Dewar valence isomers, the third type of environmentally relevant DNA photoproducts induced by solar radiation
Received
13th October 2015
, Accepted 12th December 2015
First published on 14th December 2015
Abstract
UV-induced DNA damage is the main initiating event in solar carcinogenesis. UV radiation is known to induce pyrimidine dimers in DNA, including cyclobutane dimers and (6-4) photoproducts which have been extensively studied. In contrast, much less attention has been paid to Dewar valence isomers, the photoisomerisation product of (6-4) photoproducts. Yet, the available data show that Dewar isomers can be produced by exposure to sunlight and may lead to mutations. Dewars are thus environmentally and biologically relevant. The present review summarizes currently available information on the formation, mutagenic properties and repair of this class of UV-induced DNA damage.
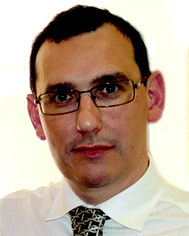 T. Douki | Thierry Douki graduated in organic synthesis and became interested in DNA reactivity. His scientific activity is devoted to the study of DNA damage induced by a series of genotoxic physical and chemical agents. Among them, solar UV radiation represents a major part of his research interest, in terms of basic photochemistry as well as damage and repair in cellular DNA. His work is thus at the interface between chemistry and biology. His main areas of competence are photochemistry, mechanism of formation of DNA damage, analytical chemistry and DNA repair. |
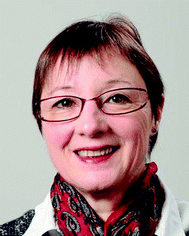 E. Sage | Evelyne Sage graduated in Biophysics from the University of Orléans (France), led the research team entitled “Biology of radiations” at Institut Curie in Orsay. Currently she is a CNRS Research Director Emeritus at Institut Curie. She has been active in the field of DNA damage, repair and mutagenesis induced by solar UV radiation, exploring in particular the contribution of UVA in sunlight-induced mutagenesis and carcinogenesis. |
Introduction
Induction of DNA damage by solar UV radiation is a major issue for living organisms, from bacteria to plants and mammals. Presence of photoproducts in the genome results in several deleterious processes, such as growth reduction in plants, lethality in microorganisms, and skin ageing and cancer and possibly ocular diseases in humans. Because of its major impact on the environment and on human health, UV-induced DNA damage has been the topic of numerous photochemical, biochemical and cellular investigations. It is now well demonstrated that the most highly DNA damaging portion of the terrestrial solar spectrum is UVB which induces dimerization reactions between adjacent pyrimidine bases, thymine (T), cytosine (C) or 5-methylcytosine (mC). Two main types of photoproducts are produced: the cyclobutane pyrimidine dimers, specifically their cis,syn diastereoisomer (c,s CPDs), and the pyrimidine (6-4) pyrimidone photoproducts (64PPs) (Fig. 1). Both have been the subject of extensive research in recent decades. In addition, photochemists have shown that 64PPs can give rise, by absorption of a second photon, to a third type of photoproduct known as the Dewar valence isomers (Dewars).1 Although well characterized from a chemical point of view, Dewars have been much less studied for their biological properties. However, in 2000, one publication investigating the formation of DNA photoproducts in cultured cells was subtitled “Possible role of Dewar photoproducts in solar mutagenesis”.2 Later, the same group proposed the involvement of Dewars in mutations induced by simulated sunlight.3 More recently, a large scale field study has demonstrated that roughly 20% of the 64PPs were converted into Dewars in the DNA of microorganisms exposed to natural sunlight.4 These observations, combined with the data accumulated by different groups using simulated solar radiation now strongly support the relevance of Dewar isomers to solar genotoxicity. The present review provides information on the formation and biological properties of Dewars in DNA and summarizes data showing the relevance of this class of photoproducts upon exposure to sunlight and not only to laboratory settings.
