DOI:
10.1039/C5MD00376H
(Review Article)
Med. Chem. Commun., 2016,
7, 69-85
Targeting the trehalose utilization pathways of Mycobacterium tuberculosis
Received
1st September 2015
, Accepted 4th November 2015
First published on 16th November 2015
Abstract
Tuberculosis (TB) is an epidemic disease and the growing burden of multidrug-resistant (MDR) TB world wide underlines the need to discover new drugs to treat the disease. Mycobacterium tuberculosis (Mtb) is the etiological agent of most cases of TB. Mtb is difficult to treat, in part, due to the presence of a sturdy hydrophobic barrier that prevents penetration of drugs through the cell wall. Mtb can also survive in a non-replicative state for long periods of time avoiding the action of common antibiotics. Trehalose is an essential metabolite in mycobacteria since it plays key roles in cell wall synthesis, transport of cell wall glycolipids, and energy storage. It is also known for its stress protective roles such as: protection from desiccation, freezing, starvation and osmotic stress in bacteria. In this review we discuss the drug discovery efforts against enzymes involved in the trehalose utilization pathways (TUPs) and their likelihood of becoming drug targets.
1. Introduction
TB is a dreadful disease caused by the Mycobacterium tuberculosis (Mtb) complex.1 It has been estimated that TB has been around for 4000 years by examining prehistoric human skeletal remains.2 Despite its ancient presence, it was not until the 17th century that the symptoms of TB were classified and finally in the 18th century Dr. Robert Koch announced the discovery of Mtb and J. L. Schonlein named this infection as tuberculosis (TB). Today, the burden of TB is approximately 9.0 million active disease cases with about half a million of these cases being caused by multidrug resistant strains (MDR-TB).1 The yearly death toll is around 1.5 million and the number of reported MDR-TB has tripled from 2009 to 2013.1 These statistics emphasize the importance of discovering new drug targets to treat Mtb that, when inhibited, will lead to rapid Mtb killing.1 The current treatment for TB takes at least 6–8 months. There are five different classes of drugs used to treat TB and the treatment regime is highly complex. The common first line drugs are isoniazid (H/Inh), rifampicin/rifampin (R/Rif), pyrazinamide (Z/Pza), ethambutol (E/Emb), and rifapentine (P/Rpt) or rifabutin (Rfb). The current standard drug treatment involves two months of four drugs (Inh, Rif, Pza and Emb) in an intensive phase and four months of Inh and Rif in the continuation phase.3
After disease classification, several attempts were made to cure TB. In the 19th century the β-lactam antibiotics (e.g., penicillin, sulfonamide) grabbed attention due to their broad spectrum bactericidal activity. However, β-lactam antibiotics turned out to be ineffective against Mtb.4 This is due to presence of penicillin-binding proteins which inactivate β-lactam antibiotics and the presence of a complex cell wall and hydrophobic layer.5–8 There are currently several molecular targets being exploited to treat Mtb, the prominent targets include: i) the cell wall and hydrophobic layer (H/Inh, E/Emb, thioamides, and cycloserine), ii) RNA synthesis (R/Rif), and iii) DNA synthesis (aminosalicylate sodium, and fluoroquinolones). Some of the most effective approaches for targeting Mtb, and bacteria in general, have been to target the bacterial cell wall.8–10 For example, the first line TB antibiotics H/Inh, E/Emb target enzymes required for biosynthesis of cell wall building blocks and assembly of the cell wall or hydrophobic layer. We have chosen to focus on the trehalose utilization pathways (TUPs) since they contain several genetically validated targets and also play essential roles in cell wall and glycolipid synthesis.
This review briefly describes the structure of the Mtb cell wall and the TUPs. We describe the key enzymes and their potential as drug targets. Discussion of selected inhibitor–protein complexes and the strategies used to developed inhibitors are also described.
