DOI:
10.1039/C5NJ02437D
(Paper)
New J. Chem., 2016,
40, 497-502
Waste corn-cob cellulose supported bio-heterogeneous copper nanoparticles for aza-Michael reactions†
Received
(in Nottingham, UK)
11th September 2015
, Accepted 29th October 2015
First published on 4th November 2015
Abstract
Bio-heterogeneous poly(amidoxime) copper nanoparticles were prepared on the modified surface of waste corn-cob cellulose through a graft copolymerization process. The Cu-nanoparticles (0.05 mol% to 50 mol ppm) selectively promoted the aza-Michael reaction of aliphatic amines to give the corresponding alkylated products at room temperature in methanol. The supported nanoparticles were easy to recover and reused eight times without a significant loss of their activity.
1. Introduction
The conjugate reaction of various amines with α,β-unsaturated carbonyl compounds provides β-amino carbonyl ingredients, which have attracted great attention in the synthesis of biological active molecules and some essential intermediates of antibiotics and pharmaceutical products.1–6 The conjugate addition of amines to α,β-unsaturated compounds is known as an atom economic aza-Michael reaction and is very simple to carry out. Generally, aza-Michael addition proceeds in the presence of strong acids or bases and some side products are formed. Thus, chemists have paid more attention to the development of milder catalytic systems. A number of homogeneous aza-Michael reaction catalysts such as AlCl3,7 PtCl4·5H2O,8 InCl3/TMSCl,9 Bi(NO)3,10 samarium iodobinaphtholate,11 boric acid,12 [Ni(PPP)(THF)](ClO4)2,13 Pd(N,N′-ppo)Cl2,14 ionic liquids,15–18 and TMSCl-promoted transition metals19 have been reported. The loss of material and difficulty of purification in each step of the reaction will dramatically reduce the overall efficiency of a synthetic process. Thus, not only the catalytic activity and selectivity but also the stability and reusability for safe, non-toxic, sustainable chemistry and green organic synthesis, have become particular interests in the development of heterogeneous metal catalysts. Therefore, the development of a perfect heterogeneous metal catalyst that meets the reactivity, selectivity, broad applicability and reusability criteria of a perfect catalyst is highly desirable. In this context, a lot of heterogeneous metal catalysts are employed. For instance, MOF-99,20 a polystyrene supported CuI–imidazole complex,21 biomaterial supported organo-catalyst,22 silica sulfuric acid,23 phosphate impregnated titania,24 nanocrystalline copper(II) oxide,25 PANI–Cu,26 PANI–In,27 PSSA,28 Amberlyst-15,29 cellulose–Cu(0),30 CeCl3·7H2O/NaI/Al2O3,31 polystyrene–AlCl3,32 and enzymes33,34 showed good catalytic activity for Michael additions. Although many economic and sustainable protocols have been employed by researchers dedicated to the development of green processes for aza-Michael additions, some of the catalysts have poor or even weak catalytic effects.35–37
Recently, scientists have been searching for cheap and more environmentally friendly sustainable resources and processes. In this context, cellulose, which is widely abundant in nature, would be the most acceptable material. The development of bio-based materials and composites could be a promising solution both in terms of environmental and performance aspects. The structure of biopolymers provides several advantages such as a low density and cost, a bio-renewable character, ubiquitous availability and interesting mechanical properties compared with glass fibres.38 Thus, natural celluloses would be highly desirable, attractive candidates to explore as the solid support for catalysts.39 Cellulose has interesting features, for example, a high sorption capacity, stability, and physical and chemical versatility, making it attractive for use as supports. Cellulose can be chemically modified and effective chelating ligands can be introduced onto the cellulose backbone.40,41 Recently, several biopolymers, for example alginate,42 gelatin,43 starch44 and chitosan45 derivatives, have been utilized as a support for catalytic applications. Thus, the development of a general, simple and convenient method, which can be applied to a number of substrates of different natures in a catalytic process under milder and environmentally friendly conditions, is highly desirable. Recently, we developed a waste corn-cob cellulose supported poly(amidoxime) ligand which successfully absorbed a lot of metal complexes from industrial waste water.46
Herein we report the corn-cob cellulose supported poly(amidoxime) copper nanoparticle (CuN@PA) catalyzed regioselective aza-Michael reaction of aliphatic amines with α,β-unsaturated compounds in methanol at room temperature. CuN@PA showed high efficiency (0.05 mol% to 50 mol ppm) and it was reused several times without a significant loss of catalytic activity.
