ≡ bN/L ≈ bN2/3(c*)1/3 | (8) |
Replacing the parameters in eqn (1)–(6) by B, it is calculated that
| 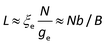 | (11) |
3.2. Rheology
Fig. 1 shows the dependence of specific viscosity (ηsp) on the normalized concentration (c/c*) for samples PATP–OH, PATP–MPC10%, PATP–MPC90%, PATP–CPC5% and PATP–CPC45%. In terms of nomenclature, PATP refers to the polycation, while OH, MPC and CPC refer to three types of counterions respectively, including hydroxide (OH−), single carboxylate ion-terminated MPEG (MPC−) and double carboxylate ion-terminated PEG (−CPC−). Moreover, the number in the nomenclature means the molar fraction of macrocounterions over all the counterions, and the rest of the counterions are OH−. Note that though the molar fraction of −CPC− is half that of MPC−, they contain the same amount of carboxylate ions (COO−). Concerning c*, it is determined by the concentration which corresponds to ηsp ≈ c/c* ≈ 1.23 In this article, concentration refers to the monomer concentration. It should also be mentioned that c/c* ≈ 400 is the highest concentration studied for PATP–MPC90% and PATP–CPC45% due to the maximum solubility of polyethylene glycol in water at 20 °C. In Fig. 1, ηsp of all the samples are well overlapped when c/c* < 7, whereas ηsp of PATP–MPC90% and PATP–CPC45% deviate and become another group at a higher concentration. Concerning the ordinary polyelectrolyte PATP–OH, it shows ηsp ∼ c0.97, ηsp ∼ c0.56 and ηsp ∼ c1.42 as the concentration increases, which is in agreement with the theoretical scaling relation for dilute, semidilute unentangled and entangled solutions respectively.21 In particular, entanglement of PATP–OH occurs at a concentration c/c* ≈ 100. The ηsp of PATP–MPC10% and PATP–CPC5% is close to that of PATP–OH, but entanglement occurs at a lower concentration and ηsp becomes slightly larger than that of PATP–OH in the entangled solution. It is possible that the small amounts of macrocounterions promote entanglement of the polycations. In contrast to PATP–OH, PATP–MPC10% and PATP–CPC5%, PATP–MPC90% and PATP–CPC45% exhibit ηsp ∼ c1.57 when c/c* > 7. Though the scaling exponent 1.57 resembles that for entangled polyelectrolytes, it does not mean that PATP–MPC90% and PATP–CPC45% have entangled. The static structure and dynamics of polycations with macrocounterions will be analyzed on the basis of the scaling theory.21–23
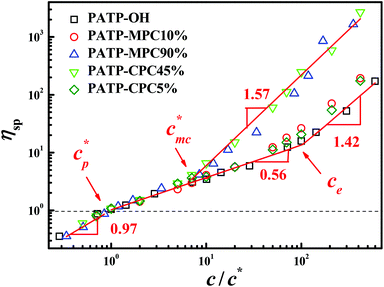 |
| Fig. 1 Dependence of specific viscosity (ηsp) on the normalized concentration (c/c*) for aqueous solutions of PATP–OH, PATP–MPC10%, PATP–MPC90%, PATP–CPC5% and PATP–CPC45%. ηsp = 1 is guided by the line. | |
Considering the fact that all the samples present similar ηsp when c/c* < 7, it means that the effect of macrocounterions can be ignored within this concentration region. Therefore, it is believed that the long-range electrostatic interaction is dominant, and the scaling approach to describe the conformation and dynamics of ordinary polyelectrolytes can still be applicable to polycations with macrocounterions for c/c* < 7. For instance, the contour length of polycations with macrocounterions can also be described as an array of electrostatic blobs in dilute solution and the polycations can be considered as the random walk of correlation blobs when 1 < c/c* < 7. Table 2 summarizes the static molecular parameters including the size (ξe) and number of monomers (ge) of the electrostatic blob, stretching parameter (B) and contour length (L) for PATP–OH, PATP–MPC10%, PATP–CPC5%, PATP–MPC90% and PATP–CPC45%, calculated by eqn (8)–(11). In Table 2, the stretching ratio B is 3.4–3.8, which indicates a highly stretched conformation due to the high charge density of the polycations. However, the slight difference in c* of all the samples needs further investigation. Scheme 2(a)–(c) illustrate the electrostatic blobs of PATP–OH, PATP–MPC90% and PATP–CPC45% respectively, accompanying their counterions in dilute solution. In Scheme 2(a), the electrostatic blob of ξe ≈ 1.4 nm and ge ≈ 20 is at the center, closely surrounded by condensed counterions (OH−), and the uncondensed OH− are relatively far away from the center. In Scheme 2(b) the electrostatic blob of PATP–MPC90% of ξe ≈ 1.4 nm and ge ≈ 18 is surrounded by brush-like macrocounterions (MPC−), with condensed and uncondensed MPC− at the inner and the outer space respectively. It is estimated that the end-to-end distance of MPC− is