1.1
Mycobacterium tuberculosis cell wall
The Mtb cell wall remains a central focus of TB research. The structure and pathways of the cell wall synthesis have been examined for potential targets to treat TB in a short time span. The cell wall of Mtb is a bastion (Fig. 1). It is composed of a plasma membrane, a peptidoglycan layer, a layer of extractable, non-covalently linked glycans with mycolyl-arabinogalactan (mAG), glycolipids and an outer capsule.11–13
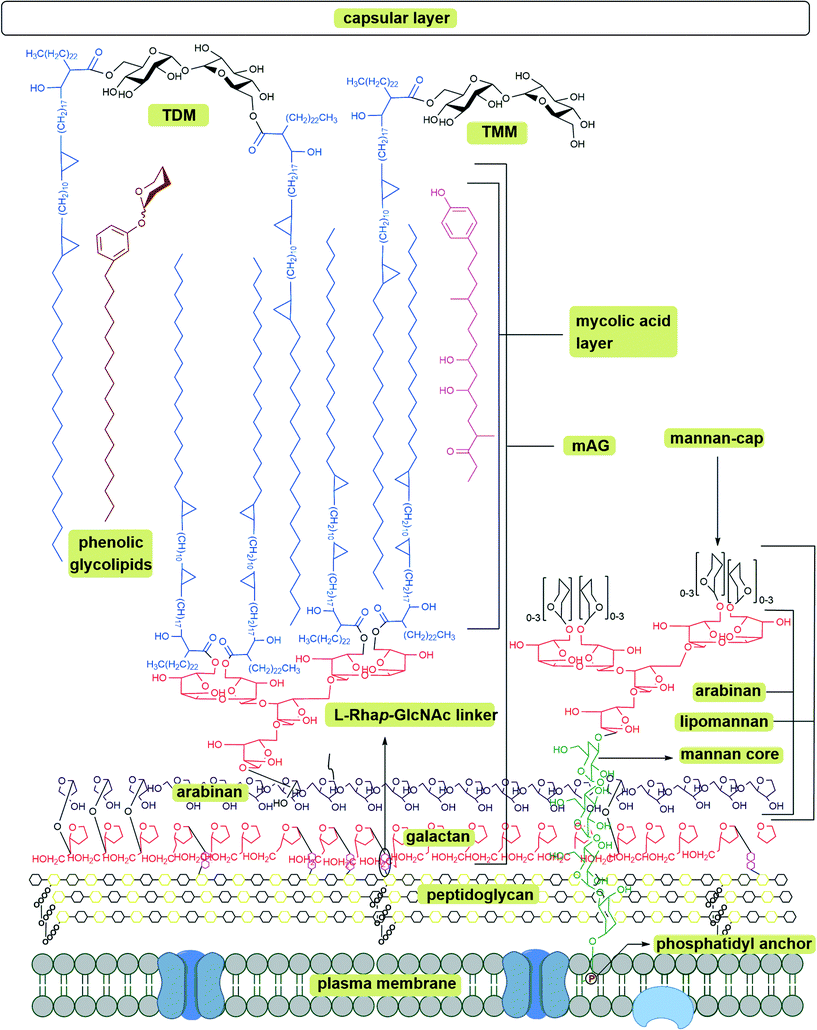 |
| Fig. 1 Schematic representation of Mycobacterium tuberculosis cell wall; mAG = mycolyl-arabinogalactan, AG = arabinogalactan, TMM = trehalose monomycolate, TDM = trehalose dimycolate.8–10 | |
The plasma membrane, which is covered with a peptidoglycan layer, is a selectively permeable membrane that separates the contents of the cell from the outer environment. The repeating units of N-acetyl-β-D-glucosaminyl (NAG)-(1 → 4)-N-glycolylmuramic acid (NAM) forms a polymeric chain, in which NAM components are cross-linked by L-alanyl-D-isoglutaminyl-meso-diaminopymelyl-D-alanine tetrapeptide to form the peptidoglycan layer (represented as repeating units of black and yellow hexagons in Fig. 1). The mAG is composed of covalently bonded units of mycolic acids (MAs), arabinan and galactan. Galactan is a linear polymer of β-D-galactofuranose units linked alternatively through C-5 or C-6. The non-reducing end of the galactan is linked to peptidoglycan via a phosphoryl-N-acetylglycosaminosyl-rhamnosyl (L-Rhap-(1 → 3)-D-GlcNAc (1 → P)) linker.14 The galactan is attached to the reducing end of arabinan through the C-5 of a β-D-galactofuranose moiety. The arabinan polymer is made of α-D-arabinofuranosyl (Araf) moieties connected to each other through α (1 → 5), α (1 → 3), and α (1 → 2) linkages. A branched hexa-arabinofuranosyl moiety is present at the nonreducing end of the arabinan. Two thirds of the terminal units are esterified with MAs.15,16
Products formed by esterification of MAs play a key role in Mtb virulence.17 The major MAs in Mtb are α-, methoxy-, and keto- MAs which are α-alkyl β-hydroxy fatty acids with carbon lengths varying from C60 to C90.18 Among these, α-MAs make up 70% of MAs.19 Along with the MAs attached to AG, Mtb has several extractable glycolipids. Trehalose monomycolate (TMM), trehalose dimycolate (TDM, Cord Factor), phenolic glycolipids (PGL), phosphatidylinositol mannosides (PIMs), phosphatidylethanolamine (PE), triacylglycerols (TAGs), phospholipids, and