Tuning the concentration of dye loaded polymer films for maximum photosensitization efficiency: phloxine B in poly(2-hydroxyethyl methacrylate)
Received
29th September 2015
, Accepted 30th November 2015
First published on 2nd December 2015
Abstract
Fluorescence and singlet molecular oxygen (1O2) quantum yields for phloxine B loaded poly(2-hydroxyethyl methacrylate) thin films are determined at dye concentrations from 0.015 to 22 wt%. Fluorescence self-quenching and the fall off of the 1O2 quantum yield observed above 0.1 wt% are attributed to very weakly interacting close-lying dye molecules acting as energy traps arising from molecular confinement. The maximum singlet oxygen generation efficiency (quantum yield × absorption factor) lies at concentrations around 2 wt%, where fluorescence self quenching amounts to more than 80%. Data are fitted quantitatively by using a quenching radius model involving energy migration and trapping with rQ = 1.2 nm. The present results constitute a proof of concept for the rational design of heterogeneous photosensitizers in general and, particularly, for applications in which the antimicrobial activity of singlet oxygen is central.
Introduction
Research on dye loaded polymer films covers different fields of practical interest such as optical chemical sensing,1 development of laser media,2 high density optical data storage,3 security authentication,4 light trapping for water splitting devices,5 and singlet molecular oxygen (1O2) photosensitization,6 among others. For certain applications such as optical storage and photosensitization, small dye-to-dye distances or large absorbances, attainable at high concentrations, are required. However, interactions among the dye molecules play a negative role in this case because chromophores lose their individuality and new channels for radiationless deactivation become operative. Eventually, organic dyes aggregate with formation of photochemically inactive dimers and oligomers. Interactions with the polymer backbone weaken ground state dye-to-dye interactions7 but they are generally ineffective in avoiding the coupling of excited state chromophores and ground state molecules. As a consequence, excitation energy trapping is produced also in cases where no dye aggregation is spectroscopically evidenced.8 As energy trapping increases at high loadings faster than the absorption factor,† there is a practical dye concentration which maximizes energy exploitation. In spite of this, very few quantitative studies have been performed in polymers at different dye loadings in order to understand, and consequently to manage dye aggregation and energy wasting. Mirenda et al. studied Rose Bengal (RB) aggregation in polyelectrolyte aqueous alkaline solutions and layer-by-layer self-assembled thin films of positively charged poly[diallyldimethylammonium] chloride and negatively charged RB.9 At dye concentrations on the order of 1 M, though aggregation and energy trapping are unavoidable, measurable quantum yields of fluorescence, ΦF, and singlet oxygen production, ΦΔ, have been observed. A concentration dependence study was not possible in that case because self-assembly is ineffective at lower dye concentrations. Priimagi et al. investigated different polymers at high dye loadings and measured absorption spectral changes on increasing the dye concentration in order to evaluate dye aggregation.7 However, their results do not allow the quantification of energy wasting by dark excimer formation and other ways of energy trapping.
In this work, phloxine B (PhB) was loaded into poly(2-hydroxyethyl methacrylate) (pHEMA), a bio-compatible polymer that forms hydrogels in water and, conveniently cross-linked, builds up hardly hydrolysable materials, which are mainly used in the production of contact lenses.10,11 PhB is a hydrophilic dye with low toxicity, used as a color additive in foods, drugs and cosmetic formulations, with ΦΔ = 0.65 in water12 and a low tendency to aggregate when included into microcrystalline cellulose particles.13 The dye is phototoxic for Gram-positive and Gram-negative bacteria,14,15 insects16 and individual cells of higher organisms.17,18 It is potentially useful for the prevention of endophthalmitis after cataract surgery through bonding to the surface of intraocular lenses, in analogy to the case of tetrakis(4-N-methylpyridyl)porphyrin, which was electrostatically bound to the surface methacrylate groups of pHEMA-co-(methacrylic acid).19 Other possible application fields are the development of photoactive wound dressings for medicine and cosmetic applications and coatings for disposable materials, hospital doors and medical equipment, where the antimicrobial activity of singlet oxygen at the material surface is central.