2. Experimental
2.1 General information
All manipulations were performed under atmospheric conditions unless otherwise noted. Reagents and solvents were obtained from commercial suppliers and used without further purification. Water was deionized with a Millipore system to Milli-Q grade. CuSO4·5H2O was purchased from Aldrich Chemical Industries, Ltd. 1H NMR (500 MHz) and 13C NMR (125 MHz) spectra were measured with a BRUKER-500 spectrometer, central laboratory, University Malaysia Pahang. The 1H NMR chemical shifts were reported relative to tetramethylsilane (TMS, 0.00 ppm). The 13C NMR chemical shifts were reported relative to CDCl3 (77.0 ppm). Inductively coupled plasma atomic emission spectrometry (ICP-AES) was performed on Shimadzu ICPS-8100 equipment by the central laboratory, University Malaysia Pahang. FTIR spectra were measured with a Perkin Elmer (670) spectrometer equipped with an ATR device (ZnSe crystal), FIST, University Malaysia Pahang. XPS spectra were measured with an ESCALA 250 (Thermo Fisher Scientific K.K.) by Hi-Tech Instruments SDN BHD, Puchong, Malaysia. FE-SEM was performed with a JSM-7800F, central laboratory, University Malaysia Pahang. TEM was performed with a HT-7700, Hi-Tech Instruments SDN BHD, Puchong, Malaysia. TLC analysis was performed using Merck silica gel 60 F254. Column chromatography was carried out on silica gel (Wakogel C-200).
2.2 Materials
The waste corn-cob was obtained from Gambang, Pahang, Malaysia. Corn-cobs were cut into small sizes (3 cm long average). The raw corn-cob (100 g) was boiled with 10% NaOH (800 mL) and glacial acetic acid (800 mL) for 4 h and 2 h respectively, and washed with distilled water. The raw corn-cob cellulose was bleached with hydrogen peroxide (500 mL) and 5% NaOH (300 mL), washed with distilled water (500 mL) several times and oven dried at 50 °C before use. Acrylonitrile monomer was purchased from Aldrich and the monomer was passed through columns filled with chromatographic grade activated alumina to remove the inhibitor. Other chemicals such as ceric ammonium nitrate (CAN) (Sigma-Aldrich), methanol (Merck), and other analytical grade reagents were used without purification.
2.3 Graft copolymerization
The graft copolymerization reaction was carried out in a 1 L three-neck round bottom flask equipped with a stirrer and condenser in a thermostatic water bath. The cellulose slurry was prepared by stirring 6 g of corn-cob cellulose in 150 mL of distilled water overnight. N2 gas was purged into the flask to remove oxygen during the grafting process. The slurry was heated to 55 °C and 2.17 mL of diluted sulphuric acid (H2SO4/H2O, 1
:
1) was added to the mixture. After 5 min, 2 g of CAN (in 10 mL solution) was added and the reaction mixture was stirred continuously. After 20 minutes, 14 mL of acrylonitrile monomer was added into the cellulose suspension and stirred for 4 h under a nitrogen atmosphere. The reaction mixture was cooled and the obtained cellulose was washed several times with aqueous methanol (methanol/water, 4
:
1). The product was finally oven dried at 50 °C to obtain a constant weight.47
2.4 Synthesis of poly(amidoxime) ligand 1
Hydroxylamine hydrochloride (NH2OH·HCl, 20 g) was dissolved in 500 mL of aqueous methanol (methanol/water, 4
:
1) and neutralized using NaOH solution and the resulting NaCl precipitate was removed by filtration. The pH of the reaction was adjusted to pH 11 through the addition of NaOH solution. The ratio of methanol and water was maintained at 4
:
1 (v/v). Poly(acrylonitrile) grafted corn-cob cellulose (10 g) was placed into a two-neck round bottom flask equipped with a stirrer, condenser and thermostatic water bath. The hydroxylamine solution was then added to the flask and the reaction was stirred at 70 °C for 4 h. After the completion of the reaction, the chelating ligands were separated from the hydroxylamine solution using filtration followed by washing with aqueous methanol. The obtained cellulose based amidoxime ligand was neutralized with 100 mL of methanolic 0.1 M HCl solution. The ligand was filtered and washed several times with aqueous methanol and dried at 50 °C for 6 h.48,49
2.5 Preparation of the poly(amidoxime)copper complex 2
An aqueous solution of CuSO4·5H2O (126 mg, in 5 mL H2O) was added into a stirred mixture of 1 g of poly(amidoxime) ligand 1 in 25 mL of water at room temperature. The blue CuSO4 immediately turned a green colour and the mixture was stirred for 1 h at room temperature. The reaction mixture was filtered and washed several times with excess amounts of water and MeOH, and dried at 60 °C for 1 h. The ICP-AES analysis showed that 0.5 mmol g−1 of copper49 was coordinated with the poly(amidoxime) ligand.
