DOI:
10.1039/C5NR07810E
(Communication)
Nanoscale, 2016,
8, 1299-1304
Medium-sized Au40(SR)24 and Au52(SR)32 nanoclusters with distinct gold-kernel structures and spectroscopic features†
Received
7th November 2015
, Accepted 8th December 2015
First published on 9th December 2015
Abstract
We have analyzed the structures of two medium-sized thiolate-protected gold nanoparticles (RS-AuNPs) Au40(SR)24 and Au52(SR)32 and identified the distinct structural features in their Au kernels [Sci. Adv., 2015, 1, e1500425]. We find that both Au kernels of the Au40(SR)24 and Au52(SR)32 nanoclusters can be classified as interpenetrating cuboctahedra. Simulated X-ray diffraction patterns of the RS-AuNPs with the cuboctahedral kernel are collected and then compared with the X-ray diffraction patterns of the RS-AuNPs of two other prevailing Au-kernels identified from previous experiments, namely the Ino-decahedral kernel and icosahedral kernel. The distinct X-ray diffraction patterns of RS-AuNPs with the three different types of Au-kernels can be utilized as signature features for future studies of structures of RS-AuNPs. Moreover, the simulated UV/Vis absorption spectra and Kohn–Sham orbital energy-level diagrams are obtained for the Au40(SR)24 and Au52(SR)32, on the basis of time-dependent density functional theory computation. The extrapolated optical band-edges of Au40(SR)24 and Au52(SR)32 are 1.1 eV and 1.25 eV, respectively. The feature peaks in the UV/Vis absorption spectra of the two clusters can be attributed to the d → sp electronic transition. Lastly, the catalytic activities of the Au40(SR)24 and Au52(SR)32 are examined using CO oxidation as a probe. Both medium-sized thiolate-protected gold clusters can serve as effective stand-alone nanocatalysts.
Introduction
Thiolate-protected gold nanoclusters (RS-AuNPs) have received considerable attention over the past decade owing to their unique structures and physicochemical properties compared with other types of gold nanoparticles,1–7 and their potential applications in catalysis, nanotechnology, and biomedicine, among others.8–11 To date, the total atomic structures of a number of RS-AuNPs, including Au18(SC6H11)14,12 Au20(TBBT)16 (TBBT = SPh-p-tBu),13 Au24±1(SAdm)16,14 Au25(PET)18−1/0 (PET = phenylethylthiolate SCH2CH2Ph),15–17 Au28(TBBT)20,18 Au30S(S-t-Bu)18,19 Au36(TBBT)24,20 Au38(PET)24,21 Au102(p-MBA)44 (p-MBA = p-mercaptobenzoic acid, SC7O2H5),22 Au130(p-MBT)50 (p-MBT = para-methylbenzenethiol),23 Au133(TBBT)52,24,25etc. have been successfully resolved via X-ray crystallography. Among these clusters, Au102(p-MBA)44 and Au25(PET)18−1/0 were the first two crystallized and characterized in 2007 and 2008, respectively. The total structure determination of Au102(p-MBA)44 and Au25(PET)18−1/0 provides profound insights into many new characteristics such as gold–sulfur bonding, atomic packing structure in RS-AuNPs, staple motifs of ligands, as well as some generic rules of Au distribution among the Au-kernels and ligands. Until now, Au133(TBBT)52 is the largest ligand-covered gold cluster whose crystal structure has been obtained.
Although tremendous progress has been made in the structural determination of RS-AuNPs from small to large-size via X-ray crystallography, the crystal structures in the medium-size range from Au38(PET)24 to Au102(p-MBA)44 are largely unknown. The lack of crystal structures of medium-sized thiolate-protected gold clusters hinders the understanding of the structure–property relationship for many known stable clusters, such as Au44(TBBT)28,26 Au55(PET)31,27 Au64(SC6H11)32,28 Au67(PET)35,29 Au75(SC6H13)40,30 Au76(4-MEBA)44 (4-MEBA = 4-(2-mercaptoethyl)benzoic acid),31 and Au99(SPh)42,32,33 all synthesized or isolated in the laboratory. Recently, Azubel et al. applied single-particle transmission electron microscopy (SP-TEM) to determine the positions of 68 Au atoms without a single crystal of Au68(3-MBA)32.34 However, this SP-TEM measurement still requires theoretical input on the protection ligands to achieve the total structure of the cluster.