, where N = 117, b = 0.25 nm and C∞ = 6.7 are respectively the degree of polymerization of MPC, monomer size and characteristic ratio for poly(ethylene oxide) in Θ solvent.24 Therefore, Rmc is about 5 times ξe, and the radius of the shell around the electrostatic blob is even larger due to the existence of uncondensed MPC−. In Scheme 2(c), for the electrostatic blob of PATP–CPC45% of ξe ≈ 1.9 nm and ge ≈ 28, there are condensed −CPC− close to electrostatic blobs and uncondensed −CPC− at the outer space of the electrostatic blobs. Note that the amount of −CPC− is half that of MPC− because of their double carboxylate ions. Moreover, concerning the double carboxylate ions of −CPC−, only intra-chain condensation will take place in dilute solution. Scheme 2(d) shows the single chain conformation of PATP–MPC90%. MPC− could serve as brushes which increase the rigidity of the polycation. Though the size of MPC− (Rmc) is about 5 times the ξe, the contour length of the polycation is much larger than Rmc, with the ratio Rmc/L ≈ 7 nm/194 nm ≈ 4%. In terms of topological structure, polycations with macrocounterions can be analogous to polymacromonomers, which contain high density of combs.25,26
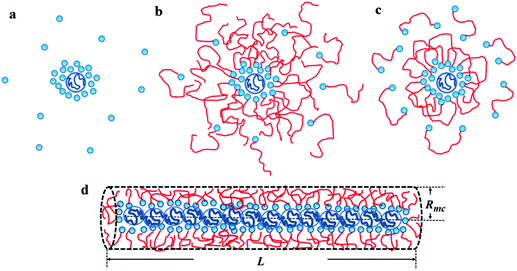 |
| Scheme 2 Illustrations of electrostatic blobs and counterions of (a) PATP–OH, (b) PATP–MPC90% and (c) PATP–CPC45%, and the single chain conformation of (d) PATP–MPC90% in dilute solution. The large blue circle at the center represents an electrostatic blob, the small blue circles around the electrostatic blob represent OH−, and the red tails with single/double blue circles at the ends represent MPC−/−CPC−. | |
Table 2 Molecular parameters of polyelectrolytes
Sample |
c*/mmol L−1 |
ξ
e/nm |
g
e
|
B
|
L/nm |
PATP–OH |
0.66 |
1.5 |
20 |
3.4 |
190 |
PATP–MPC90% |
0.56 |
1.4 |
18 |
3.2 |
194 |
PATP–CPC45% |
0.95 |
1.9 |
28 |
3.8 |
169 |
PATP–MPC10% |
0.95 |
1.9 |
28 |
3.8 |
169 |
PATP–CPC5% |
0.95 |
1.9 |
28 |
3.8 |
169 |
Fig. 2 shows the dependence of the longest relaxation time (τ) and the terminal modulus (G) on the normalized concentration (c/c*) for samples PATP–MPC90% and PATP–CPC45%. Here, τ and G refer to the relaxation time and modulus on the length scale of the whole chain, where τ is obtained by fitting the Carreau model and G is determined by G = η/τ.23Fig. 2(b) shows that G ∼ c1.17, which agrees with the scaling relation of unentangled polymers.22 In analogy to polymacromonomers whose dense brushes prevent entanglement of the linear backbone,25 the dense brush-like macrocounterions will also increase the rigidity of the polycations and prevent them from entanglement. In Fig. 2(a), τ presents two slopes as the concentration increases. At the beginning, the slopes of PATP–MPC90% and PATP–CPC45% are −0.6 and −0.58 respectively, close to the scaling exponent of ordinary polyelectrolytes (−0.5) in semidilute unentangled solutions. As c/c* further increases to 20 and 10 for PATP–MPC90% and PATP–CPC45% respectively, the slopes of τ change to 0.54 and 0.51 respectively. In comparison with the scaling relation of the relaxation of entangled polyelectrolytes (τ ∼ c0), the unique scaling relation of PATP–MPC90% and PATP–CPC45% (τ ∼ c0.5) indicates characteristic dynamics of polycations with macrocounterions rather than entanglement.