Photophysical properties of PhB loaded pHEMA films, i.e. ΦF and ΦΔ, are determined as a function of dye concentration in order to obtain a practical concentration which maximizes the production of 1O2. The results are interpreted in the context of a quenching radius model (QRM) and calculation of energy migration and trapping rates based on Loring, Andersen and Fayer (LAF) theory.20,21
Experimental section
Chemicals and preparation of films
Phloxine B disodium salt (PhB), certified dye purity 89%, and poly(2-hydroxyethyl methacrylate) (pHEMA), 20
000 kDa, density 1.15 g mL−1 at 25 °C, were obtained from Sigma-Aldrich and used as received. Analytical grade ethanol and acetone from Biopak were used without further purification. Microscope slides were cleaned by ultrasonication in acetone, ethanol and water, 30 min in each solvent. Thin films were prepared by spin coating from 20 mg mL−1 or 30 mg mL−1 ethanolic solutions of pHEMA with different PhB concentrations, using a spin coater WS-400B-GNPP/Lite (Laurell Technologies) at increasing acceleration, reaching an angular speed of 2500 rpm after 30 s. Reproducible thicknesses either of (150 ± 15) or (245 ± 15) nm were determined by stylus profilometry using a Dektak 150 Profilometer (Veeco). Thin films with PhB concentrations spanning more than three orders of magnitude, from 0.015 to 22 wt%, were prepared. Dye molar concentrations, needed for the calculation of absorption coefficients and application of the QRM, were calculated as [PhB] = nρ/m, where n/m is the ratio between the number of moles of PhB and the mass of the film and ρ is its density. As a working approximation, valid at n/m → 0, the mass and the density (1.15 g cm−3) of the pure solid polymer were used. Within this approximation level, | [PhB] = (ρ wt%/M)/(100 − wt%) | (1) |
where M is the molar mass of PhB (829.63 g mol−1), leading to the concentration range 0.0002 to ca. 0.4 M. Thick films were prepared from the same solutions, depositing sequentially three layers over microscope slides and evaporating the solvent at room temperature. Thicknesses of 16 ± 3 μm were estimated assuming that the absorption coefficient is the same as in thin films. In all cases optically clear films were obtained.
Photophysical characterization
Absorption spectra were recorded on a Shimadzu UV-3600 spectrophotometer. Measurements were performed by placing the film in a home-made holder which allows horizontal and vertical displacements in order to evaluate the absorption at different positions. Steady-state emission spectra were recorded on a PTI model QM-4 spectrofluorometer. Films were measured in back face with normal illumination (λexc = 505 nm) and a right-angle triangular prism (PS911 Thorlabs N-BK7) was used to drive the emitted light into the detection channel. A suitable optical filter (Schott, OG515, 0.2 cm thickness) was positioned in front of the detector to block excitation stray light, which was further reduced changing the position of the emission lens. Spectra were corrected according to the dependence of the detection responsivity on the wavelength obtained from the manufacturer and checked in our laboratory. Thin film ΦF values were determined in reference to 0.5–1 μM PhB in ethanol in a 1 mm pathlength quartz cuvette, whereas the reference ΦF values were determined against Rhodamine 101 in the same solvent. This setup was selected to avoid irreproducibility issues inherent to measurements in the front face or the back face when films are positioned angularly with respect to the normal to the excitation beam, which cause erratic quantum yield results. The difference in sample and reference geometries causes a negligible uncertainty in the measurement. Considering that pHEMA and glass have similar refractive indexes and according to Fresnel's law for normal incidence, reflections at the two extra quartz–ethanol interfaces in the reference lead to an overestimation of ΦF for the films on the order of only 0.5%.
Determination of singlet oxygen quantum yields was performed using 1,3-diphenylisobenzofuran (DPBF, Aldrich) in dichloromethane (DCM, Cicarelli, analytical grade) as a chemical monitor.22,23 The absorbance of DPBF was monitored at 415 nm. A 0.1 μM methylene blue (Química Bonaerense, analytical grade) solution in DCM was used as the reference (ΦΔ = 0.57). A halogen lamp (OSRAM HLX 64657, 250 W, 24 V) was used for illumination. A water filter and two glass filters (Schott, KG5, 0.2 cm thickness) were interposed between the lamp and the film in order to block the IR component of the light source. A second pair of filters (Schott, OG495 and OG515, 0.2 cm thickness) was used to avoid direct excitation of the monitor. The emission spectrum of the lamp and filter transmittance were taken into account in order to calculate the fraction of absorbed light. Experiments were carried out on dry and humidified films, the latter pretreated with a flow of water saturated oxygen.