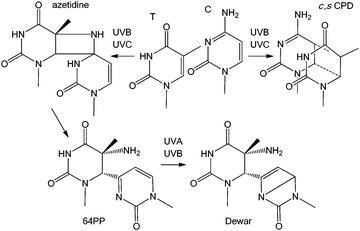 |
| Fig. 1 The three main types of pyrimidine dimers. The figure represents photoproducts produced at a TC sequence. The azetidine is the intermediate in the formation of the 64PP. The same reactions take place at TT, CT and CC sequences. | |
Photochemistry and properties of Dewar isomers
c,s CPDs were first isolated and identified in 1958 and 64PPs a few years later. Numerous works on monomers and isolated DNA have provided extensive information on the basic photochemical and chemical properties of these two classes of photoproducts. In particular a strong absorption around 320 nm associated with fluorescence emission was established for 64PPs, both resulting from the presence of the pyrimidone moiety. Interestingly, it was also observed, as early as 1964, that UVB irradiation of the 64PP of the dinucleoside monophosphate TpT led to a photoproduct, for a long time known as TpT3, which had lost both its 325 nm absorption and its fluorescence.5 It was only more than 20 years later that Taylor and Cohrs reported in a key paper1 that TpT3 was in fact the Dewar valence isomer of TT 64PP.6,7
The Dewar valence isomer, which owes its name to a British scientist of the XIXth century working on the structure of benzene, results from a photoinduced intramolecular 4π electrocyclisation of the pyrimidone ring. The photoisomerisation into Dewar valence isomers was subsequently reported for the 64PPs of TpdC,8 dCpT,9 dCpdC10 and Tp5mdC.11 In addition to UVB, UVC was also reported to induce 64PP isomerisation5,7 although with a lesser efficiency. Recent time-resolved spectroscopic studies have shown that, in a model system where the thymines of a 64PP are linked by a silyl spacer, this photoisomerisation takes place within 130 ps, namely much more slowly than formation of c,s CPDs.12 Surprisingly, these groups also reported and provided theoretical explanations for the absence of photoisomerisation of structurally unconstrained 64PP lacking the bridge between the deoxyribose moieties of the two nucleotides.12–14 This observation contrasts with previous reports of the UVB-induced isomerisation of 64PPs of thymine and thymidine.15,16 As shown in Fig. 2, the diastereoisomers of thymidine (Thd) 64PP are readily converted into two dimeric photoisomers lacking UVB absorption unambiguously characterized as Dewar isomers.17
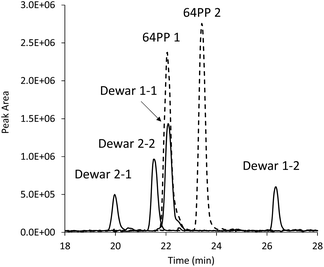 |
| Fig. 2 Evidence for the photoisomerisation of thymidine 64PP. The chromatogram corresponds to an HPLC-MS/MS analysis monitoring dimeric thymidine photoproducts. The two purified diastereoisomers of 64PPs (64PP1 and 64PP2, dotted lines) of Thd were exposed to UVB and each yielded two diastereoisomeric Dewars (Dewar 1-1, 1-2, 2-1 and 2-2, solid lines). Absorption at 325 nm at the retention time of the two 64PPs was lost upon UVB irradiation. Data were taken from ref. 17. | |
Several works have provided basic information on the photochemical process leading to the formation of Dewars. Recent theoretical studies suggest that the excited states involved in the formation of Dewars are singlet ππ* states. Excitation of 64PPs also produces triplet states that do not seem to be involved in the photoconversion into Dewars.13,18 A quantum yield of 2% was determined for the isomerisation of TpT and TpdC 64PPs.19 A higher efficiency of 8% was recently reported for the photoisomerisation using time-resolved spectroscopy.12 However, the quantum yield of conversion of 64PP into Dewar is reduced by a factor of 4 in double-stranded DNA due to the presence of the adjacent bases.20 These values are slightly larger than for the formation of c,s CPDs and more than one order of magnitude larger than for induction of 64PPs in DNA. The photoisomerisation of 64PPs can thus be considered as an efficient photochemical process. In that respect, it would be interesting to know how the proposed triplet–triplet energy transfer from excited pyrimidone in 64PPs21 can compete with the conversion of the latter photoproducts into Dewars.
Once formed, some of the Dewars may undergo a secondary hydrolytic reaction, the well-known deamination that converts cytosine, and even more efficiently its 5,6-saturated derivatives, into the related uracil compounds. Importantly, because the 3′-end base of Dewars and 64PPs is a pyrimidone and no longer a pyrimidine, only Dewars and 64PPs with a 5′-end cytosine, namely the CT and CC derivatives in DNA, are targets for deamination. The half-life times for deamination of the dCpT Dewar and 64PP were found to be more than 400 h, namely two orders of magnitude longer than that of the c,s CPD.22
Structural and mutational properties of Dewar isomers
Determination of the structural and mutational properties of Dewars has been made possible by the synthesis of oligonucleotides bearing specific photoproducts.23,24 Extensive NMR characterization and thermodynamic analyses have provided information on the structural impact of the presence of Dewars in DNA. Like their 64PP precursors, Dewars induce a much more severe bend into DNA than c,s CPDs. The bending angles are 44°, 21° and less than 10° for TT 64PP, Dewar and CPD respectively.25 The slightly lower structural impact of Dewars with respect to 64PPs is also evident in the effects of the photoproducts on duplex stability. A 2 °C higher melting temperature was reported for a 12-mer containing a Dewar compared to a 64PP.26 Interestingly, the hydrogen bonding of the two TT photoproducts with complementary strand bases shows opposite trends. In the 64PP, the 5′-end pyrimidine base retains one hydrogen bond with the opposite adenine while none remains on the 3′-end pyrimidone side. After photoisomerisation, no hydrogen bond is observed on the 5′-end thymine of the Dewar while bonding with the NH2 at position 6 of A or at position 2 of G becomes possible for the Dewar pyrimidone ring.25,27 Another interesting observation, based on thermodynamic considerations, is that the presence of G as compared to A opposite the 3′-end of a TT 64PP strongly stabilizes the duplex. This trend is much less pronounced for Dewars.26,28 Further work has shown that pairing of the Dewar pyrimidone with G is stronger than with A but the resulting duplex is more constrained.29 As a result, no preferential binding of either G or A takes place and Dewars may be considered as non-coding lesions.