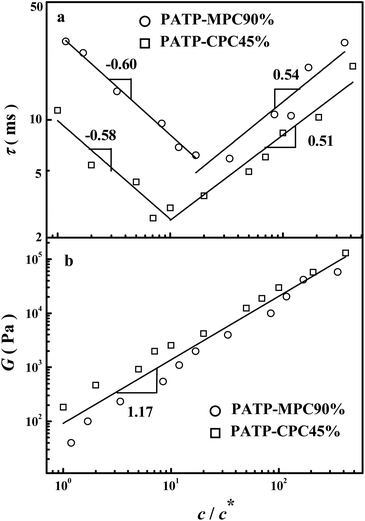 |
| Fig. 2 Dependence of the longest relaxation time (τ) and terminal modulus (G) on the normalized concentration (c/c*) for samples PATP–MPC90% and PATP–CPC45%. | |
Scheme 3 illustrates the random walk of correlation blobs of PATP–MPC90% when c/c* > 1, on the basis that the electrostatic interaction is dominant on the long length scale and therefore correlation blobs predicted for ordinary polyelectrolytes are applicable to polycations with macrocounterions. In analogy to comb polyelectrolytes, Papagiannopoulos and his coworkers27,28 confirmed that the correlation length of comb polyelectrolytes is independent of the comb architecture through small angle X-ray scattering (SAXS) measurement. But it should be noted that for comb polyelectrolytes above c*, their backbones and side chains are polyelectrolytes consisted of the same type of correlation blobs, while for polycations with macrocounterions, each correlation blob contains polycations surrounded by macrocounterions with neutral tails. In Scheme 3, the left part shows the random walk of correlation blobs. Each blob is of size ξ and contains g monomers of the polycation as well as g macrocounterions (MPC−). The conformation of polycation inside the correlation blob, represented by a thick blue line, is an array of electrostatic blobs of size ξe, and MPC− are represented by red circles whose size is Rmc. When the concentration is slightly above c*, the size of the correlation blob ξ is close to the contour length (L) of the polycation and ξ ≫ Rmc, similar to Scheme 2(d). Therefore, the effect of macrocounterions on the relaxation time of the correlation blob (τblob) can be ignored, and τblob follows the Zimm mode as ordinary polyelectrolytes:
where
ηs is the solvent viscosity. As the concentration further increases,
ξ decreases as
ξ ∼
c−1/2 and the longest relaxation time
τ also decreases as
τ ∼
c−1/2, as ordinary polyelectrolytes do.
22 However, once the concentration reaches a critical value
cc, it is supposed that the role of macrocounterions becomes dominant.
Scheme 4(a) shows a correlation blob at
cc, where
gc macrocounterions of volume
Rmc3 fill the space of the critical correlation blob
ξc3, namely
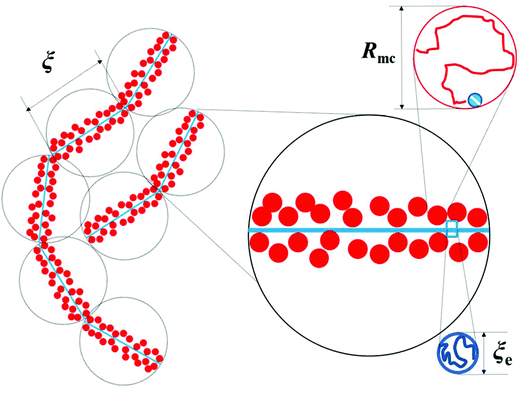 |
| Scheme 3 Illustration of correlation blobs of PATP–MPC90%. | |
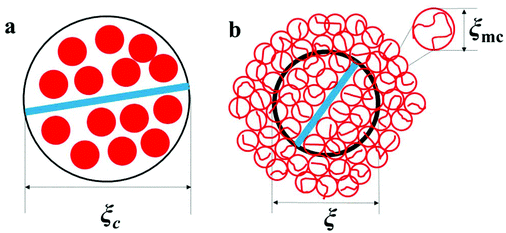 |
| Scheme 4 Illustration of a correlation blob of PATP–MPC90% at (a) the critical concentration cc and (b) above cc. | |
Concerning the definition of the critical overlap concentration, cc in fact equals the critical overlap concentration of macrocounterions (
). As shown in Scheme 4(b), when c > cc, macrocounterions become overlapped and each macrocounterion is the random walk of their own correlation blobs of size ξmc, represented by red circles. Inside ξmc, there are gmc macrocounterion monomers which relax in the Zimm mode, so the relaxation time of each blob (τblob_mc) is
| τblob\_mc ≈ ηsξmc3/kT | (14) |
The correlation blob of polycations is represented by the thick black circle of size ξ. Since macrocounterions serve as thick shells around the polycation, the polycation cannot relax until the macrocounterions around the polycation have relaxed. Therefore, the relaxation time of the correlation blob of polycations (τblob) is determined by the Rouse mode relaxation of multiple correlation blobs of macrocounterions. If one macrocounterion contains n monomers, each correlation blob of polycations contains (n/gmc)g correlation blobs of macrocounterions, so
| 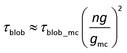 | (15) |
Concerning the relaxation of the whole chain of polycations with macrocounterions (τchain), it is the Rouse mode relaxation of N/g correlation blobs of polycations:
|  | (16) |
where for neutral polymers,
ν = 0.5 in Θ solvent, and
ν = 0.588 in good solvent, while for polyelectrolytes,
ν = 1.