lipoarabinomannan (LAM) are all non-covalently associated with the lipid layer.8–10 Among all the lipids TDM (Cord Factor) is the most abundant lipid released by virulent Mtb.6 In host macrophage cells C-type lectin mincle receptor recognizes TDM.20 TDM possesses two sets of activities. On the Mtb cell, it is non-toxic and protects the cell from macrophages. Whereas when TDM is released into the host cell it becomes antigenic and highly toxic. TDM possesses unique immunostimulatory activities; for example, it activates macrophages to produce inflammatory cytokines and nitric oxide. It can also induce granulomagenesis and can act as an adjuvant for cell-meditated and humoral immune responses.7,20 The products formed from mycolic acid and other extractable lipids form the hydrophobic layer (mycomembrane) as shown in Fig. 1.
2. Trehalose utilization pathways (TUPs)
Trehalose (α-D-glucopyranosyl-(1 → 1)-α-D-glucopyranoside) is an essential sugar-based metabolite observed in Mtb. It is also a major structural constituent of cell wall glycolipids and acts as a carrier for MAs during cell wall synthesis (Scheme 1).21,22 Trehalose has many roles in Mtb physiology. It is used as carbon and energy source, protects Mtb against desiccation, freezing, osmotic stress, and plays a key role in Mtb virulence.23 Trehalose is found in bacteria, yeast, fungi, plants, and invertebrates; however, it is absent in mammalian cells.24 The absence of trehalose from mammalian cells and its pleiotropic role in mycobacteria make the TUPs attractive antibiotic targets. The TUPs (Scheme 1) include: i) the OtsA/B or TPS-TPPS pathway (shown in blue), ii) the GlgE pathway (shown in plum), iii) the TreY/Z pathway (shown in red), and iv) the pathway where trehalose acts as a mycolyl carrier (shown in black).
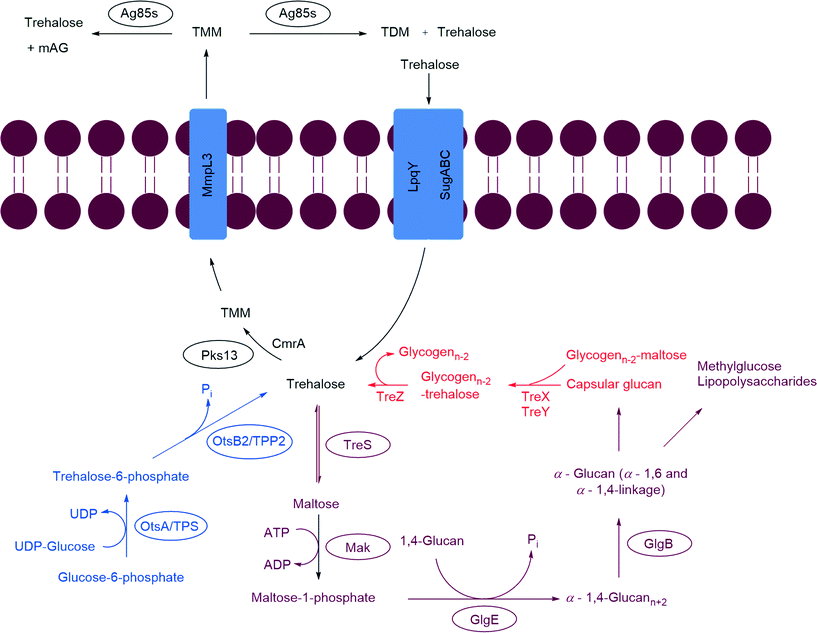 |
| Scheme 1 Trehalose utilization pathway (TUP) in Mycobacterium tuberculosis. Antigen85 complex (Ag85s), mycobacterial membrane protein large 3 (MmpL3), polyketide synthase (Pks13), trehalose phosphate synthase (TPS), trehalose-6-phosphate phosphatase (TPP2), trehalose synthase (TreS), maltokinase (Mak), LpqY-SugA-SugB-SugC (LpqY-SugABC). | |
2.1 Biosynthesis of trehalose