2.6 Preparation of the poly(amidoxime) Cu-nanoparticles (CuN@PA)
Poly(amidoxime)copper complex 2 (500 mg) was dispersed in 50 mL of deionized water and then hydrazine hydrate (0.7 mL) was added. The resulting dark brown colored CuN@PA material was collected by centrifugation, washed with methanol and acetone, dried under vacuum at 100 °C and stored under a nitrogen atmosphere.
2.7 General procedure for the aza-Michael reaction
In a typical experiment, a mixture of amine (1 mmol), α,β-unsaturated Michael acceptor (1.1–4 mmol), and corn-cob cellulose supported CuN@PA (0.05 mol%) in 2 mL of MeOH was stirred at room temperature for 1 h. The reaction progress was monitored by TLC analysis. After the completion of the reaction, CuN@PA was filtered, washed with MeOH (3 × 5 mL), dried, and reused in the next run under the same reaction conditions. The filtrate was concentrated to give the crude product, which was purified by column chromatography (hexane/ethyl acetate) to give the corresponding Michael addition products.
3. Results and discussion
The waste corn-cob was obtained from Gambang, Pahang, Malaysia.
The waste corn-cobs were cut into small pieces and successively boiled with 10% NaOH and glacial acetic acid for 4 h and 2 h respectively. The resulting cellulose was washed with water and bleached with hydrogen peroxide and 5% NaOH. The obtained white coloured cellulose was graft polymerized with acrylonitrile using ceric ammonium nitrate as a redox initiator to give cellulose supported poly(acrylonitrile) further reacted with hydroxylamine provided the poly(amidoxime) chelating ligand 1 (Scheme 1).
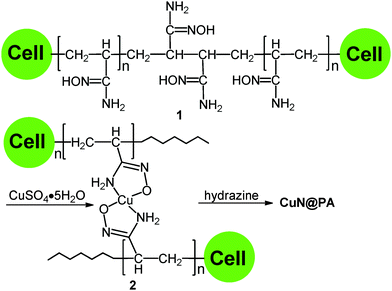 |
| Scheme 1 Synthesis of waste corn-cob cellulose-supported CuN@PA. | |
Fig. 1a–d show photographic images of waste corn-cob, corn-cob cellulose, the poly(amidoxime) copper complex 2, and CuN@PA respectively. The IR spectrum of cellulose shows absorption bands at 3432 cm−1 and 2924 cm−1 for O–H and C–H stretching, respectively (Fig. 1g). The small peak at 1424 cm−1 belongs to the CH2 symmetric stretching.50 The small peak at 1371 cm−1 and broad band at 1162 cm−1 showed C–O stretching. The C–O–C pyranose ring skeletal vibration gives a strong band at 1062 cm−1. A small sharp peak at 892 cm−1 corresponds to the glycosidic C1–H deformation with a ring vibration contribution and OH bending, which is characteristic of the α-glycosidic linkages between glucose in cellulose.46,48 The IR spectrum of the acrylonitrile-grafted cellulose exhibits a new absorption band at 2244 cm−1 attributed to the CN stretching of nitrile.