Laser flash photolysis (LFP) measurements were performed on thick films. A Nd-YAG laser (Spectron, 8 ns @ 532 nm) and a horizontally driven Xe lamp (OSRAM XBO 150 W/1 OFR) were used for excitation and analysis, respectively. The film was placed inside a quartz cuvette in a LP920 laser flash photolysis compartment (Edinburgh Instruments) at an angle of 45° with respect to both light sources. Correct overlapping of both beams on the film surface was ensured. Two filters (Schott, GG475, 0.2 and 0.3 cm thickness) were placed in front of the film to block unwanted analysis light below 450 nm to avoid dye bleaching. Laser-induced luminescence (LIL) experiments were carried out with the same setup blocking analysis light. The analysis light in LFP and the emitted light in LIL experiments were detected using a photomultiplier tube (PMT Hamamatsu R929), after passing through appropriate filters (Schott, OG580, 0.2 cm thickness and Schott, OG590, 0.3 cm thickness) and a computer controlled high throughput 1/4 m f/2.5 monochromator (Sciencetech 9055F). To increase the signal-to-noise ratio, currently 64 traces were averaged. Analysis wavelengths were 610 nm for LFP and 695 nm for LIL. They were selected as a compromise between the signal-to-noise ratio, interference of the laser beam, interference of phosphorescence in LFP and dye photobleaching. The system was purged either with dry or water saturated oxygen or water saturated argon.
Quenching radius model
The quenching radius model used to fit ΦF against the dye concentration was similar to that applied elsewhere8 to evaluate the self-quenching of Rhodamine 6G adsorbed on microcrystalline cellulose. In the present case a 3D random distribution of dye molecules was assumed. Traps were identified as pairs of molecules within a distance ≤rQ, the quenching radius, and Poisson statistics was used to estimate the monomer and trap concentrations. Energy migration and trapping rates were calculated using Loring, Andersen and Fayer (LAF) theory under the assumption of non-fluorescing, perfect traps.20,21 Förster radii were calculated as R0,M–M = 52.3 Å and R0,M–T = 58.7 Å for monomer–monomer and monomer–trap energy transfer, respectively. An orientational parameter κ2 = 0.476 (randomly distributed orientations fixed in time) and a refractive index n = 1.51 for pHEMA24 were considered. R0,M–T was calculated considering that the absorption coefficient of traps is twice the absorption coefficient of the monomeric dye, consistent with the traps arising from weakly interacting ground state dye molecules.
Results and discussion
Absorption and emission spectra at different dye concentrations are shown in Fig. 1. Both spectra show constant shapes for films containing up to 10 wt% PhB. For more concentrated films, minimal changes are observed on increasing the dye concentration, namely a small increase of the absorption shoulder relative to the main band and small red shifts in the main band and some distortions in the long wavelength band of fluorescence spectra. Changes in fluorescence spectra at the highest concentrations can be partly attributed to small reabsorption effects. In the figure inset a linear relationship between Amax/d and concentration, where Amax is the absorbance at maximum, (552 ± 1) nm, and d is the film thickness, is observed above 1 wt% (slope 1 in the log–log plot). From these results, the PhB absorption coefficient in the films and the absorbance of very dilute films, which could not be experimentally determined, were calculated. The absorption coefficient, (9.9 ± 0.2) × 104 M−1 cm−1 at 552 nm, is similar to the value measured in ethanol solution, 9.87 × 104 M−1 cm−1 at the same wavelength. Absorption spectra were recorded against a film devoid of the dye. Films were masked to a small area and spectra were determined at different positions for the same film. No significative differences were observed. Fluorescence spectra (λexc = 505 nm) were recorded in back face and quantum yields were calculated against PhB ethanolic solutions in a 1 mm pathlength regular absorption cell (see the Experimental section).
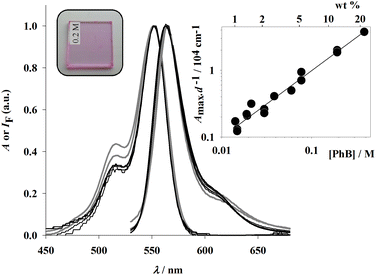 |
| Fig. 1 PhB–pHEMA films absorption and emission (λexc = 505 nm) spectra at PhB concentrations 21.7; 12.2; 5.2; 4.1; 2.7; and 2.1 wt%. Gray lines correspond to the two most concentrated films. Inset: absorbance at maximum, (552 ± 1) nm, per unit pathlength as a function of concentration for 150 and 245 nm thick films. | |
Fluorescence quantum yields are shown as a function of dye concentration in Fig. 2. Up to ca. 0.3 wt% PhB, the values are similar, within experimental uncertainty, to that obtained in ethanol solution (ΦF = 0.76 ± 0.02). For more concentrated films a dramatic decrease is observed until undetectable values are observed above ca. 7 wt% PhB.