Trehalose is produced via three biosynthetic pathways (Scheme 1).25,26 The major pathway (OtsA/B pathway or TPS-TPP2) involves two steps. The first step is catalyzed by trehalose phosphate synthase (TPS, OtsA), which transfers a glycosyl moiety from UDP-glucose onto glucose-6-phosphate to form trehalose-6-phosphate (T-6-P). The second step is catalyzed by T-6-P phosphatase (TPP, OtsB) were T-6-P undergoes dephosphorylation to generate free trehalose. The second pathway, referred to herein as the GlgE pathway, contains the enzyme trehalose synthase (TreS). TreS can reversibly isomerize maltose to trehalose. The third pathway, TreY/Z, utilizes maltooligosaccharides (glycogen) as a starting material. The first enzyme, maltooligosyltrehalose synthase (TreY), isomerizes the maltosyl moiety at the reducing end of maltooligosaccharide to a trehalose moiety. The second enzyme maltooligosyltrehalose trehalohydrolase (TreZ), hydrolyze the terminal disaccharide to afford free trehalose.
2.2 Utilization of trehalose as substrate for α-glucan synthesis (GlgE pathway)
The GlgE pathway is a four step pathway that contains the enzymes TreS, Mak, GlgE, and GlgB (Scheme 1).27,28 These enzymes act sequentially on trehalose, to produce branched α-glucan with α-1,4 and α-1,6 glycoside linkages. In this pathway trehalose is isomerized by TreS to maltose. The maltose is phosphorylated using ATP by Mak to form maltose-1-phosphate (M1P). The M1P is transferred by the maltosyltransferase GlgE onto the nonreducing end of α-1,4-glucans. The α-1,4-glucan is further modified to afford a branched glucan with α-1,4 and α-1,6 glycosidic bonds. This pathway is interesting due to the fact that α-glucan may be part of capsular α-glucan and methylglucose lipopolysaccharides which play crucial roles in virulence and immune evasion.29–32
2.3 Trehalose as a mycolyl carrier
The thick outer envelope of Mtb is characterized by an array of exotic lipids that are key players in the infection process.17 This envelope is, in part, the consequence of the action of Pks13, mycobacterial membrane protein large 3 (MmpL3), and the Ag85 complex (Ag85s). The enzyme Pks13 catalyses the condensation of key lipids to produce α-alkyl β-ketoacids. The α-alkyl β-ketoacids, with the help of the thiosesterase (TE) domain of Pks13, are transferred onto trehalose. This step is followed by reduction of a keto moiety by CmrA to produce TMM, a MA carrier.33 MmpL3 transports TMM from the cytoplasm to the periplasm.34 The TMM is utilized by the Ag85 complex for biosynthesis of TDM and mAG and releases free trehalose in pseudoperiplasmic space. The TDM and mAG are constituents of Mtb cell wall.35 LpqY-SugA-SugB-SugC (LpqY-SugABC) transports the free trehalose from periplasm back to the cytosol of Mtb.36