Very recently, two medium-sized clusters, Au40(o-MBT)24 and Au52(TBBT)32, have been successfully crystallized by Zeng et al.35 It was found that both nanoclusters are composed of 4-atom tetrahedral units which can coil up into a Kekulé-like ring in the Au40(o-MBT)24 (o-MBT = ortho-methylbenzenethiol) cluster or a DNA-like double helix in Au52(TBBT)32. In particular, the Au52(TBBT)32 cluster is a new species to the RS-AuNP family, while the Au40(o-MBT)24 cluster is akin to the known phenylethylthiolate-capped Au40(PET)24 cluster which is an intermediate in the synthesis of the Au38(PET)24 cluster.36,37 However, the marked differences in the absorption spectrum indicate that Au40(o-MBT)24 and Au40(PET)24 have different structures.38 The Kekulé-like ring in Au40(o-MBT)24 is completely different from the Au26 bi-icosahedral core in the Au40(PET)24 originally proposed by Malola et al.39 The experimental work by Zeng et al. sheds new light on the total structures of RS-AuNPs within the range of Au38(PET)24 and Au102(p-MBA)44.35
In this communication, we perform an in-depth kernel structure analysis of Au40(o-MBT)24 and Au52(TBBT)32. We classify all known RS-AuNPs into three groups: (1) cuboctahedra, (2) Ino-decahedra, and (3) icosahedra. The calculated powder X-ray diffraction (XRD) results reveal different characteristic peaks for the Au-kernel structures in these groups, which can be useful in identifying the structures of gold nanoparticles even when the crystal structure is lacking. In addition, the computed optical absorption spectra of Au40(o-MBT)24 and Au52(TBBT)32 are found to be consistent with experimental measurements. Lastly, the catalytic properties of both clusters are examined by using CO oxidation as a probe.
Computational methods
The theoretical powder X-ray diffraction (XRD) curve is calculated using the Debye formula:
where s is the diffraction vector length and θ is the scattering angle, satisfying s = 2sin
θ/λ. λ and α are determined by the experimental setup and are set to be 0.1051967 nm and 1.01, respectively. B is the damping factor, which reflects thermal vibrations, and is set to be 0.03 nm2. The corresponding atomic numbers are used for the scattering factors fi. dij is the distance between atoms i and j. The atomic distance dij is taken from the optimized structure of clusters based on the density functional theory (DFT) code DMol3 (version 7.0).40,41 The generalized gradient approximation with the Perdew–Burke–Ernzerhof (PBE)42 functional and the double numeric polarized (DNP) basis set coupled with semi-core pseudo-potential are employed. Self-consistent calculations are done with a convergence criterion of 10−5 hartree for the total energy. The linear and quadratic synchronous transit (LST/QST) method is used to locate the transition state of CO oxidation on the Au40(o-MBT)24 and Au52(TBBT)32.43–48 In all calculations, the o-MBT in Au40(o-MBT)24, TBBT in Au52(TBBT)32, and the ligands of other RS-AuNPs are simplified by methyl groups to lower computation cost.