22 Since the long tails of macrocounterions are neutral polyethylene glycol,
eqn (16) is calculated to be
τchain ∼
c1 in Θ solvent and
τchain ∼
c0.31 in good solvent. Therefore, the scaling exponent for relaxation time of polycations with macrocounterions will be in the range of 0.31–1, dependent on the solvent quality for macrocounterions. Experimentally, the scaling relation is
τchain ∼
c0.5, which is within the theoretical prediction. In essence, the relaxation time of polycations with macrocounterions is determined by relaxation of their macrocounterions in semidilute unentangled solution.
Next we compared the calculated cc with the experimental result. The
of MPEG and PEG is measured to be about 14 mmol L−1, determined by concentration corresponding to ηsp ≈ 1. Therefore, the normalized critical concentration cc/c* of PATP–MPC90% is predicted to be
, which is close to the experimental turning point at cc/c* = 20. For PATP–CPC45%, since the ratio of monomers of the polycation to CPC is 2
:
1,
, then
, which is three times the experimental result (cc/c* = 10). The difference between the theoretical calculation and the experimental result of cc for PATP–CPC45% may be attributed to more complex states of −CPC− which bears double carboxylate ions. At a low concentration, −CPC− will adopt a loop conformation on a single chain; as the concentration increases, −CPC− may also adopt a bridge conformation coupling different chains. Both loop and bridge conformations of −CPC− could influence the relaxation of the polycations. Research on PATP–CPC45% is under further investigation.
In comparison with η ∼ c0.5 for unentangled ordinary polyelectrolytes, η ∼ c1.5 for polycations with macrocounterions is mainly contributed by the long relaxation time τ while the terminal modulus G is similar to those of ordinary linear polyelectrolytes. This phenomenon is similar to the comb polyelectrolytes such as aggrecan, whose side combs do not contribute appreciably to the modulus, instead they contribute to energy dissipation in a range of naturally occurring shock absorbing tissues.29
In addition, it is worth mentioning that the viscosity of polycations with macrocounterions is significantly higher than that of solution mixtures of PATP–OH and MPEG (or PEG) at comparable concentrations (see ESI SI.3†). Therefore, it is believed that the high viscosity of polycations with macrocounterions is not merely attributed to the addition of a large amount of MPEG (or PEG), but more importantly, it is due to the characteristic relaxation of macrocounterions around the polycations.
3.3. Conductivity
Fig. 3 shows the dependences of (a) zero-shear viscosity (η0) and (b) conductivity (κ) on the molar fraction of MPC, CPC, MPEG and PEG for aqueous solutions of PATP–MPC, PATP–CPC, PATP–OH/MPEG and PATP–OH/PEG at 9 mmol L−1 at 20 °C. In particular, PATP–OH/MPEG (or PATP–OH/PEG) is the solution mixtures of PATP–OH with various molar fractions of MPEG (or PEG). For PATP–OH/MPEG and PATP–OH/PEG, their η0 increases while κ decreases as the molar fraction of MPEG and PEG increases. The conductivity of PATP–OH/MPEG (or PATP–OH/PEG) is contributed by both polycation (κ+) and OH− (κ−): | κ = κ+ + κ− = cp+λp+ + cOH−λOH− = (λp+ + λOH−)cp+ | (17) |
where λp+ and λOH− are the molar conductivity of the polycation and OH− respectively, cp+ and cOH− are the concentrations of dissociated monomers of polycations and dissociated OH− respectively and cp+ = cOH−.30 Moreover, the molar conductivity is related to ionic mobility (u):where up+ and uOH− are ionic mobilities of the polycation and OH− respectively, NA is Avogadro's number, e is the elementary charge, and N is the number of monomers per polycation. Furthermore, both up+ and uOH− are inversely proportional to solvent viscosity (ηs).30 As MPEG (or PEG) are added into PATP–OH solution, they