2.4 Enzymes that biosynthesize trehalose
2.4.1 Targeting OtsA/B.
OtsA/B is one of the three trehalose biosynthetic pathways shown in Scheme 1. Helen et al. showed that the OtsA/B pathway in Mtb is the dominant pathway.37 The OtsA/B pathway produces trehalose from glucose-6-phosphate. Sassetti et al. performed a transposon site hybridization mutagenesis study and showed that insertions into OtsA and OtsB2 lead to serious growth defects in Mtb, whereas mutants ΔTreS, ΔTreY, ΔOtsA, and ΔOtsB1 showed no apparent growth impairment. This emphasizes the importance of OtsB2 and OtsA.38 Helen et al. studied the essentiality of enzymes involved in three TUPs, by constructing Mtb mutants (ΔTreS, ΔTreY, ΔOtsA, ΔOtsB1, and ΔOtsB2). They showed that OtsB2 is essential for bacterial growth and OtsA deletion will decrease Mtb virulence in mice using these mutants strains.37 Similar studies on other organisms which are closely related to Mtb showed surprisingly different results. In Corynebacterium glutamicum TreY/Z is the predominant trehalose synthesis pathway,39 In Mycobacterium smegmatis the three pathways are functionally redundant,40 and in Mycobacterium leprae, TreY and TreS are pseudogenes, leaving OtsA/B as the only pathway for trehalose biosynthesis.41 The OtsA/B pathway involves condensation of glucose-6-phosphate with uridine diphosphate-glucose forming T-6-P catalyzed by TPS. The T-6-P is utilized by TPP2 to produce trehalose by releasing inorganic phosphate (Pi).14 Two separate open reading frames (ORFs) in the Mtb genome encode for the two homologs of TPP, Rv2006 for TPP1 and Rv3372 for TPP2. Vineetha et al. purified the two enzymes encoded by Rv2006 and Rv3372 then demonstrated that TPP2 shows trehalose phosphate phosphatase activity; whereas, TPP1 activity is unknown. TPP2 is relatively specific for T-6-P (Kd = 39.52, ITC assay) and is active in the presence of Mg2+.23,37,42
Lina et al. modelled the 3D structure of TPP2 and proposed that it has a hydrolase domain, cap domain and an N-terminal domain. The substrate, T-6-P, and Mg2+ binds between the hydrolase domain and the cap domain.23 The OtsB2 gene encodes for TPP2 which dephosphorylates T-6-P. Inhibiting TPP2 leads to accumulation of T-6-P. In Saccharomyces cerevisiae accumulation of T-6-P is detrimental as it acts as a signal for metabolic control of hexokinase II and regulates the first step of glycolysis.37 These factors make OtsA/B a potential target for drug design.
2.4.2 Targeting trehalose synthase (TreS).
TreS is an α-D-glucosylmutase (EC 5.4.99.16) enzyme belonging to the GH13_3 family (CAZy database).43–45 TreS interconverts α-1,4-maltose and trehalose by catalyzing an intramolecular rearrangement, Scheme 1.46,47 TreS has also gained significant interest for its promise as a low cost means to produce trehalose. Pimelobacter sp. R48 and Pseudomonas putida46 TreS was of interest for antibiotic development in Mtb until the OtsA/B and TreY/Z pathways were discovered.47,48 Initially, in vitro experiments and genetic experiments revealed that all three pathways could operate in mycobacteria and other pathogenic organisms26,40 However, Helen et al. showed that TreS plays a secondary in the role of trehalose biosynthesis in Mtb.37 The significance of TreS is different for different microorganism: in Mtb TreS has a role in prolonged infection; in M. leprae, it is a pseudogene; in C. glutamicum, TreS contributes to trehalose degradation; in E. coli and Salmonella enterica, TreS appears to have no role; whereas in Pseudomonas syringae, both TreY/Z and TreS are essential.49 Maih et al. using a genetic approach showed that TreS is not required for trehalose biosynthesis and that in Mtb flux through TreS flows from trehalose to α-maltose the substrate for Mtb maltokinase (Mak).48