Results and discussion
To understand the structure formation of Au40(SR)24 and Au52(SR)32, their kernel structures are analyzed first. The formation of the Au34 kernel of Au40(SR)24 can be divided into two steps, with the Au13 cuboctahedra as building blocks, as shown in Fig. 1. In step 1, the two Au13 cuboctahedra interpenetrate each other to form a Au20 structure by sharing six Au atoms. This rod-like Au20 geometry has been found as a kernel in the structures of Au28(SR)20 and Au30S(SR)18.18,19 Next, one of the Au13 cuboctahedra in the Au20 interpenetrates with the other two Au13 cuboctahedra in the same way as in step 1 to form the kernel of Au34 with a fcc-like structure. Likewise, as shown in Fig. 2, the formation of the Au44 kernel of Au52(SR)32 can be divided into three steps in the same way as Au40 in Au40(SR)24. In step 1 three Au20 geometries interpenetrate each other to form an Au25 structure. Next, the Au25 structure interpenetrates with another Au13 cuboctahedra to form a Au28 geometry, a kernel found in the structure of Au36(SR)24.17 In step 3, the Au44 kernel with fcc-like symmetry can be formed through two interpenetrating Au28 geometries. The above structure analysis on the kernels of Au40(SR)24 and Au52(SR)32, as well as Au28(SR)20, Au30S(SR)18, and Au36(SR)24 (ESI Fig. S1†), shows that the Au kernels of all these clusters with fcc-like symmetry are comprised of several interpenetrating cuboctahedra.
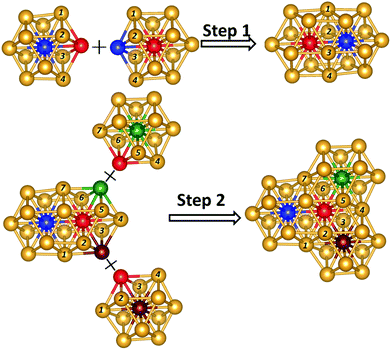 |
| Fig. 1 The formation of Au34 kernel in Au40(SR)24. The Au atoms marked with the same number and color can be fused together. | |
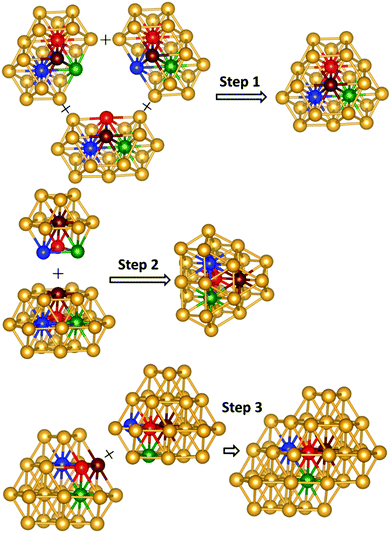 |
| Fig. 2 The formation of Au44 kernel in Au52(SR)32. The Au atoms marked with the same color can be fused together. | |
Besides Au28(SR)20, Au30S(SR)18, Au36(SR)24, Au40(SR)24, and Au52(SR)32, structure analysis for the kernels of other RS-AuNPs such as Au25(SR)18, Au38(SR)24, Au102(SR)44, Au130(SR)50, and Au133(SR)52 are presented in ESI Fig. S2 and S3.† Interestingly, according to different types of kernel structures, those crystallized RS-AuNPs larger than Au25(SR)18 can be classified into three categories, as shown in Fig. 3: the first category includes Au28(SR)20, Au30S(SR)18, Au36(SR)24, Au40(SR)24, and Au52(SR)32, whose Au kernels possess fcc-like symmetry, are comprised of several interpenetrating cuboctahedra. The Au102(SR)44 and Au130(SR)50 nanoclusters in which the Au kernels possess Ino-decahedral (D5h) symmetry can be grouped into the second category. The last category includes the remaining RS-AuNPs such as Au25(SR)18 and Au133(SR)52 with icosahedral Au kernels, and Au38(SR)24 with a fused biicosahedral kernel.