These observations have helped to rationalize the mutagenic properties of the TT and TC derivatives of 64PPs and Dewars. Mutation spectra experiments have mostly been conducted using the phage vector approach in SOS-induced bacteria. Dewars are less mutagenic than their 64PP precursors but exhibit a reduced specificity, producing a broader range of mutations.29–31 Both are 4 to 8 times more mutagenic than c,s CPD. 64PPs and Dewars do not exhibit the same mutational specificity.29–32 Either as the result of hydrogen bonding properties of the pyrimidone ring or in agreement with the “A rule” for noncoding lesions, A is incorporated much more frequently on the 3′-end side of Dewars than opposite the 64PPs where the 3′-end pyrimidone codes mostly for G. Incorporation of G opposite the 3′-end base is also observed for Dewars but 4 to 5-times less frequently than incorporation of A.32 For both photoproducts, incorporation of A opposite the 5′-end pyrimidine is the most frequent event. Altogether, after two replication cycles, 64PPs give rise mostly to TC and Dewars to TT sequences, respectively. Such replication events represent transition mutations at the 3′-end in the case of TT 64PP30,32 and TC Dewar,31 respectively (Fig. 3). Photoisomerisation exhibits thus a drastic effect on the mutational properties of 64PPs. For TT Dewars, the less frequent incorporation of G opposite the 3′-end base is also the cause of some TT to TC transitions.30,32 Very little is known about the mutational properties of Dewars in vivo. Experiments carried out in Saccharomyces cerevisiae suggested that the difference in mutation spectra determined in cells exposed to either UVC or SSL could be explained by the formation of Dewars by the latter radiation.3 Moreover, the same type of study in yeast lacking specific DNA polymerases suggested a major role for polymerase ζ in the error-prone trans-lesion synthesis of Dewars.
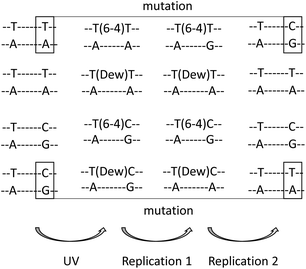 |
| Fig. 3 Most frequent mutational events at TT and TC 64PPs and Dewars. During the first replication, A is inserted opposite the 5′-end T of both photoproducts. At the 3′-end base, a 64PP most frequently pairs with G and a Dewar with A. As a consequence, a second replication induces mutation for the TT 64PP and the TC Dewar. As a general trend, a mutational event is infrequent for TC 64PP and TT Dewar, although TT to TC mutation may occur for the latter photoproduct as a minor pathway. | |
Repair of Dewar isomers
Because of their mutagenic and cytotoxic properties, UV-induced photoproducts ought to be actively removed from DNA by repair enzymes in all cell types. A major pathway is nucleotide excision repair (NER), a DNA repair system that recognizes a wide range of substrates, and in particular 64PPs and CPDs. Information on the efficacy of repair of Dewars in human cells is limited. Some works carried out in CHO cells2 and human fibroblasts33 led to the conclusion that the Dewars were repaired with a rate similar to that of CPDs. However, in both studies, UV doses applied to cells for the quantification of Dewars were much larger than for CPDs. In contrast, works performed in mammalian cells exposed to the same UV dose for all photoproducts measurements led to the conclusion that the efficiency of the repair of Dewars is similar to that of 64PPs.34,35 The observation that the rate of repair depends on the applied dose and the initial level of photoproducts36,37 could explain the differences between these two sets of data. Altogether, it seems thus likely that Dewars are repaired with an efficiency similar that of 64PPs.