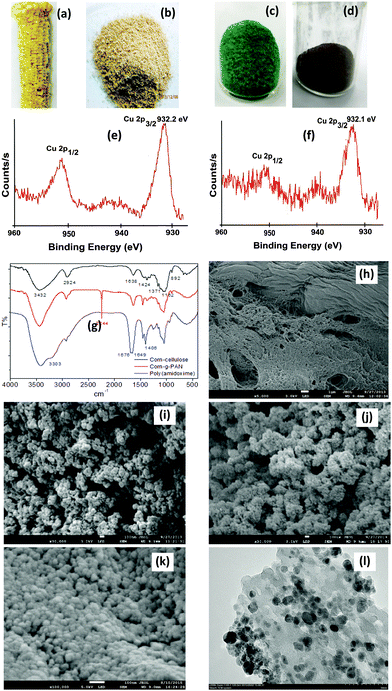 |
| Fig. 1 (a) Photo image of waste corn-cob, (b) photo image of 1, (c) photo image of 2, (d) photo image of CuN@PA, (e) XPS spectrum of CuN@PA, (f) XPS spectrum of used CuN@PA, (g) IR spectrum of cellulose and modified cellulose, (h–k) SEM images of cellulose, cellulose supported poly(acrylonitrile), 1, and copper complex 2 respectively, (l) TEM image of CuN@PA. | |
The poly(amidoxime) chelating ligand showed new absorption bands at 1676 cm−1 and 1649 cm−1 corresponding to the C
N stretching and N–H bending modes, respectively. In addition, a shoulder was created at 3303 cm−1 for the N–H and OH stretching bands and at 1406 cm−1 for bending. Clear evidence of the CN band at 2244 cm−1 disappeared and new absorption bands for the amidoxime group appeared, which confirmed the successful production of poly(amidoxime) functionality on the corn-cob cellulose grafted copolymers. The poly(amidoxime) functional group was then treated with aqueous copper sulphate at room temperature to give blue coloured poly(amidoxime) copper complex 2 (Fig. 1c). The IR spectrum of 2 shows bands at 3303 cm−1 and 1125 cm−1,51 which indicate that the copper salt was coordinated with the poly(amidoxime) ligand 2. The copper complex 2 was then treated with hydrazine hydrate52 to give dark brown coloured copper nanoparticles CuN@PA (∅ = 8.0 ± 7 nm) which were stabilized by the poly(amidoxime) ligands (Fig. 1d and l).
The XPS spectrum shows (Fig. 1e) a single Cu 2p3/2 peak at 932.2 eV, which is assigned to the binding energy of Cu(0).52 The FE-SEM micrograph of the corn-cob cellulose shows an unsmooth morphology including a fine crystalline surface (Fig. 1h). The FE-SEM micrograph of the poly(acrylonitrile) grafted corn-cob cellulose shows a spherical shaped bead structure which is distinguishable from the surface of corn-cob cellulosic fine crystalline structure (Fig. 1h) and is clear evidence that grafting occurred on the cellulosic materials (Fig. 1i). The poly(amidoxime) chelating ligand 1 showed a distinct morphology with a fine bead structure that looks like a small grain like structure (Fig. 1j). The SEM micrograph of the poly(amidoxime) ligand after the adsorption of CuSO4 shows a compact surface (Fig. 1k), which is different to the fine bead structure of 1 (Fig. 1j). The TEM image shows the good dispersion of copper nanoparticles on to the cellulose surface (Fig. 1k).
To check the reactivity of CuN@PA we applied these corn-cob cellulose supported copper-nanoparticles CuN@PA to the conjugate addition of aliphatic amines with different Michael acceptors at room temperature and the results are summarized in Table 1. The cellulose supported copper nanoparticles CuN@PA efficiently (0.05 mol%) promoted aza-Michael reactions in methanol at room temperature. The α,β-unsaturated carbonyl Michael acceptor, methyl acrylate, smoothly promoted the aza-Michael reaction with secondary aliphatic amines such as dibutylamine, piperidine, morpholine and sterically hindered secondary dibenzylamine to give the corresponding products in up to 95% yields (5a–d). Similarly, butyl acrylate efficiently provided the corresponding Michael addition adducts in up to 96% yields (5e–i). The α,β-unsaturated acrylonitrile also promoted the addition reaction to give the mono-alkylated products (5j–n). It should be noted that cyclohexylamine and ethylenediamines also efficiently underwent the aza-Michael reaction to give the poly-alkylated products 5o–q with methyl, butyl acrylate and acrylonitrile Michael acceptors respectively.