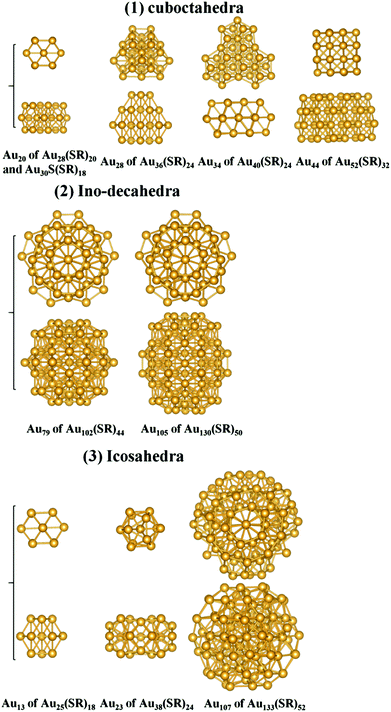 |
| Fig. 3 Two orthogonal views of the Au kernels of the crystallized RS-AuNPs larger than Au25(SR)18, all classified into three categories: (1) cuboctahedra, (2) Ino-decahedra, and (3) icosahedra. | |
Simulated powder X-ray diffraction (XRD) curves of these crystallized RS-AuNPs exhibit different characteristic peaks for nanoclusters with different Au kernel structures. First, we confirm the reliability of the theoretical formula for computing the XRD curves by comparing the simulated XRD curves and the measured XRD curves of Au25(SR)18 and Au38(SR)24, as shown in ESI Fig. S4.† Next, the XRD spectra of the RS-AuNPs in all three categories are presented in Fig. 4. From Fig. 4(a), one can see that the Au28(SR)20, Au30S(SR)18, Au36(SR)24, Au40(SR)24, and Au52(SR)32 nanoclusters exhibit similar diffraction patterns, where a main peak is located at about 4.0 nm−1, and the two weaker peaks are located at 6.5 nm−1 and 7.5 nm−1, respectively. In the second category (Fig. 4(b)), the consistent XRD curves of Au102(SR)44 and Au130(SR)50 nanoclusters, where only one less pronounced peak is located at 7.7 nm−1, are significantly different from those in the first category. For the last category, as shown in Fig. 4(c), the diffraction pattern of the Au133(SR)52 nanocluster, where four weaker peaks are located in the range 5–9 nm−1, differ from those of Au25(SR)18 and Au38(SR)24. The multi-peak diffraction pattern can be attributed to the multi-shell structure of the Au133(SR)52 nanocluster. The analysis above shows that the diffraction pattern is closely related to the structure of the Au kernel in RS-AuNP, which can offer clues to theoretical prediction of the structure of RS-AuNP even when the single crystal is lacking.
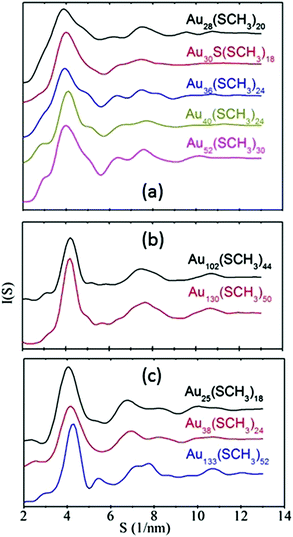 |
| Fig. 4 Theoretical powder X-ray diffraction (XRD) curves of the crystallized RS-AuNPs. | |
Fig. 5 presents the simulated UV/Vis optical absorption spectra of Au40(SR)24 and Au52(SR)32 based on the time-dependent DFT (TD-DFT) computation. In addition, the atomic orbital (AO) component of Kohn–Sham molecular orbitals is displayed. As shown in Fig. 5(a), the extrapolated optical band-edge of Au40(SR)24 is 1.1 eV, consistent with the measured optical gap.39 The extrapolated optical band-edge of Au52(SR)32 is 1.25 eV (Fig. 5(c)). The population analysis of Kohn–Sham (KS) molecular orbitals, as shown in Fig. 5(b) and (d), demonstrates that the occupied frontier molecular orbitals are mainly contributed from the Au(5d), denoted as the d band, while the Au(6sp) atomic orbitals show dominant contributions to the unoccupied orbitals (sp band). The feature absorption peaks can therefore be assigned to the d → sp interband transition.