These observations can easily be explained from a molecular point of view. A key step in NER is the recognition of the damage by proteins sensitive to large structural modifications of DNA. Consequently, DNA lesions that induce large structural distortion are more easily recognized than less helix-distorting damage. These considerations explain why 64PPs and Dewars are repaired more efficiently by global genome-NER than c,s CPDs which induce a lesser bending of DNA. Accordingly, damage-sensing proteins like DDB2 (XPE) bind to photoproduct-containing duplexes in the following order: 64PP > Dewar ≫ c,s CPD.38 No data are available for the repair of Dewars in prokaryotic cells but UvrA protein, which recognizes DNA damage in the UvrABC NER system in bacteria, was found to bind to thymine dimeric photoproducts with a selectivity equivalent to that of DDB2.38 Moreover, an in vitro assay with reconstituted bacterial UvrABC excinuclease showed that the repair rates for 64PP, Dewar and c,s CPD were in a 9
:
9
:
1 ratio.39
In some organisms, NER is not the only repair pathway that removes UV-induced dimeric photoproducts. For instance, some yeasts and bacteria possess a protein known as ultraviolet DNA endonuclease (UVDE) that cleaves DNA at the 5′-end of dimeric pyrimidine photoproducts. TT Dewar was found to be a substrate for UVDE from Schizosaccharomyces pombe.40 A more common alternative DNA repair mechanism for photoproducts, found in bacteria, yeasts, plants, fishes and some mammals involves photo-activated enzymes, the photolyases. These proteins, which contain a light harvesting chromophore and a flavin cofactor, revert the dimeric photoproducts into the original bases upon absorption of blue light. Photolyases are substrate-specific, those reverting CPDs being the most common enzymes. Yet certain organisms such as some yeasts, fishes and plants, but not bacteria, also express a (6-4) photoproduct photolyase (64PP-PL). No information is available yet on the existence of specific Dewar-photolyase. However, TT Dewar was found to bind efficiently to 64PP-PL but was very poorly photoreverted if at all.41–44 In contrast, TC Dewar appeared to be a better substrate for 64PP-PL.45 The repair mechanism of 64PP-PL is still under debate but in the case of Dewars, it seems accepted that the initial step is reversion into the 64PP precursor by electron injection. This was proposed on the basis of biochemical studies using mutant enzymes46 and validated by theoretical considerations.18,47,48 This mechanism provides an explanation for the poor repair of TT Dewar compared to the TC derivative. Indeed, methylation at C5 was found to destabilize the radical anion transition state involved in the reversion of the Dewar into 64PP within the protein.49 It is worth mentioning that 64PP-PL exhibits a low quantum yield even with its favoured 64PP substrate and that Dewars are not as efficiently reverted as 64PPs. It is thus probable that NER remains the most important repair mechanism for Dewar photoproducts even in cells expressing 64PP-PL.
Formation of Dewar isomers in isolated and cellular DNA
Gathering information on DNA damage is a challenge because it requires the design of appropriate analytical tools. Dewars could not be detected using acidic hydrolysis of radiolabeled DNA, which was widely used in early studies, because the lesion is unstable under acidic and alkaline conditions.7,23 Noticeably, the alkaline cleavage of UV-irradiated DNA, once believed to be associated with the presence of 64PPs, reveals Dewars.34 Production of specific antibodies50–52 made it possible to extend to the detection of Dewars the immunological approaches, which had been successful for CPDs and 64PPs. More recently, mild enzymatic hydrolysis of DNA has enabled liquid chromatography coupled with mass spectrometry (HPLC-MS/MS) to be used for the sensitive quantification of dimeric photoproducts including Dewar isomers.53
Using either method, the formation of Dewars has been established in a variety of biological systems. Upon exposure to simulated sunlight, Dewars were detected in the DNA of cultured human fibroblasts,33 human mononuclear cells54 and Chinese Hamster Ovary cells.2 In the latter work, optimizing the immunological detection allowed the authors to show that the distribution of the 3 dimeric photoproducts greatly varies with UV wavelengths. In particular, the Dewars to CPDs ratios were 1
:
70 and 1
:
16, and the Dewars to 64PP ratios were 1
:
8 and 1
:
3, upon UVB and simulated sunlight irradiation, respectively. In addition, simulated sunlight induced about 5 times more Dewars than 8-oxoguanine, emphasizing that Dewars are quantitatively the third photolesion induced by sunlight.2,56 More specific HPLC-MS/MS measurements on the DNA of the same cells confirmed the presence of large amounts of both TT and TC Dewars in samples exposed to simulated sunlight while they were not detectable for biologically relevant UVB doses.55 In SSL-exposed cells, the overall ratio between CPDs and Dewars was 6.1. Eighty six and 66% of the 64PP were converted into Dewars at TT and TC sites, respectively. Dewars were also detected in human skin explants exposed to doses as low as 1 minimal erythemal dose (MED) of simulated sunlight (Fig. 4), and even 0.5 MED for the most sensitive donors.56 Interestingly, the ratio between the amount of 64PPs and Dewars decreases with increasing SSL dose, from 4.9 at 1 MED to 1.7 at 3 MED. This observation is explained by the quadratic-shape of the dose-course formation of Dewars. In addition to mammalian systems, the formation of Dewars has also been reported in plants57 and bacteria58–60 exposed to various combinations of UVB and UVA.