contribute to solution viscosity, which can be considered as the increase of solvent viscosity (ηs). Therefore, up+ and uOH− decrease as ηs increases. Correspondingly, λp+ and λOH− decrease, and the solution conductivity κ falls. Concerning PATP–MPC and PATP–CPC solutions, as MPC or CPC are added to PATP–OH, the neutralization reaction takes place where certain amount of OH− is replaced by MPC− or −CPC−. It is observed that κ first decreases rapidly and then keeps constant, while η0 first decreases slightly and then increases significantly. The turning points of κ and η0 are almost the same, namely 40 and 20 mol% for PATP–MPC and PATP–CPC respectively. The conductivity of the PATP–MPC solution can be written as: | κ = κ+ + κ− = cp+λp+ + cOH−λOH− + cMPC−λMPC− | (20) |
where λp+, λOH− and λMPC− are the molar conductivity of the polycation, OH− and MPC− respectively, cp+, cOH−, and cMPC− are the concentrations of dissociated monomers of polycations, dissociated OH− and dissociated MPC− respectively. The concentration of cations equals that of anions due to electrostatic neutrality, and it is assumed that the effective charge density of polyelectrolytes keeps constant, so | cp+ = cOH− + cMPC− = constant | (21) |
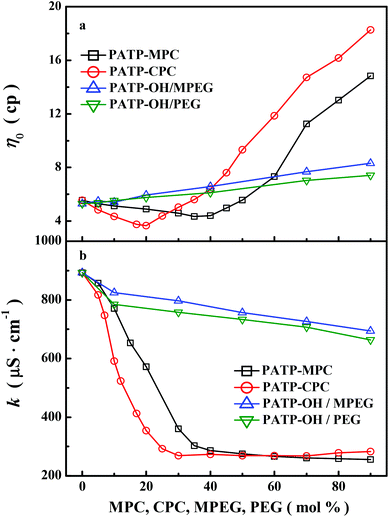 |
| Fig. 3 (a) Dependence of zero-shear viscosity (η0) and (b) conductivity (κ) on the molar fraction of MPC, CPC, MPEG and PEG for aqueous solutions of PATP–MPC, PATP–CPC, PATP–OH/MPEG and PATP–OH/PEG at 9 mmol L−1 at 20 °C. | |
Therefore, eqn (20) can be written as:
| κ = (λp+ + λOH−)cp+ + (λMPC− − λOH−)cMPC− | (22) |
where
λp+,
λOH− and
λMPC− are assumed to be constants since there is no change in solvent viscosity
ηs or temperature. Moreover,
λMPC− −
λOH− < 0 since OH
− exhibit higher ionic mobility due to the smaller hydrodynamic radius in comparison with MPC
−. Therefore,
κ changes with
cMPC− in
eqn (22). Comparing
eqn (17) and (22), it is understandable that
κ of PATP–MPC is lower than that of PATP–OH/MPEG or PATP–OH/PEG due to replacement of OH
− by MPC
−. This can also be verified by the result that the concentration of OH
− decreases in the same trend as
κ does when CPC are added to PATP–OH (see ESI SI.4
†). As
κ becomes constant when more MPC
− are added, it indicates that
cMPC− becomes constant. This is attributed to condensation of MPC
− which makes no contribution to conductivity. Therefore, the turning point of
κ indicates the effective charge density of polyelectrolytes, which is about 40 mol% for both PATP–MPC and PATP–CPC. This value is close to the prediction of Manning theory for polyelectrolytes in aqueous solution (35%). Concerning
η0, the increasing
η0 above 40 mol% (or 20 mol%) for PATP–MPC (or PATP–CPC) can be ascribed to the condensed macrocounterions which serve as dense brushes. However, it is unclear why
η0 decreases with MPC
− or
−CPC
− below the turning point? Considering the long polyethylene glycol (PEG) tails of macrocounterions (
Mn ≈ 5000), the local dielectric constant
ε is assumed to decrease due to much lower
ε of PEG in comparison with water. As the local dielectric constant decreases, the Coulomb's force between the macrocounterions and the polycations can be enhanced. The stronger electrostatic correlation between the free macrocounterions and the polycations will result in less electrostatic repulsion among the nearby monomers of polycations and thus a less stretched conformation. As the molar fraction of macrocounterions increases (but still below the turning point), the conformation of polycations is more collapsed and the viscosity decreases. When the molar fraction of macrocounterions is above the turning point, condensed macrocounterions can serve as brushes on the polycations, which may compensate the less stretched conformation and even stretch the polycations due to increased molecular rigidity.