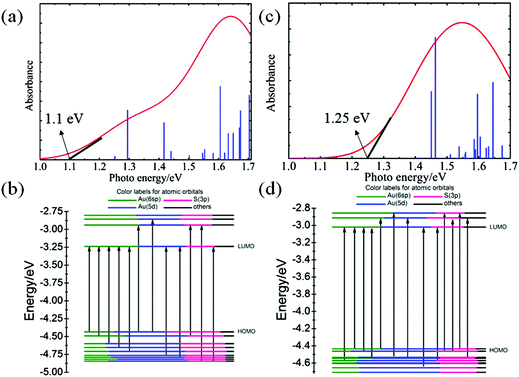 |
| Fig. 5 Simulated UV/Vis absorption spectra and Kohn–Sham orbital energy level diagrams of Au40(SR)24 (a and b) and Au52(SR)32 (c and d). The energies are in eV. Each Kohn–Sham orbital is drawn to show the relative contributions (line length with color labels) of the atomic orbitals of Au(6sp) olive, Au(5d) blue, S(3p) magenta, and other orbital contributions from C and H atoms are in black. | |
Finally, the catalytic properties of Au40(SR)24 and Au52(SR)32 are examined by using CO oxidation as a probe. To this end, a few surface staple motifs on the clusters are removed to allow for the catalytic reaction.49,50 The computed catalytic reaction pathway for CO oxidation on the Au40(SR)24 cluster is shown in Fig. 6(a). CO and O2 are coadsorbed initially with a binding energy of −1.76 eV. Then the O2 molecule moves toward the adsorbed CO molecule to form a bridge-like metastable intermediate state characterized by the O–C–O–O species with the O–O bond length being 1.45 Å, and the CO oxidation requires overcoming a reaction barrier of 0.51 eV (TS1). The O–O bond length is further elongated to 1.81 Å, while CO fully grasps an O atom of O2 to form a CO2 molecule. The CO2 can eventually desorb by overcoming a relatively low-energy barrier of 0.35 eV (TS2), leaving the other O atom adsorbed on the gold cluster.
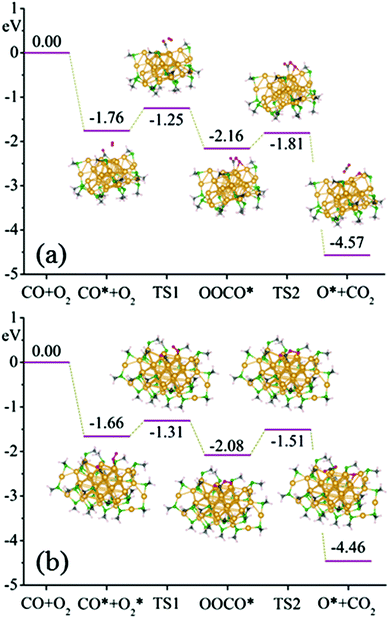 |
| Fig. 6 Computed reaction pathway for the CO oxidation on Au40(SR)24 (a) and Au52(SR)32 (b) clusters. Here, the symbol * denotes adsorption of the corresponding molecules on Au atoms. Au, S, C, O, and H atoms are in gold, olive, dark gray, red, and white, respectively. | |
For CO oxidation on the Au52(SR)32 cluster, as shown in Fig. 6(b), the CO and O2 molecules can be favorably coadsorbed on two neighboring low-coordinated Au atoms, with the coadsorption energy of CO and O2 being about −1.66 eV. The reaction pathway is similar to that for the Au40(SR)24, in which the two molecular species require to overcome reaction barriers of 0.35 eV (TS1) and 0.57 eV (TS2), respectively, to arrive at the final product state. Both reaction barriers are comparable to those of typical nanogold catalysts,51–53 indicating that the Au40(SR)24 and Au52(SR)32 clusters can be a stand-alone nanoscale catalyst for CO oxidation.