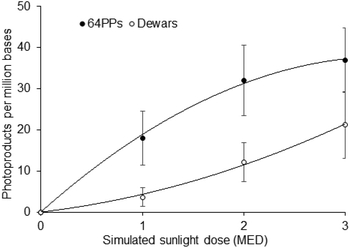 |
| Fig. 4 Formation of 64PPs and Dewars in skin explants exposed to simulated sunlight. The reported values correspond to the sum of the level of the TT and TC photoproducts. Data are the average ± standard error for three donors. Under the irradiation conditions used, 1 MED corresponded to 5.5 J cm−2. Data were taken from ref. 55. | |
Dewars also constitute relevant lesions upon exposure to sunlight. They have been first detected following exposure of cultured human mononuclear cells to actual solar radiation.54 While the level of 64PPs rapidly reached a plateau, that of Dewars kept on increasing and became the second most frequent type of photoproduct after 2 h of exposure. Dewars were also detected, although in lower proportion compared to 64PPs, in mouse skin,61 and, more recently, in marine microorganisms during a large field experiment across the eastern Pacific Ocean.4 In the latter works, the level of Dewars represented approximately 10% of that of CPDs and 20 to 30% of that of 64PPs, respectively. One can thus now state unambiguously that Dewar valence isomers are environmentally relevant DNA photoproducts.
These reports of the formation of Dewars were obtained with natural or simulated sunlight, namely with UV sources containing a few percent of UVB in UVA. In addition, several studies have reported the formation of Dewars by sequential irradiation with either UVC or UVB and UVA, namely in mammalian cells34,35 and in human skin.62 Similarly, a large proportion of Dewars, detected by an immunological assay, was determined in irradiated human cells when the UVB source contained a significant amount of UVA radiation.63 On the opposite, the level of Dewars remained below the detection limit of the HPLC-MS/MS in cultured human cells and human skin when only UVB was applied.35,64 The major role of UVA in the formation of Dewars, already pointed out at the time of their identification,1 may seem unexpected since the maximum absorption of 64PPs is around 320 nm. However, the formation of a Dewar requires two photons, one for the formation of a 64PP, the other for its isomerisation. In the first step the chromophore is a normal DNA pyrimidine base that exhibits absorption in the UVC and UVB ranges. In contrast, in the second step, the photon is absorbed by the 64PP that absorbs both UVB and UVA. At low levels of DNA modification, as in the case of biologically relevant radiation doses, normal bases are present in amounts that are orders of magnitude larger than 64PPs. Although they do not very efficiently absorb UVB, those normal bases shield a significant proportion of the 64PPs from the incident photons. As a consequence, the efficiency of the photoisomerisation depends on the frequency of 64PPs and increases with the UV dose. This is clearly shown by the quadratic shape of the formation of 64PPs in isolated DNA exposed to pure UVB.10 It is also in agreement with the fact that the maximum of the action spectra for the photolysis of 64PPs in heavily damaged DNA coincides with the absorption spectrum of the 64PP.50 The situation differs when DNA or cells are exposed to a mixture of UVB and UVA. 64PPs have an absorption which is maximal around 320 nm and extends into the UVA range. In this wavelengths range, absorption by normal bases is much lower than in the UVB region. As a consequence, photolysis of the 64PPs by UVA photons is much less hampered by shielding from normal DNA bases and takes place at a lower dose.