Moreover, the condensed macrocounterions are removable when NaCl is added to the solution, where Cl− can replace macrocounterions. As a result, the viscosities of PATP–MPC90%, PATP–CPC45% and PATP–OH become similar to one another (see ESI SI.5†).
3.4. Dynamic light scattering
On the basis of the static parameters of electrostatic blobs calculated in Table 2 and described in Scheme 2(a) and (b), we investigated the diffusive relaxation on the length scales of electrostatic blobs by using polyelectrolytes synthesized by ATRP. The degree of polymerization of (PATP_ATRP)–OH is comparable to the number of monomers of an electrostatic blob shown in Table 2. Moreover, though the molecular weight of samples synthesized by ATRP is much lower than that of samples synthesized by free radical polymerization, (PATP_ATRP)–MPC90% exhibits higher viscosity compared with (PATP_ATRP)–OH (see ESI SI.6†). Fig. 4 shows the distribution of the hydrodynamics radius (Rh) of 2 mmol L−1 MPC, (PATP_ATRP)–OH, (PATP_ATRP)–OH in the presence of 10 mmol L−1 NaCl, and (PATP_ATRP)–MPC90% at an angle of 30° and at temperature of 20 °C. The concentrations are below the c* of (PATP_ATRP)–OH and (PATP_ATRP)–MPC90%. It is observed that Rh of MPC is 3.7 nm. As to (PATP_ATRP)–OH, two peaks of Rh are observed, which are centered at 1.1 and 21.5 nm respectively. In general, the smaller size indicates the coupled diffusive motion of polycations and OH−, while the large size indicates the inter-chain interaction at the long length scale. When 10 mmol L−1 NaCl is added to (PATP_ATRP)–OH, the long-range electrostatic interaction has been screened. As a result, a single peak at 13.4 nm is observed which resembles its neutral counterpart. Interestingly, Rh of (PATP_ATRP)–OH with added salt is about 4 times that of MPC, though their static size ξe is calculated to be smaller than that of MPC. This may be attributed to the fact that large amounts of charged groups of (PATP_ATRP)–OH are highly hydrated, which could contribute to a stronger hydrodynamic interaction and lead to larger Rh. For (PATP_ATRP)–MPC90%, it shows two peaks of Rh, which are centered at 8.6 and 113.4 nm respectively, where the slow and fast modes become 8 and 5 times larger than those of (PATP_ATRP)–OH respectively.
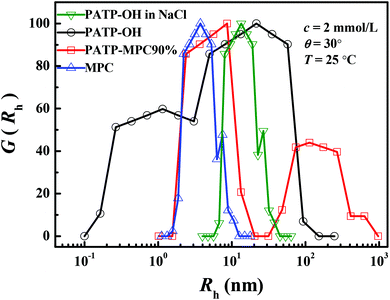 |
| Fig. 4 Distribution of the hydrodynamic radius (Rh) of 2 mmol L−1 MPC, (PATP_ATRP)–OH, (PATP_ATRP)–MPC90% and (PATP_ATRP)–OH in the presence of 10 mmol L−1 NaCl at an angle of 30° and at temperature of 20 °C. | |
In dynamic light scattering, the intensity autocorrelation function <I(t)I(t + τ)> can be related to the normalized electric-field autocorrelation function |g(1)(q,t)| = exp(−Γt), where Γ is the characteristic line width. For diffusive motion, Γ = Dq2, where D and q are the diffusion coefficient and the scattering vector respectively. When |g(1)(q,t)| contains two distinguishable relaxation modes, it can be analyzed using a combination of two exponential functions:
| 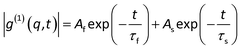 | (23) |
where
Af and
As are intensity contributions of the fast and slow modes respectively.
31 Note that
As +
Af = 1.
Fig. 5 shows the scattering vector (
q) dependence of (a) average characteristic line widths (
Γ) and (b) the intensity contribution of slow relaxation mode (
As/(
As +
Af)) for the sample PATP–MPC90% at 2 mmol L
−1 and at angles from 30° to 150°.
Fig. 5(a) shows that both slow and fast modes are diffusive motions because
Γ are linear functions of
q2 passing through the origin.