Conclusions
We have performed a systematic structure analysis of two medium-sized Au40(SR)24 and Au52(SR)32 nanoclusters. We find that the kernel structures of both nanoclusters can be viewed as several interpenetrating cuboctahedra. Based on this observation, we suggest classification of the crystallized RS-AuNPs into three groups according to their kernel structures. In the first group, Au kernels of RS-AuNPs such as Au28(SR)20, Au30S(SR)18, Au36(SR)24, Au40(SR)24, and Au52(SR)32 exhibit fcc-like symmetry, comprising several interpenetrating cuboctahedra. In the second group, Au kernels of RS-AuNPs such as Au102(SR)44 and Au130(SR)50 possess Ino-decahedral (D5h) symmetry. The third group includes Au25(SR)18, Au133(SR)52 with icosahedral Au kernels, or Au38(SR)24 with a fused icosahedral Au kernel. Clusters in each group give rise to distinct diffraction patterns, thereby providing important clues for the theoretical prediction of the structure of RS-AuNPs as long as the XRD data are known. We have also computed the UV/Vis absorption spectra and Kohn–Sham orbital energy level diagrams for Au40(SR)24 and Au52(SR)32. Our calculation indicates that the first absorption peak can be assigned to the d → sp interband transition. Examination of the catalytic properties of Au40(SR)24 and Au52(SR)32 suggests that both clusters can be stand-alone nanoscale catalysts for CO oxidation.
Acknowledgements
W. W. X. is supported by the China Postdoctoral Science Foundation Project (Y419022011, Y519031011), and the National Natural Science Foundation of China (11504396). Y. G. is supported by the startup funding from the Shanghai Institute of Applied Physics, the Chinese Academy of Sciences (Y290011011), the National Natural Science Foundation of China (21273268, 11574340), the “Hundred People Project” from the Chinese Academy of Sciences, the “Pu-jiang Rencai Project” from the Science and Technology Commission of Shanghai Municipality (13PJ1410400), and the CAS-Shanghai Science Research Center (CAS-SSRC-YJ-2015-01). The computational resources utilized in this research were provided by the Shanghai Supercomputer Center, the National Supercomputing Center in Tianjin and the Supercomputing Center of the Chinese Academy of Sciences in Beijing. X. C. Z. is supported by a grant from the Nebraska Center for Energy Sciences Research and a USTC fund for the 1000-Talents B program (summer research).
References
- M. M. Alvarez, J. T. Khoury, T. G. Schaaff, M. N. Shafigullin, I. Vezmar and R. L. Whetten, J. Phys. Chem. B, 1997, 101, 3706–3712 CrossRef CAS.
- Y. Negishi, K. Nobusada and T. Tsukuda, J. Am. Chem. Soc., 2005, 127, 5261–5270 CrossRef CAS PubMed.
- S. Chen, R. S. Ingram, M. J. Hostetler, J. J. Pietron, R. W. Murray, T. G. Schaaff, J. T. Khoury, M. M. Alvarez and R. L. Whetten, Science, 1998, 280, 2098–2101 CrossRef CAS PubMed.
- A. S. K. Hashmi and G. J. Hutchings, Angew. Chem., Int. Ed., 2006, 45, 7896–7936 CrossRef PubMed.
- H. Häkkinen, Nat. Chem., 2012, 4, 443–455 CrossRef PubMed.
- Y. Pei and X. C. Zeng, Nanoscale, 2012, 4, 4054–4072 RSC.
- H. Qian, M. Zhu, Z. Wu and R. Jin, Acc. Chem. Res., 2012, 45, 1470–1479 CrossRef CAS PubMed.
- R. W. Murray, Chem. Rev., 2008, 108, 2688–2720 CrossRef CAS PubMed.
- R. Jin, Nanoscale, 2010, 2, 343–362 RSC.
- R. Jin, Nanoscale, 2015, 7, 1549–1565 RSC.
- P. Maity, S. Xie, M. Yamauchi and T. Tsukuda, Nanoscale, 2012, 4, 4027–4037 RSC.
- A. Das, C. Liu, H. Y. Byun, K. Nobusada, S. Zhao, N. Rosi and R. Jin, Angew. Chem., Int. Ed., 2015, 54, 3140–3144 CrossRef CAS PubMed.