Table 1 Aza-Michael addition reaction of aminesa

|
Reactions were carried out using 1 mmol of amine, 1.1 mol equiv. of Michael acceptor, and 0.05 mol% of CuN@PA in 2 mL MeOH at room temperature for 1 h.
|
|
The recycling of the catalyst is an important issue in heterogeneous catalysis systems. We turned our attention to the reuse of our cellulose supported copper nanoparticles CuN@PA and the results are summarized in Table 2. After the completion of the first run, the reaction mixture was filtrated and washed with methanol. The XPS image (Fig. 1f) of reused CuN@PA shows similarity with the fresh CuN@PA (Fig. 1e). The solid brown coloured CuN@PA was dried at 80 °C under vacuum, and was then used in the next run without changing the reaction conditions.
Table 2 Aza-Michael reaction with recycled catalyst CuN@PAa

|
Cycle |
Yielda (%) |
Cycle |
Yielda (%) |
Reaction was carried out using 1 mmol of piperidine, 1.1 mmol of butyl acrylate, 0.05% of CuN@PA in 2 mL of MeOH at room temperature.
|
1 |
96 |
6 |
93 |
2 |
94 |
7 |
92 |
3 |
94 |
8 |
91 |
4 |
93 |
|
|
5 |
94 (<0.11 mol ppm Cu leached out) |
|
|
The CuN@PA could be used eight times without a significant loss of catalytic activity. Only a slight loss of catalytic activity was observed under the same reaction conditions as the initial run. A slight loss of catalytic activity was observed after five cycles due to the loss of CuN@PA during the filtration process. A trace amount of copper species (<0.11 mol ppm of Cu, ICP-AES) leached out from the reaction mixture. Thus, it is reasonable to believe that the cellulose supported copper catalyst can be repeatedly used for large-scale production without a significant loss of its catalytic activity.
The chemoselectivity of CuN@PA was then investigated. A mixture of aniline and piperidine was exposed to acrylonitrile (4 mol equiv.) under the presented reaction conditions (Scheme 2). Interestingly, aniline did not undergo the reaction; only the mono-Michael adduct of piperidine was obtained exclusively. This clearly reflects the chemoselectivity of aliphatic amines versus aromatic amines. This selectivity is due to the lower reactivity of aromatic amines in comparison to aliphatic amines. This selectivity could be useful in discriminating between the two types of amines for synthetic applications.
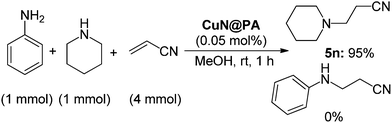 |
| Scheme 2 Chemoselective aza-Michael reaction. | |
An investigation into the highest catalytic activity obtainable for the aza-Michael reaction was also performed (Scheme 3). The Michael addition reaction was carried out using 10 mmol of dibutyl amine and acrylonitrile in the presence of 50 mol ppm (0.1 mg) of CuN@PA which still efficiently promoted the aza-Michael reaction to provide 5j in 94% yield. The TON and the turnover frequency (TOF) of the catalyst reached 18
412 and 7364 h−1, respectively; these are, as far as we know, the highest TON and TOF obtained for a cellulose supported CuN@PA catalysed aza-Michael reaction.
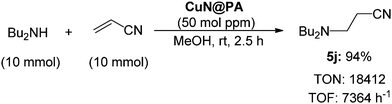 |
| Scheme 3 Lower catalytic loading (50 ppm) in the aza-Michael reaction. | |
4. Conclusion
In summary, waste corn-cob cellulose supported poly(amidoxime) copper-nanoparticles efficiently promoted the chemoselective aza-Michael addition of aliphatic amines with conjugate α,β-unsaturated Michael acceptors. The addition reactions were efficiently (0.05 mol% to 50 mol ppm) promoted to provide the corresponding products with excellent yields. After the completion of the reaction, the supported nanoparticles were separated from the reaction mixture by simple filtration, and they were reused several times without a significant loss of activity.
Acknowledgements
This work is supported by Ministry of Education Malaysia, fund no. RDU 140124. The authors are grateful to Nor Hafizah Bt Zainal Abidin, scientific officer, and central lab, University Malaysia Pahang.