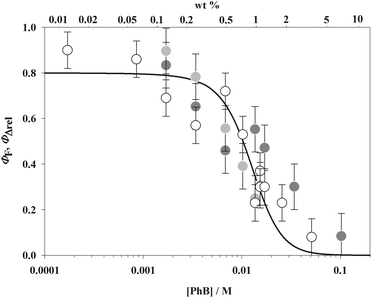 |
| Fig. 2 Fluorescence (hollow circles) and scaled singlet oxygen quantum yields in dry (dark gray circles) and humidified (light gray circles) 245 nm thick films as a function of PhB concentration. The solid line is a fit according to a quenching radius model and LAF theory calculations (see text). | |
As a chemical monitor, 1,3-diphenylisobenzofuran (DPBF) in dichloromethane (DCM) solution was used to estimate singlet molecular oxygen quantum yields. Its absorbance at λ = 415 nm decreases when the film is immersed in the solution and irradiated at wavelengths within the absorption range of PhB. No change was observed in the dark and a negligible decrease was detected under illumination in the absence of the film. No desorption of PhB was observed in all cases. The oxygen diffusion coefficient in hydrated (35 vol%) pHEMA crosslinked with tetraethylene glycol dimethacrylate25 is D = 1.36 × 10−7 cm2 s−1 and the 1O2 lifetime in the polymer can be estimated as τ ≈ 20 μs.26 Assuming that these values apply to the system under study and according to the Brownian motion equation in one-dimension, <x2> = 2Dτ, the average distance traveled by a 1O2 molecule during time τ is around 23 nm. This value implies that only a fraction of the total singlet oxygen formed, roughly 10% for 245 nm thick films, is able to reach the film surface and react with the chemical monitor. Therefore, a strongly underestimated, effective value of ΦΔ is obtained. Thinner films can, in principle, be considered but the uncertainty in absorbance determination would be too high. Nevertheless the measured values are proportional to the actual quantum yields. The results show a marked decrease of ΦΔ with the PhB concentration and a large, nearly 10-fold increase when films are humidified with water saturated oxygen prior to immersion in DCM, approaching at low PhB concentration 0.01 for humidified films and 0.001 for dry films. Humidity induces swelling of the polymer matrix, increasing the O2 diffusion coefficient and, consequently, increasing the effective ΦΔ values. Recall that actual ΦΔ values cannot exceed 1 − ΦF ≈ 0.20, much lower than in water (see above). This is in accordance with the general behavior of xanthene dyes, which strongly increase their ΦF and accordingly reduce ΦT values when passing from water to environments with less proton donating capacity.27 In Fig. 2, ΦΔ values scaled to match absolute ΦF values at low concentrations are displayed. It may be seen that both quantum yields follow similar trends with the concentration.
The formation of the PhB triplet state was evidenced by laser flash photolysis and time resolved phosphorescence analysis. As a consequence of the small absorption of thin films, thick films (∼16 μm) were used in these experiments (see the Experimental section). Transient decays are shown in Fig. 3. After purging the film with humidified argon, similar triplet absorption and phosphorescence signals are obtained, which can be fitted by a biexponential function with decay times of 1.6 (∼50%) and 3.1 (∼50%) ms. After purging with humidified oxygen the amplitude of both signals decreases up to a negligible value (triplet signal reported in the figure). This behavior is consistent with triplet detection in the absence of oxygen and quantitative triplet quenching in its presence. Saturation with water is needed in order to swell the film and activate transport of the purging gas.28 Purging a previously oxygenated film with dry oxygen has the consequence of partially recovering the triplet and phosphorescence signals, showing that drying leads to an important deoxygenation of the film even in the presence of gaseous oxygen.