- C. Zeng, C. Liu, Y. Chen, N. L. Rosi and R. Jin, J. Am. Chem. Soc., 2014, 136, 11922–11925 CrossRef CAS PubMed.
- D. Crasto, G. Barcaro, M. Stener, L. Sementa, A. Fortunelli and A. Dass, J. Am. Chem. Soc., 2014, 136, 14933–14940 CrossRef CAS PubMed.
- M. Zhu, C. M. Aikens, F. J. Hollander, G. C. Schatz and R. Jin, J. Am. Chem. Soc., 2008, 130, 5883–5885 CrossRef CAS PubMed.
- J. Akola, M. Walter, R. L. Whetten, H. Häkkinen and H. Grönbeck, J. Am. Chem. Soc., 2008, 130, 3756–3757 CrossRef CAS PubMed.
- M. W. Heaven, A. Dass, P. S. White, K. M. Holt and R. W. Murray, J. Am. Chem. Soc., 2008, 130, 3754–3755 CrossRef CAS PubMed.
- C. Zeng, T. Li, A. Das, N. L. Rosi and R. Jin, J. Am. Chem. Soc., 2013, 135, 10011–10013 CrossRef CAS PubMed.
- D. Crasto, S. Malola, G. Brosofsky, A. Dass and H. Häkkinen, J. Am. Chem. Soc., 2014, 136, 5000–5005 CrossRef CAS PubMed.
- C. Zeng, H. Qian, T. Li, G. Li, N. L. Rosi, B. Yoon, R. N. Barnett, R. L. Whetten, U. Landman and R. Jin, Angew. Chem., Int. Ed., 2012, 51, 13114–13118 CrossRef CAS PubMed.
- H. Qian, W. T. Eckenhoff, Y. Zhu, T. Pintauer and R. Jin, J. Am. Chem. Soc., 2010, 132, 8280–8281 CrossRef CAS PubMed.
- P. D. Jadzinsky, G. Calero, C. J. Ackerson, D. A. Bushnell and R. D. Kornberg, Science, 2007, 318, 430–433 CrossRef CAS PubMed.
- Y. Chen, C. Zeng, C. Liu, K. Kirschbaum, C. Gayathri, R. R. Gil, N. L. Rosi and R. Jin, J. Am. Chem. Soc., 2015, 137, 10076–10079 CrossRef CAS PubMed.
- A. Dass, S. Theivendran, P. R. Nimmala, C. Kumara, V. R. Jupally, A. Fortunelli, L. Sementa, G. Barcaro, X. Zuo and B. C. Noll, J. Am. Chem. Soc., 2015, 137, 4610–4613 CrossRef CAS PubMed.
- C. Zeng, Y. Chen, K. Kirschbaum, K. Appavoo, M. Y. Sfeir and R. Jin, Sci. Adv., 2015, 1, e1500045 Search PubMed.
- C. Zeng, Y. Chen, G. Li and R. Jin, Chem. Commun., 2014, 50, 55–57 RSC.
- H. Qian and R. Jin, Chem. Commun., 2011, 47, 11462–11464 RSC.
- C. Zeng, Y. Chen, G. Li and R. Jin, Chem. Mater., 2014, 26, 2635–2641 CrossRef CAS.
- P. R. Nimmala, B. Yoon, R. L. Whetten, U. Landman and A. Dass, J. Phys. Chem. A, 2013, 117, 504–517 CrossRef CAS PubMed.
- R. Balasubramanian, R. Guo, A. J. Mills and R. W. Murray, J. Am. Chem. Soc., 2005, 127, 8126 CrossRef CAS PubMed.
- S. Takano, S. Yamazoe, K. Koyasu and T. Tsukuda, J. Am. Chem. Soc., 2015, 137, 7027–7030 CrossRef CAS PubMed.
- G. Li, C. Zeng and R. Jin, J. Am. Chem. Soc., 2014, 136, 3673–3679 CrossRef CAS PubMed.
- P. R. Nimmala and A. Dass, J. Am. Chem. Soc., 2014, 136, 17016–17023 CrossRef CAS PubMed.