Conclusion
It is clear in light of current evidence that Dewar valence isomers are relevant DNA lesions induced by exposure to sunlight in all types of cells and organisms. Because a substantial fraction of 64PPs is converted into this isomer, experiments aiming at an accurate determination of the load of bipyrimidine photoproducts in exposed cells have to take Dewars into account. Indeed, after hours of exposure to natural solar radiation, Dewars are quantitatively the second most frequent photoproducts after CPDs as shown in cultured human cells.54
Significant amounts of information are available on the chemical and biological properties of Dewars. In particular, they have been shown to be mutagenic, especially at TC dinucleotides which are mutational hotspots in UV-exposed cells. In mammalian cells, and specifically in human skin, Dewars are likely removed from DNA almost as efficiently as 64PPs. This may limit the contribution of Dewars to solar mutagenesis as has been shown for 64PPs.65 In contrast to NER, Dewars are poorly handled by 64PP photolyases in organisms expressing this class of proteins and they are not substrates for bacterial photolyases that are specific for CPDs. Consequently, it may be anticipated that Dewars, like 64PPs, play a major role in the genotoxic stress induced by solar exposure in bacteria.
The data reported in the present review show that Dewars are mutagenic lesions and can be produced in significant yield. Dewars thus need to be considered when estimating possible outcomes of exposure to UV. The fact that formation of Dewars is most efficient in the case of combined exposure to UVB and UVA makes this class of photoproducts environmentally relevant to the deleterious effects of sunlight.
Acknowledgements
TD wishes to thank the French “Agence National pour la Recherche” for financial support through the OPHID (ANR-12-BS08-0001-02) project.
References
- J.-S. Taylor and M. P. Cohrs, J. Am. Chem. Soc., 1987, 109, 2834–2835 CrossRef CAS.
- D. Perdiz, P. Grof, M. Mezzina, O. Nikaido, E. Moustacchi and E. Sage, J. Biol. Chem., 2000, 275, 26732–26742 CAS.
- S. G. Kozmin, Y. I. Pavlov, T. A. Kunkel and E. Sage, Nucleic Acids Res., 2003, 31, 4541–4552 CrossRef CAS PubMed.
- J. A. Meador, A. J. Baldwin, J. D. Pakulski, W. H. Jeffrey, D. L. Mitchell and T. Douki, Environ. Microbiol., 2014, 16, 1808–1820 CrossRef CAS PubMed.
- H. E. Johns, M. L. Pearson, J. C. LeBlanc and C. W. Helleiner, J. Mol. Biol., 1964, 9, 503–524 CrossRef CAS PubMed.
- J.-S. Taylor, D. S. Garrett and M. P. Cohrs, Biochemistry, 1988, 27, 7206–7215 CrossRef CAS PubMed.
- L.-S. Kan, L. Voituriez and J. Cadet, J. Photochem. Photobiol., B, 1992, 12, 339–357 CrossRef CAS.
- J.-S. Taylor, H.-L. Lu and J. J. Kotyk, Photochem. Photobiol., 1990, 51, 161–167 CrossRef CAS PubMed.
- T. Douki, L. Voituriez and J. Cadet, Photochem. Photobiol., 1991, 27, 293–297 CrossRef.
- T. Douki and J. Cadet, Biochemistry, 2001, 40, 2495–2501 CrossRef CAS PubMed.
- L. Celewicz, M. Mayer and M. D. Shetlar, Photochem. Photobiol., 2005, 81, 404–418 CrossRef CAS PubMed.
- K. Haiser, B. P. Fingerhut, K. Heil, A. Glas, T. T. Herzog, B. M. Pilles, W. J. Schreier, W. Zinth, R. de Vivie-Riedle and T. Carell, Angew. Chem., Int. Ed., 2012, 51, 408–411 CrossRef CAS PubMed.
- B. P. Fingerhut, T. T. Herzog, G. Ryseck, K. Haiser, F. F. Graupner, K. Heil, P. Gilch, W. J. Schreier, T. Carell, R. de Vivie-Riedle and W. Zinth, New J. Phys., 2012, 14 CrossRef CAS , 065006.
- B. P. Fingerhut, S. Oesterling, K. Haiser, K. Heil, A. Glas, W. J. Schreier, W. Zinth, T. Carell and R. de Vivie-Riedle, J. Chem. Phys., 2012, 136, 204307 CrossRef PubMed.
- A. J. Varghese, Photochem. Photobiol., 1971, 13, 357–364 CrossRef CAS.
- T. Douki, M. Court and J. Cadet, J. Photochem. Photobiol., B, 2000, 54, 145–154 CrossRef CAS.
- T. Douki, S. Rebelo-Moreira, N. Hamon and P. A. Bayle, Org. Lett., 2015, 17, 246–249 CrossRef CAS PubMed.
- Y. J. Ai, R. Z. Liao, S. F. Chen, Y. Luo and W. H. Fang, J. Phys. Chem. B, 2010, 114, 14096–14102 CrossRef CAS PubMed.
- D. G. E. Lemaire and B. P. Ruzsicska, Photochem. Photobiol., 1993, 57, 755–769 CrossRef CAS PubMed.