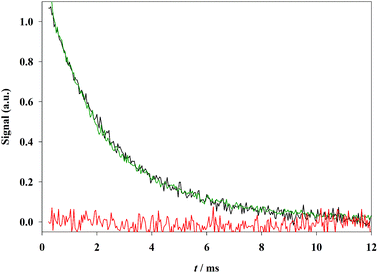 |
| Fig. 3 Phosphorescence intensity measured at 695 nm (black) and triplet–triplet absorption measured at 610 nm (green) after purging with humidified argon and triplet signal (red) after purging with humidified oxygen obtained from a thick film containing 0.7 wt% in PhB (see text). | |
The tendency of xanthene dyes to aggregate increases with the number and polarizability of halogen atoms.29 PhB, together with eosin Y, erythrosin B and RB, belongs to the xanthenes containing heavy halogens and, therefore, they aggregate strongly in water and ethanol solutions, with important changes in the absorption spectrum even at concentrations below 10−4 M. Our results show that deviations of the absorption spectrum do not take place in pHEMA even at three orders of magnitude larger concentrations. A similar tendency has been observed for xanthene dyes adsorbed on cellulose.30 However, the lack of spectroscopically detectable aggregates does not guarantee the absence of concentration quenching. López et al. applied a QRM in their study on the dependence of ΦF on dye loading for Rhodamine 6G adsorbed on cellulose microparticles.8 Dye molecules are distributed randomly in space and, below a critical radius, rQ ≈ 1.2–1.5 nm, dye pairs act as non-fluorescing energy traps. The interaction between dye molecules in traps is too weak to cause detectable spectroscopic changes. In this work, the model applied to the system PhB–pHEMA (see the Experimental section) yields a similar value, rQ = 1.2 nm. The agreement between the ΦF values calculated by the QRM and the experimentally determined ΦF and scaled ΦΔ values against the PhB concentration (Fig. 2) shows that energy traps quench the excited singlet state, blocking simultaneously triplet state formation. The quenching is of both static (light absorption by traps) and dynamic (energy transfer from the excited nearly isolated dye molecules to traps) nature.
In ref. 7 Priimagi et al. claimed that the interaction between dye molecules and the polymer chain inhibits the dye aggregation tendency and allows high loadings to be used, enhancing the performance of the film. However, as we demonstrate in this work, the lack of spectroscopic evidence of aggregation does not imply the absence of energy wasting processes because statistical traps cannot be avoided for random dye distributions. It is clear from our results that working at unnecessarily high dye concentrations has detrimental effects. The singlet oxygen generation efficiency can be calculated as the product between ΦΔ and the absorption factor. However, considering that the scaled ΦΔ values follow the same trend as ΦF and the latter quantum yields are obtained with higher accuracy, the results on ΦF were used to estimate the maximum efficiency. As shown in Fig. 4, the maximum singlet oxygen generation efficiency lies at dye concentrations on the order of 2 wt%, where fluorescence self quenching amounts to more than 80% (see Fig. 2). It is also evident that if the objective is the production of singlet molecular oxygen as a reactant for targets in solution, film thicknesses more than a few tens of nm are not necessary.
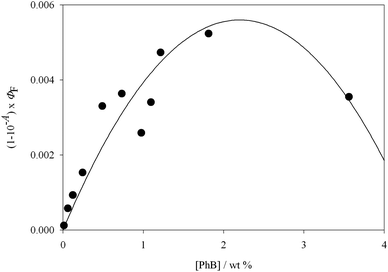 |
| Fig. 4 Excited state generation efficiency, calculated as the product between the absorption factor and the fluorescence quantum yield, as a function of PhB concentration for 245 nm thin films. The line is a quadratic function without physical meaning. | |
Conclusions
Interactions leading to energy trapping are common in arrays of dye molecules at high concentrations and constitute one of the main factors in lowering the efficiency of heterogeneous photosensitizers. Our results show that the product between the absorption factor and the fluorescence quantum yield increases up to a certain dye concentration and begins to decrease thereafter. For random arrays, the concentration which maximizes the product is the optimum value. This concentration is in turn determined by the quenching radius, rQ, whose knowledge helps the rational design through the application of suitable models. In an ordered sequence of dye molecules, the quenching radius represents the minimum distance at which dye molecules may be located. Film thickness is also relevant when the action of the photosensitizer is restricted to its surface. If the objective is to generate singlet molecular oxygen, its diffusion length determines the maximum film thickness, which must be considered to avoid unnecessary reactive species to be produced in the bulk. The present results can be regarded as a proof of concept for the development of heterogeneous photosensitizers in general, and particularly for photoactive coatings with antimicrobial activity.