X-ray scattering and analytical ultracentrifugation studies from Roy et al. on Mtb TreS showed that TreS forms a tetramer in solution and, together with Mak, forms a non-covalent hetero-octamer complex which expedites M1P formation.45 TreS has two domains, a catalytic domain with a (β/α)8 fold and a C-terminal β-sandwich domain. The active site contains an Asp nucleophile and a Glu proton donor located at the top (C-terminal) rim of the β-barrel. In addition, an Asp situated at the β7–β8 loop of the catalytic site aids in the intramolecular reaction by restraining the hydrolyzed substrate glucose from diffusing out of the active site.47 However, the reaction is not 100% intramolecular as the hydrolysis of the disaccharide (trehalose or maltose) to glucose is observed as a side reaction.47 The TreS reaction proceeds through a double displacement mechanism (Scheme 2). This was confirmed by trapping the glycosyl-enzyme intermediate with 5-fluoroglycosyl fluoride (5) on the homologous M. smegmatis TreS which shares 83% sequence similarity with Mtb TreS. The trapped glycosyl-enzyme intermediate was subjected to protease digestion followed by LC-MS/MS analysis. This showed that Asp230 is the nucleophile participating in retaining double displacement reaction mechanism.47 Zhang et al. assayed α-glycoside inhibitors 6–9 (Fig. 2) against M. smegmatis TreS.50 The results showed that all inhibitors are competitive inhibitors. This behaviour of inhibition also supports the notion that TreS has a mechanism similar to other α-glycosidases. The proposed mechanism for TreS proceeds as follows (Scheme 2): Asp acts as the initial nucleophile acting on α,α′-1,1′-trehalose (1) forming a sandwich domain-maltosyl-enzyme intermediate (2), Glu participates in this reaction by protonating the leaving glucosyl moiety, which on intramolecular rotation attains suitable pose (3) for glycosylation. The β′-maltosyl-enzyme intermediate formed is attacked by the 4-OH of α-glucose to form α′-1′,4-maltose (4) and Glu acts as general base to deprotonating the 4-OH of incoming glucose.48,50
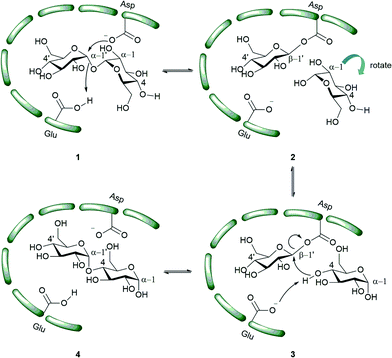 |
| Scheme 2 Proposed mechanism of action of TreS.48 The green bars represent the enzyme active site. | |
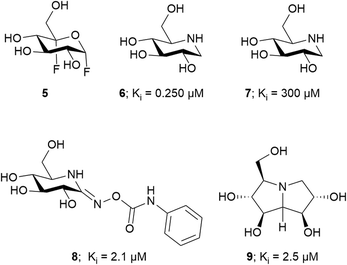 |
| Fig. 2 TreS inhibitors; 5 covalent inhibitor, 6–9 competitive inhibitor. | |
TreS catalyzes the interconversion of α′-1′,4-maltose (4) and α,α′-1,1′-trehalose (1). In trehalose biosynthesis TreS has a minor role in α′-1′,4-maltose (4) to α,α′-1,1′-trehalose interconversion and the equilibrium is favoured towards maltose.37,40,48 α′-1′,4-Maltose is a substrate for Mak and the latter produces M1P. Inhibition of TreS leads to lower levels of α′-1′,4-maltose which is followed by reduced levels of α-glucan. Inhibition of TreS does not inhibit Mtb growth since the organism has redundant pathways for producing glucan and glycogen. These alternate pathways include the classical GlgC–GlgA pathway and the Rv3032 pathway.29,51 These factors make TreS a less interesting target for drug development.
2.4.3 Targeting TreY/Z.
The TreY/Z pathway is one of the three trehalose biosynthesis pathways. It synthesizes trehalose from linear α-glycogen (Scheme 1). TreY isomerises the terminal maltose moiety at the reducing end of α-glycogen to trehalose moiety.52 TreZ cleaves the glycosidic bond between the trehalose moiety and rest of the α-glucan producing free trehalose and glycogenn−2. The genes for TreY (Orf Rv1563c) and TreZ (Orf Rv1562c) are adjacent to each other. Along with these proteins is TreX (Orf Rv1564c) which is a debranching enzyme that cleaves glycosidic bonds with 1–6 linkages on α-glucans. Unbranched α-glucans are used by TreY/Z.25,53,54 Helen et al. produced ΔTreY and showed this deletion has no effect in Mtb growth in vitro or in mice.37 However, the gene coding for TreX is considered essential for Mtb growth. This finding suggests that the recycling of linear α-D-glycogen is necessary for Mtb growth leaving the door open for research in that area.53 The lack of evidence showing the essentiality TreY/Z for Mtb growth and minor role of TreY/Z in trehalose biosynthesis makes TreY/Z lacklustre as a target.