- M. Azubel, J. Koivisto, S. Malola, D. Bushnell, G. L. Hura, A. L. Koh, H. Tsunoyama, T. Tsukuda, M. Pettersson, H. Häkkinen and R. D. Kornberg, Science, 2014, 345, 909–912 CrossRef CAS PubMed.
- C. Zeng, Y. Chen, C. Liu, K. Nobusada, N. Rosi and R. Jin, Sci. Adv., 2015, 1, e1500425 Search PubMed.
- H. Qian, Y. Zhu and R. Jin, J. Am. Chem. Soc., 2010, 132, 4583–4585 CrossRef CAS PubMed.
- S. Knoppe, J. Boudon, I. Dolamic, A. Dass and T. Bürgi, Anal. Chem., 2011, 83, 5056–5061 CrossRef CAS PubMed.
- Y. Chen, C. Zeng, D. R. Kauffman and R. Jin, Nano Lett., 2015, 15, 3603–3609 CrossRef CAS PubMed.
- S. Malola, L. Lehtovaara, S. Knoppe, K. J. Hu, R. E. Palmer, T. Burgi and H. Häkkinen, J. Am. Chem. Soc., 2012, 134, 19560–19563 CrossRef CAS PubMed.
- B. Delley, J. Chem. Phys., 1990, 92, 508–517 CrossRef CAS.
- B. Delley, J. Chem. Phys., 2003, 113, 7756–7764 CrossRef . DMoL3 is available from Accelrys.
- J. P. Perdew, K. Burke and M. Ernzerhof, Phys. Rev. Lett., 1996, 77, 3865–3868 CrossRef CAS PubMed.
- W. W. Xu, Y. Gao and X. C. Zeng, Sci. Adv., 2015, 1, e1400211 Search PubMed.
- W. W. Xu and Y. Gao, J. Phys. Chem. C, 2015, 119, 14224–14229 CAS.
- Y. Pei, Y. Gao, N. Shao and X. C. Zeng, J. Am. Chem. Soc., 2009, 131, 13619–13621 CrossRef CAS PubMed.
- Y. Pei, R. Pal, C. Liu, Y. Gao, Z. Zhang and X. C. Zeng, J. Am. Chem. Soc., 2012, 134, 3015–3024 CrossRef CAS PubMed.
- Y. Pei, Y. Gao and X. C. Zeng, J. Am. Chem. Soc., 2008, 130, 7830–7832 CrossRef CAS PubMed.
- Y. Pei, S. S. Lin, J. Su and C. Liu, J. Am. Chem. Soc., 2013, 135, 19060–19063 CrossRef CAS PubMed.
- O. Lopez-Acevedo, K. A. Kacprzak, J. Akola and H. Häkkinen, Nat. Chem., 2010, 2, 329–334 CrossRef CAS PubMed.
- Z. Wu, D. Jiang, A. K. P. Mann, D. R. Mullins, Z. Qiao, L. F. Allard, C. Zeng, R. Jin and S. H. Overbury, J. Am. Chem. Soc., 2014, 136, 6111–6122 CrossRef CAS PubMed.
- H. Li, L. Li, A. Pedersen, Y. Gao, N. Khetrapal, H. Jónsson and X. C. Zeng, Nano Lett., 2015, 15, 682–688 CrossRef CAS PubMed.
- Y. Gao, N. Shao, Y. Pei, Z. Chen and X. C. Zeng, ACS Nano, 2011, 5, 7818–7829 CrossRef CAS PubMed.
- C. Liu, Y. Tan, S. Lin, H. Li, X. Wu, L. Li, Y. Pei and X. C. Zeng, J. Am. Chem. Soc., 2013, 135, 2583–2595 CrossRef CAS PubMed.
Footnote |
† Electronic supplementary information (ESI) available. See DOI: 10.1039/c5nr07810e |
|
This journal is © The Royal Society of Chemistry 2016 |
Click here to see how this site uses Cookies. View our privacy policy here.