Acknowledgements
This work was supported by the University of Buenos Aires, the National Research Council of Argentina (CONICET) and the Agencia Nacional de Promoción Científica y Tecnológica (ANPCyT). H. B. R. and E. S. R. are staff members of CONICET. Y. L. acknowledges a fellowship from the Consejo Interuniversitario Nacional (CIN).
References
-
F. Baldini, A. N. Chester, J. Homola and S. Martellucci, Optical Chemical Sensors, Springer, Dordrecht, 2006 Search PubMed.
- Y. Huang and S.-T. Wu, Multi-Wavelength Laser from Dye-Doped Cholesteric Polymer Films, Opt. Express, 2010, 18, 27697–27702 CrossRef CAS PubMed.
- S. Kawata and Y. Kawata, Three-Dimensional Optical Data Storage Using Photochromic Materials, Chem. Rev., 2000, 100, 1777–1788 CrossRef CAS PubMed.
-
R. Waning and S. Muke, Authentication Means. PCT Int. Pat., WO2004087430, 2004 Search PubMed.
- H. Dotan, O. Kfir, E. Sharlin, O. Blank, M. Gross, I. Dumchin, G. Ankonina and A. Rothschild, Resonant Light Trapping in Ultrathin Films for Water Splitting, Nat. Mater., 2013, 12, 158–164 CrossRef CAS PubMed.
- M. Nowakowska, M. Kepczynski and K. Szczubialka, New Polymeric Photosensitizers, Pure Appl. Chem., 2001, 73, 491–495 CrossRef CAS.
- A. Priimagi, S. Cattaneo, R. H. A. Ras, S. Valkama, O. Ikkala and M. Kauranen, Polymer-Dye Complexes: A Facile Method for High Doping Level and Aggregation Control of Dye Molecules, Chem. Mater., 2005, 17, 5798–5802 CrossRef CAS.
- S. G. López, G. Worringer, H. B. Rodríguez and E. San Román, Trapping of Rhodamine 6G Excitation Energy on Cellulose Microparticles, Phys. Chem. Chem. Phys., 2010, 12, 2246–2253 RSC.
- M. Mirenda, C. A. Strassert, L. E. Dicelio and E. San Román, Dye-Polyelectrolyte Layer-by-Layer Self-Assembled Materials: Molecular Aggregation, Structural Stability, and Singlet Oxygen Photogeneration, ACS Appl. Mater. Interfaces, 2010, 2, 1556–1560 CAS.
- A. Opdahl, S. H. Kim, T. S. Koffas, C. Marmo and G. A. Somorjai, Surface Mechanical Properties of pHEMA Contact Lenses: Viscoelastic and Adhesive Property Changes on Exposure to Controlled Humidity, J. Biomed. Mater. Res., Part A, 2003, 67, 350–356 CrossRef PubMed.
- C. Maldonado-Codina and N. Efron, Dynamic Wettability of pHEMA-Based Hydrogel Contact Lenses, Ophthal. Physiol. Opt., 2006, 26, 408–418 CrossRef PubMed.
- E. Gandin, Y. Lion and A. Van de Vorst, Quantum Yield of Singlet Oxygen Production by Xanthene Derivatives, Photochem. Photobiol., 1983, 37, 271–278 CrossRef CAS.
- P. Duarte, D. P. Ferreira, I. Ferreira Machado, L. F. Vieira Ferreira, H. B. Rodríguez and E. San Román, Phloxine B as a Probe for Entrapment in Microcrystalline Cellulose, Molecules, 2012, 17, 1602–1616 CrossRef CAS PubMed.
- A. Rasooly and A. Weisz, In Vitro Antibacterial Activities of Phloxine B and Other Halogenated Fluoresceins Against Methicillin-Resistant Staphylococcus aureus, Antimicrob. Agents Chemother., 2002, 46, 3650–3653 CrossRef CAS PubMed.
- R. Rasooly, Expanding the Bactericidal Action of the Food Color Additive Phloxine B to Gram-Negative Bacteria, FEMS Immunol. Med. Microbiol., 2005, 45, 239–244 CrossRef CAS PubMed.