Notes and references
- G. Bartoli, C. Cimarelli, E. Marcantoni, G. Palmieri and M. Petrini, J. Org. Chem., 1994, 59, 5328 CrossRef CAS
.
- G. Cardillo and C. Tomasini, Chem. Soc. Rev., 1996, 25, 117 RSC
.
- A. K. Nezhad, M. N. S. Rad, G. H. Hakimelahi and B. Mokhtari, Tetrahedron, 2002, 58, 10341 CrossRef
.
- S. Fustero, B. Pina, E. Salavert, A. Navarro, M. C. Ramirez de Arellano and A. S. Fuentes, J. Org. Chem., 2002, 67, 4667 CrossRef CAS PubMed
.
- P. Traxler, U. Trinks, E. Buchdunger, H. Mett, T. Meyer, M. Mueller, U. Regenass, J. Rosel and N. Lydon, J. Med. Chem., 1995, 38, 2441 CrossRef CAS PubMed
.
- A. Graul and J. Castaner, Drugs Future, 1997, 22, 956 CrossRef CAS
.
- W. E. Hahn, W. Szalecki and W. Boszczyk, Pol. J. Chem., 1978, 52, 2497 CAS
.
- S. Kobayashi, K. Kakumoto and M. Sugiura, Org. Lett., 2002, 4, 1319 CrossRef CAS PubMed
.
- L. Yang, L. W. Xu and C. G. Xia, Tetrahedron Lett., 2007, 48, 1599 CrossRef CAS
.
- N. Srivastava and B. K. Banik, J. Org. Chem., 2003, 68, 2109 CrossRef CAS PubMed
.
- D. Didier, A. Meddour, S. B. Lafollee and J. Collin, Eur. J. Org. Chem., 2011, 2678 CrossRef CAS
.
- M. K. Chaudhuri, S. Hussain, M. L. Kantam and B. Neelima, Tetrahedron Lett., 2005, 46, 8329 CrossRef CAS
.
- L. Fadini and A. Togni, Chem. Commun., 2003, 30 RSC
.
- G. A. Ardizzoia, S. Brenna and B. Therrien, Dalton Trans., 2012, 41, 783 RSC
.
- S. R. Roy and A. K. Chakraborti, Org. Lett., 2010, 12, 3866 CrossRef CAS PubMed
.
- J. M. Xu, Q. Wu, Q. Y. Zhang, F. Zhang and X.-F. Lin, Eur. J. Org. Chem., 2007, 1798 CrossRef CAS
.
- X. Chen, X. Li, H. Song, Y. Qian and F. Wang, Tetrahedron Lett., 2011, 52, 3588 CrossRef CAS
.
- L.-W. Xu, J.-W. Li, S.-L. Zhou and C.-G. Xia, New J. Chem., 2004, 28, 183 RSC
.
- L.-W. Xu and C.-G. Xia, Synthesis, 2004, 2191 CAS
.
- L. T. L. Nguyen, T. T. Nguyen, K. D. Nguyen and N. T. S. Phan, Appl. Catal., A, 2012, 425, 44 CrossRef
.
- L. Li, Z. Liu, Q. Ling and X. Xing, J. Mol. Catal. A: Chem., 2012, 353, 178 CrossRef
.
- S. Verma, S. L. Jain and B. Sain, Org. Biomol. Chem., 2011, 9, 2314 CAS
.
- Y. Wang, Y. Q. Yuan and S. R. Guo, Molecules, 2009, 14, 4779 CrossRef CAS PubMed
.
- J. Nath and M. K. Chaudhuri, Catal. Lett., 2009, 133, 388 CrossRef CAS
.
- M. L. Kantam, S. Laha, J. Yadav and S. Jha, Tetrahedron Lett., 2009, 50, 4467 CrossRef CAS
.
- M. L. Kantam, M. Roy, S. Roy, B. Sreedhar and R. L. De, Catal. Commun., 2008, 9, 2226 CrossRef CAS
.
- M. L. Kantam, M. Roy, S. Roy, M. S. Subhas, B. Sreedhar, B. M. Choudary and R. L. De, J. Mol. Catal. A: Chem., 2007, 265, 244 CrossRef CAS
.
- V. Polshettiwar and R. S. Varma, Tetrahedron Lett., 2007, 48, 8735 CrossRef CAS
.