31 The slopes of
Γ vs. q2 lead to the fast and slow diffusion coefficient respectively, and
Df = 6.84 × 10
−7 cm
2 s
−1,
Ds = 4.37 × 10
−8 cm
2 s
−1. The diffusion coefficients of MPC, PATP–OH in NaCl, (PATP_ATRP)–OH and (PATP_ATRP)–MPC90% are summarized in
Table 3. Since the relaxation of neutral MPC can be detected by dynamic light scattering, it is possible that the independent diffusion of MPC
− (
DMPC−) can be observed. However, only two relaxation modes are observed. Therefore, it is supposed that the motion of MPC
− is no longer independent but coupled with the polycation, which is reflected by
Df. Moreover, both
Df and
Ds slowed down in comparison with (PATP_ATRP)–OH, which may be attributed to the topological constrains of multiple macrocounterions on the diffusion of polycations.
Fig. 5(b) shows that the intensity contribution of the slow mode
As keeps constant at about 30%, which is half of the contribution of the fast mode
Af dominated by macrocounterions. Therefore, the fast mode dominates over the slow mode for (PATP_ATRP)–MPC90%. However, for ordinary polyelectrolytes without added salt, the slow mode dominates over the fast mode with
As ≈ 60%.
4,31 Therefore, the existence of macrocounterions results in more significant fast mode relaxation of polyelectrolytes. Further studies on dynamic light scattering of polycations with macrocounterions on length scales of the contour length of PATP–MPC90% and PATP–CPC45% are still under investigation.
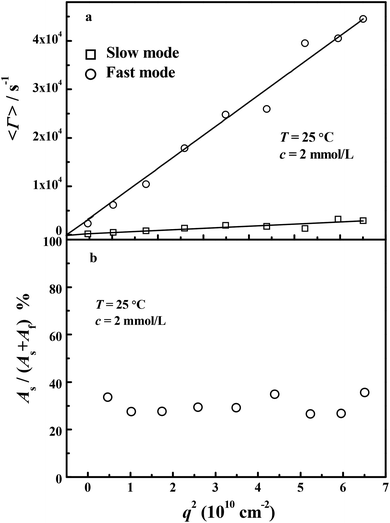 |
| Fig. 5 Scattering vector (q) dependence of (a) average characteristic line widths (Γ) and (b) intensity contribution of slow relaxation mode (As/(As + Af)) for the sample (PATP_ATRP)–MPC90% at 2 mmol L−1 and 20 °C. Note that As + Af = 1. The angular range is 30°–150°. | |
Table 3 Diffusion coefficients of 2 mmol L−1 MPC, (PATP_ATRP)–OH in 10 mmol L−1 NaCl, (PATP_ATRP)–OH and (PATP_ATRP)–MPC90%
Sample |
D/10−7 cm2 s−1 |
MPC |
11.5 |
(PATP_ATRP)–OH in NaCl |
3.19 |
|
D
f/10−7 cm2 s−1 |
D
s/10−7 cm2 s−1 |
(PATP_ATRP)–OH |
37.0 |
2.58 |
(PATP_ATRP)–MPC90% |
6.84 |
0.437 |
4. Conclusion
The present work is analyzed on the basis of the scaling theory, concerning the effective charge density, the static parameters and the dynamics of polycations with macrocounterions on various length scales. It is found that on the length scale of the contour length of polycations, a critical concentration (
) exists. When the concentration is below
, the role of macrocounterions can be ignored; while above
, macrocounterions dominate the relaxation of polycations and prevent entanglement of polycations. On the length scale of electrostatic blobs, macrocounterions lead to a significantly delayed bimodal diffusive relaxation and the fast mode relaxation becomes dominant. Since no independent relaxation of macrocounterions has been observed, it is supposed that the fast mode relaxation is the coupled relaxation of polycations and macrocounterions. Moreover, macrocounterions can't serve as brushes condensed on the polycations until their molar fraction is above the effective charge density of polyelectrolytes. Polycations with macrocounterions may provide a great chance to further explore the characteristics of polyelectrolyte relaxation, whereas many issues still require further investigation.
Acknowledgements
This work was supported by the National Nature Science Foundation of China (no. 51473145) and the Fundamental Research Funds for the Central Universities (no. 2013QNA4048). The authors are grateful for the discussion with Professor Ralph Colby and Doctor Quan Chen of The Pennsylvania State University.
References
- A. Deshkovski, S. Obukhov and M. Rubinstein, Phys. Rev. Lett., 2001, 86, 2341–2344 CrossRef CAS PubMed.
- M. Muthukumar, J. Chem. Phys., 2004, 120, 9343–9350 CrossRef CAS PubMed.
- H. Schiessel and P. Pincus, Macromolecules, 1998, 31, 7953–7959 CrossRef CAS.
- H. Matsuoka, Y. Ogura and H. Yamaoka, J. Chem. Phys., 1998, 109, 6125–6132 CrossRef CAS.
- R. W. Chang and A. Yethiraj, J. Chem. Phys., 2002, 116, 5284–5298 CrossRef CAS.