- D. S. Moreno, H. Celedonio, R. L. Mangan, J. L. Zavala and P. Montoya, Field Evaluation of a Phototoxic Dye, Phloxine B, Against Three Species of Fruit Flies (Diptera: Tephritidae), J. Econ. Entomol., 2001, 94, 1419–1427 CrossRef CAS PubMed.
- H. Qi, H. Takano, Y. Kato, Q. Wu, C. Ogata, B. Zhu, Y. Murata and Y. Nakamura, Hydrogen Peroxide-Dependent Photocytotoxicity by Phloxine B, a Xanthene-Type Food Colorant, Biochim. Biophys. Acta, 2011, 1810, 704–712 CrossRef CAS PubMed.
- H. Qi, B. Zhu, N. Abe, Y. Shin, Y. Murata and Y. Nakamura, Involvement of Intracellular Oxidative Stress-Sensitive Pathway in Phloxine B-Induced Photocytotoxicity in Human T Lymphocytic Leukemia Cells, Food Chem. Toxicol., 2012, 50, 1841–1847 CrossRef CAS PubMed.
- C. P. McCoy, R. A. Craig, S. M. McGlinchey, L. Carson, D. S. Jones and S. P. Gorman, Surface Localisation of Photosensitisers on Intraocular Lens Biomaterials for Prevention of Infectious Endophthalmitis and Retinal Protection, Biomaterials, 2012, 33, 7952–7958 CrossRef CAS PubMed.
- R. F. Loring, H. C. Andersen and M. D. Fayer, Electronic Excited State Transport and Trapping in Solution, J. Chem. Phys., 1982, 76, 2015–2027 CrossRef CAS.
- L. Kulak and C. Bojarski, Forward and Reverse Electronic Energy Transport and Trapping in Solution. II. Numerical Results and Monte Carlo Simulations, Chem. Phys., 1995, 191, 67–86 CrossRef CAS.
- Y. Usui, Determination of Quantum Yield of Singlet Oxygen Formation by Photosensitization, Chem. Lett., 1973, 2, 743–744 CrossRef.
- M. Nowakowska, Solvent Effect on the Quantum Yield of the Self-Sensitized Photoperoxidation of 1,3-Diphenilisobenzofuran, J. Chem. Soc., Faraday Trans. 1, 1984, 80, 2119–2126 RSC.
- A. De Girolamo Del Mauro, A. I. Grimaldi, V. La Ferrara, E. Massera, M. L. Miglietta, T. Polichetti and G. Di Francia, A Simple Optical Model for the Swelling Evaluation in Polymer Nanocomposites, J. Sens., 2009, 703206 Search PubMed.
- J. W. Parker and M. E. Cox, Mass Transfer of Oxygen in Poly(2-Hydroxyethyl Methacrylate), J. Polym. Sci., Part A: Polym. Chem., 1988, 26, 1179–1188 CrossRef CAS.
-
J. F. Rabek, Photodegradation of Polymers: Physical Characteristics and Applications, Springer Verlag, Berlin, 2012, p. 87 Search PubMed.
- G. R. Fleming, A. W. E. Knight, J. M. Morris, R. J. S. Morrison and G. W. Robinson, Picosecond Fluorescence Studies of Xanthene Dyes, J. Am. Chem. Soc., 1977, 99, 4306–4311 CrossRef CAS.
- Y. Tian, B. R. Shumway, W. Gao, C. Youngbull, M. R. Holl, R. H. Johnson and D. R. Meldrum, Influence of Matrices on Oxygen Sensing of Three Sensing Films with Chemically Conjugated Platinum Porphyrin Probes and Preliminary Application for Monitoring of Oxygen Consumption of Escherichia coli (E. coli), Sens. Actuators, B, 2010, 150, 579–587 CrossRef CAS PubMed.
- O. Valdez-Aguilera and D. C. Neckers, Aggregation Phenomena in Xanthene Dyes, Acc. Chem. Res., 1989, 22, 171–177 CrossRef.
- H. B. Rodríguez, E. San Román, P. Duarte, I. Ferreira Machado and L. F. Vieira Ferreira, Eosin Y Triplet State as a Probe of Spatial Heterogeneity in Microcrystalline Cellulose, Photochem. Photobiol., 2012, 88, 831–839 CrossRef PubMed.
Footnote |
† The absorption factor, also called absorptance, is the fraction of radiation absorbed by the film. |
|
This journal is © The Royal Society of Chemistry and Owner Societies 2016 |