- B. Das and N. Chowdhury, J. Mol. Catal. A: Chem., 2007, 263, 212 CrossRef CAS
.
- K. R. Reddy and N. S. Kumar, Synlett, 2006, 2246 CrossRef CAS
.
- G. Bartoli, M. Bartolacci, A. Giuliani, E. Marcantoni, M. Massaccesi and E. Torregiani, J. Org. Chem., 2005, 70, 169 CrossRef CAS PubMed
.
- L. Dai, Y. Zhang, Q. Dou, X. Wang and Y. Chen, Tetrahedron, 2013, 69, 1712 CrossRef CAS
.
- L. N. Monsalve, F. Gillanders and A. Baldessari, Eur. J. Org. Chem., 2012, 1164 CrossRef CAS
.
- K. P. Dhake, P. J. Tambade, R. S. Singhal and B. M. Bhanage, Tetrahedron Lett., 2010, 51, 4455 CrossRef CAS
.
- J. Christoffers, Eur. J. Org. Chem., 1998, 1259 CrossRef CAS
.
- J. Clariana, N. Galvez, C. Marchi, M. M. Manas, A. Vallribera and E. Molins, Tetrahedron, 1999, 55, 7331 CrossRef CAS
.
- N. Giuseppone, V. P. Weghe, M. Mellah and J. Collin, Tetrahedron, 1998, 54, 13129 CrossRef CAS
.
- A. J. Bolton, Mat. Tech., 1994, 9, 12 Search PubMed
.
- E. Guibal, Prog. Polym. Sci., 2005, 30, 71 CrossRef CAS
.
- D. W. O'Connell, C. Birkinshaw and T. F. O'Dwyer, Bioresour. Technol., 2008, 99, 6709 CrossRef PubMed
.
- W. Dahou, D. Ghemati, A. Oudia and D. Aliouche, Biochem. Eng. J., 2010, 48, 187 CrossRef CAS
.
- W. L. Wei, H. Y. Zhu, C. L. Zhao, M. Y. Huang and Y.-Y. Jiang, React. Funct. Polym., 2004, 59, 33 CrossRef CAS
.
- X. Zhang, Y. Geng, B. Han, M. Y. Ying, M. Y. Huang and Y. Y. Jiang, Polym. Adv. Technol., 2001, 12, 642 CrossRef CAS
.
- K. Huang, L. Xue, Y. C. Hu, M. Y. Huang and Y.-Y. Jiang, React. Funct. Polym., 2002, 50, 199 CrossRef CAS
.
- F. Quignard, A. Choplin and A. Domard, Langmuir, 2000, 16, 9106 CrossRef CAS
.
- M. L. Rahman, N. R. N. Mustapa and M. M. Yusoff, J. Appl. Polym. Sci., 2014, 131, 40833 Search PubMed
.
- M. L. Rahman, S. Silong, W. M. Z. W. Yunus, M. Z. A. Rahman, M. Ahmad and J. Haron, Eur. Polym. J., 2000, 36, 2105 CrossRef
.
- W. S. S. Yong, M. L. Rahman, S. E. Arshad, N. L. Surugau and B. Musta, J. Appl. Polym. Sci., 2012, 124, 4443 CrossRef
.
- M. L. Rahman, S. Silong, W. M. Z. W. Yunus, M. Z. A. Rahman, M. Ahmad and J. Haron, J. Appl. Polym. Sci., 2001, 79, 1256 CrossRef
.
- F. L. Chuan, L. R. Jun, X. Feng, J. L. Jina, X. S. Jin and C. S. Run, J. Agric. Food Chem., 2006, 54, 5742 CrossRef PubMed
.
- E. Prenesti and S. Berto, J. Inorg. Biochem., 2002, 88, 37 CrossRef CAS PubMed
.
- Z. Huang, F. Li, B. Chen, F. Xue, G. Chen and G. Yuan, Appl. Catal., A, 2011, 403, 104 CrossRef CAS
.
Footnote |
† Electronic supplementary information (ESI) available: NMR data of the aza-Michael products are available. See DOI: 10.1039/c5nj02437d |
|
This journal is © The Royal Society of Chemistry and the Centre National de la Recherche Scientifique 2016 |