- D. B. Bucher, B. M. Pilles, T. Carell and W. Zinth, J. Phys. Chem. B, 2015, 119, 8685–8692 CrossRef CAS PubMed.
- V. Vendrell-Criado, G. M. Rodriguez-Muniz, M. C. Cuquerella, V. Lhiaubet-Vallet and M. A. Miranda, Angew. Chem., Int. Ed., 2013, 52, 6476–6479 CrossRef CAS PubMed.
- T. Douki and J. Cadet, J. Photochem. Photobiol., B, 1992, 15, 199–213 CrossRef CAS.
- C. A. Smith and J.-S. Taylor, J. Biol. Chem., 1993, 268, 11143–11151 CAS.
- S. Iwai, M. Shimizu, H. Kamiya and E. Ohtsuka, J. Am. Chem. Soc., 1996, 118, 7642–7643 CrossRef CAS.
- J. H. Lee, G. S. Hwang, J. K. Kim and B. S. Choi, FEBS Lett., 1998, 428, 269–274 CrossRef CAS PubMed.
- Y. Q. Jing, J. F. L. Kao and J. S. Taylor, Nucleic Acids Res., 1998, 26, 3845–3853 CrossRef CAS PubMed.
- G. S. Hwang, J. K. Kim and B. S. Choi, Eur. J. Biochem., 1996, 235, 359–365 CAS.
- Y. Fujiwara and S. Iwai, Biochemistry, 1997, 36, 1544–1550 CrossRef CAS PubMed.
- J.-H. Lee, S.-H. Bae and B.-S. Choi, Proc. Natl. Acad. Sci. U. S. A., 2000, 97, 4591–4596 CrossRef CAS PubMed.
- J. E. Leclerc, A. Borden and C. W. Lawrence, Proc. Natl. Acad. Sci. U. S. A., 1991, 88, 9685–9689 CrossRef CAS.
- M. J. Horsfall and C. W. Lawrence, J. Mol. Biol., 1994, 235, 465–471 CrossRef CAS PubMed.
- C. A. Smith, M. Wang, N. Jiang, L. Che, X. D. Zhao and J. S. Taylor, Biochemistry, 1996, 35, 4146–4154 CrossRef CAS PubMed.
- B. S. Rosenstein and D. L. Mitchell, Radiat. Res., 1991, 126, 338–342 CrossRef CAS PubMed.
- D. L. Mitchell, Mutat. Res., 1988, 194, 227–237 CAS.
- S. Courdavault, C. Baudouin, M. Charveron, B. Canguilhem, A. Favier, J. Cadet and T. Douki, DNA Repair, 2005, 4, 836–844 CrossRef CAS PubMed.
- R. Greinert, O. Boguhn, D. Harder, E. W. Breitbart, D. L. Mitchell and B. Volkmer, Photochem. Photobiol., 2000, 72, 701–708 CrossRef CAS PubMed.
- S. Courdavault, C. Baudouin, S. Sauvaigo, S. Mouret, S. Candéias, M. Charveron, A. Favier, J. Cadet and T. Douki, Photochem. Photobiol., 2004, 79, 145–151 CrossRef CAS PubMed.
- J. T. Reardon, A. F. Nichols, S. Keeney, C. A. Smith, J. S. Taylor, S. Linn and A. Sancar, J. Biol. Chem., 1993, 268, 21301–21308 CAS.
- D. L. Svoboda, C. A. Smith, J. S. A. Taylor and A. Sancar, J. Biol. Chem., 1993, 268, 10694–10700 CAS.
- A. M. Avery, B. Kaur, J. S. Taylor, J. A. Mello, J. M. Essigmann and P. W. Doetsch, Nucleic Acids Res., 1999, 27, 2256–2264 CrossRef CAS PubMed.
- S. T. Kim, K. Malhotra, C. A. Smith, J. S. Taylor and A. Sancar, J. Biol. Chem., 1994, 269, 8535–8540 CAS.
- K. Hitomi, S. T. Kim, S. Iwai, N. Harima, E. Otoshi, M. Ikenaga and T. Todo, J. Biol. Chem., 1997, 272, 32591–32598 CrossRef CAS PubMed.
- X. D. Zhao, J. Q. Liu, D. S. Hsu, S. Y. Zhao, J. S. Taylor and A. Sancar, J. Biol. Chem., 1997, 272, 32580–32590 CrossRef CAS PubMed.
- J. Yamamoto, K. Hitomi, T. Todo and S. Iwai, Nucleic Acids Res., 2006, 34, 4406–4415 CrossRef CAS PubMed.
- A. F. Glas, S. Schneider, M. J. Maul, U. Hennecke and T. Carell, Chem. – Eur. J., 2009, 15, 10387–10396 CrossRef CAS PubMed.