- M. Rubinstein and G. A. Papoian, Soft Matter, 2012, 8, 9265–9267 RSC.
- T. E. Angelini, L. K. Sanders, H. J. Liang, W. Wriggers, J. X. Tang and G. C. L. Wong, J. Phys.: Condens. Matter, 2005, 17, S1123–S1135 CrossRef CAS.
- M. Kanduc, A. Naji and R. Podgornik, J. Chem. Phys., 2010, 132 Search PubMed.
- G. Decher, J. D. Hong and J. Schmitt, Thin Solid Films, 1992, 210, 831–835 CrossRef.
- Q. Zhao, Q. F. F. An, Y. L. Ji, J. W. Qian and C. J. Gao, J. Membr. Sci., 2011, 379, 19–45 CrossRef CAS.
- J. P. Gong, Y. Katsuyama, T. Kurokawa and Y. Osada, Adv. Mater., 2003, 15, 1155–1158 CrossRef CAS.
- A. V. Dobrynin, R. H. Colby and M. Rubinstein, J. Polym. Sci., Part B: Polym. Phys., 2004, 42, 3513–3538 CrossRef CAS.
- G. S. Georgiev, E. B. Karnenska, E. D. Vassileva, I. P. Kamenova, V. T. Georgieva, S. B. Iliev and I. A. Ivanov, Biomacromolecules, 2006, 7, 1329–1334 CrossRef CAS PubMed.
- Y. B. Zhang, J. F. Douglas, B. D. Ermi and E. J. Amis, J. Chem. Phys., 2001, 114, 3299–3313 CrossRef CAS.
- S. K. Filippov, T. A. P. Seery, J. Kriz, M. Hruby, P. Cernoch, O. Sedlacek, P. Kadlec, J. Panek and P. Stepanek, Polym. Int., 2013, 62, 1271–1276 CrossRef CAS.
- A. Mehrdad, M. T. Taghizadeh and R. Moladoust, J. Solution Chem., 2011, 40, 832–842 CrossRef CAS.
- M. Spitzer, E. Sabadini and W. Loh, J. Phys. Chem. B, 2002, 106, 12448–12452 CrossRef CAS.
- J.-H. H. Wang and R. H. Colby, Soft Matter, 2013, 9, 10275–10286 RSC.
- W. Wang, W. Liu, G. J. Tudryn, R. H. Colby and K. I. Winey, Macromolecules, 2010, 43, 4223–4229 CrossRef CAS.
- J. Bodycomb and M. Hara, Macromolecules, 1995, 28, 8190–8197 CrossRef CAS.
- A. V. Dobrynin, R. H. Colby and M. Rubinstein, Macromolecules, 1995, 28, 1859–1871 CrossRef CAS.
- R. H. Colby, Rheol. Acta, 2010, 49, 425–442 CrossRef CAS.
- S. Dou and R. H. Colby, J. Polym. Sci., Part B: Polym. Phys., 2006, 44, 2001–2013 CrossRef CAS.
-
M. Rubinstein and R. H. Colby, Polymer Physics, Oxford University Press, USA, 2003 Search PubMed.
- S. Namba, Y. Tsukahara, K. Kaeriyama, K. Okamoto and M. Takahashi, Polymer, 2000, 41, 5165–5171 CrossRef CAS.
- S. Desvergne, V. Heroguez, Y. Gnanou and R. Borsali, Macromolecules, 2005, 38, 2400–2409 CrossRef CAS.
- A. Papagiannopoulos, C. M. Fernyhough and T. A. Waigh, J. Chem. Phys., 2005, 123 Search PubMed.
- A. Papagiannopoulos, C. M. Fernyhough, T. A. Waigh and A. Radulescu, Macromol. Chem. Phys., 2008, 209, 2475–2486 CrossRef CAS.
- A. Papagiannopoulos, T. A. Waigh, T. Hardingham and M. Heinrich, Biomacromolecules, 2006, 7, 2162–2172 CrossRef CAS PubMed.
- F. Bordi, C. Cametti and R. H. Colby, J. Phys.: Condens. Matter, 2004, 16, 1423–1463 CrossRef.
- K. J. Zhou, J. F. Li, Y. J. Lu, G. Z. Zhang, Z. W. Xie and C. Wu, Macromolecules, 2009, 42, 7146–7154 CrossRef CAS.
Footnote |
† Electronic supplementary information (ESI) available. See DOI: 10.1039/c5py01022e |
|
This journal is © The Royal Society of Chemistry 2016 |
Click here to see how this site uses Cookies. View our privacy policy here.