- A. F. Glas, E. Kaya, S. Schneider, K. Heil, D. Fazio, M. J. Maul and T. Carell, J. Am. Chem. Soc., 2010, 132, 3254–3255 CrossRef CAS PubMed.
- Y. S. Wang, P. P. Gaspar and J. S. Taylor, J. Am. Chem. Soc., 2000, 122, 5510–5519 CrossRef CAS.
- Y. J. Ai, R. Z. Liao, S. L. Chen, W. J. Hua, W. H. Fang and Y. Luo, J. Phys. Chem. B, 2011, 115, 10976–10982 CrossRef CAS PubMed.
- B. P. Fingerhut, K. Heil, E. Kaya, S. Oesterling, R. de Vivie-Riedle and T. Carell, Chem. Sci., 2012, 3, 1794–1797 RSC.
- D. L. Mitchell and B. S. Rosenstein, Photochem. Photobiol., 1987, 45, 781–786 CrossRef CAS PubMed.
- T. Matsunaga, Y. Hatakeyama, M. Ohta, T. Mori and O. Nikaido, Photochem. Photobiol., 1993, 57, 934–940 CrossRef CAS PubMed.
- H. Morioka, M. Kurihara, H. Kobayashi, K. Satou, Y. Komatsu, M. Uchida, E. Ohtsuka, T. Torizawa, K. Kato, I. Shimada, T. Matsunaga and O. Nikaido, Nucleosides, Nucleotides Nucleic Acids, 2006, 25, 667–679 CAS.
- T. Douki, Photochem. Photobiol. Sci., 2013, 12, 1286–1302 CAS.
- P. H. Clingen, C. F. Arlett, L. Roza, T. Mori, O. Nikaido and M. H. L. Green, Cancer Res., 1995, 55, 2245–2248 CAS.
- T. Douki, A. Reynaud-Angelin, J. Cadet and E. Sage, Biochemistry, 2003, 42, 9221–9226 CrossRef CAS PubMed.
- D. Bacqueville, T. Douki, L. Duprat, S. Rebelo-Moreira, B. Guiraud, H. Dromigny, V. Perier, S. Bessou-Touya and H. Duplan, J. Photochem. Photobiol., B, 2015, 151, 31–38 CrossRef CAS PubMed.
- Y. Takeuchi, M. Murakami, N. Nakajima, N. Kondo and O. Nikaido, Plant Cell Physiol., 1998, 39, 745–750 CrossRef CAS.
- U. P. De la Vega, P. Rettberg, T. Douki, J. Cadet and G. Horneck, Int. J. Radiat. Biol., 2005, 81, 601–611 CrossRef PubMed.
- R. Moeller, E. Stackebrandt, T. Douki, J. Cadet, P. Rettberg, H. J. Mollenkopf, G. Reitz and G. Horneck, Arch. Microbiol., 2007, 188, 421–431 CrossRef CAS PubMed.
- R. Moeller, T. Douki, P. Rettberg, G. Reitz, J. Cadet, W. L. Nicholson and G. Horneck, Arch. Microbiol., 2010, 192, 521–529 CrossRef CAS PubMed.
- X. S. Qin, S. M. Zhang, M. Zarkovic, Y. Nakatsuru, S. Shimizu, Y. Yamazaki, H. Oda, O. Nikaido and T. Ishikawa, Jpn. J. Cancer Res., 1996, 87, 685–690 CrossRef CAS PubMed.
- C. A. Chadwick, C. S. Potten, O. Nikaido, T. Matsunaga, C. Proby and A. R. Young, J. Photochem. Photobiol., B, 1995, 28, 163–170 CrossRef CAS.
- P. H. Clingen, C. F. Arlett, J. Cole, A. P. W. Waugh, J. E. Lowe, S. A. Harcourt, N. Hermanova, L. Roza, T. Mori, O. Nikaido and M. H. L. Green, Photochem. Photobiol., 1995, 61, 163–170 CrossRef CAS PubMed.
- S. Mouret, C. Baudouin, M. Charveron, A. Favier, J. Cadet and T. Douki, Proc. Natl. Acad. Sci. U. S. A., 2006, 103, 13765–13770 CrossRef CAS PubMed.
- Y. H. You, D. H. Lee, J. H. Yoon, S. Nakajima, A. Yasui and G. P. Pfeifer, J. Biol. Chem., 2001, 276, 44688–44694 CrossRef CAS PubMed.
|
This journal is © The Royal Society of Chemistry and Owner Societies 2016 |
Click here to see how this site uses Cookies. View our privacy policy here.