2.5 Utilization of trehalose as substrate for α-glucan synthesis (GlgE pathway)
2.5.1. Targeting maltokinase (Mak).
Mak catalyzes the irreversible, ATP-dependent, phosphorylation of maltose to M1P.55 In Mtb maltokinase activity in the GlgE pathway was previously reported to be performed by Pep2.28,55 Mak shows a Km = 2.52 ± 0.40 mM for maltose and a Km = 0.74 mM for ATP in M. bovis. Mak is dependent on Mg+2 for its activity.56 In 1967 Narumi et al. used dilute ethanol cell extractions to isolate M1P from Mycobacterium bovis strain BCG. Mak (EC 2.7.1.175) was first identified in Actinoplanes missouriensis and was found to be responsible for M1P production in that organism. The sequence alignment of the mak1 gene with putative maltokinases of other organisms, like Pep2 from Streptomyces coelicolor or Rv0127 of Mtb etc., showed that the N-terminal position of the maltokinase of these organisms showed high sequence homologies with TreS.57,58 Roy et al. used crystallography and analytical ultracentrifugation studies to showed that TreS and Mak form a hetero-octamer complex.45 Mak, via ATP-driven M1P synthesis, is responsible for the metabolite flux through the GlgE pathway.27,59
X-ray studies of free and non-hydrolyzable ATP-bound forms of Mak in M. vanbaalenii which shares 59% identity with Mtb, showed that Mak has a bilobal eukaryotic protein kinase-like fold. It has a novel N-terminal domain which is conserved in bifunctional TreS-Mak proteins and is unique to maltokinases.55 The Mak protein exhibits an extended concave shaped structure with two lobes, N-terminal lobe and C-terminal lobe with a central narrow acidic channel (9 Å wide and 30 Å long).28,55 In turn, the N-terminal lobe is subdivided into Cap subdomain and an intermediate subdomain. Maltose binds in the shallow cavity in the C-lobe and is in proximity to the conserved motifs essential for catalysis.28 The N-terminal and C-terminal domains are also highly cross-linked by a significant number of salt bridges. The nucleotide binds with two conformations within the deep pocket between the intermediate subdomain and the C-terminal domain.28,55
Mak may be a potential drug target since the Mak gene has been reported to be essential. However, Mak also synthesizes toxic M1P which needs to be converted to glucan quickly by GlgE since accumulation of M1P leads to cell death.
2.5.2 Targeting GlgE.
GlgE is a genetically validated TB drug target.60 It is a key enzyme in the α-glucan biosynthesis (GlgE pathway, Scheme 1). GlgE (EC 2.4.99.16) is an α-maltose 1-phosphate: (1 → 4)-α-D-glucan 4-α-D-maltosyltransferase belongs to the GH13_3 subfamily (CAZy database).43 GlgE (Rv1327c) was identified and the gene was proven as essential for Mtb growth using transposon site hybridization.38 GlgE transfers M1P (16) units onto the nonreducing end of an α-glucan to produce linear α-1,4-glucan. Kalscheuer et al. used traditional and chemical reverse genetics to show that inactivation of GlgE causes rapid Mtb cell death. The cell death is not due to its inability to produce the important metabolite instead, it is due to the hyper-accumulation of the donor substrate, M1P.27 The increased level of M1P concentration triggers a stress response. This led to over-expression of biosynthetic enzymes required for trehalose and M1P production. This disturbs respiration and leads to formation of reactive oxygen species (ROS) which cause DNA damage and Mtb cell death.61 The absence of a GlgE homologue enzyme in humans and gut flora bacteria and its presence in pathogenic mycobacteria, Pseudomonas, and Burkholderia species makes GlgE an interesting target to potentially treat TB in a short time span.
In order to attain better knowledge on the catalytic activity of GlgE, crystallization of GlgE and its homologous enzymes has been performed. Most notable is the study by Syson et al. who crystallized Streptomyces coelicolar (Sco) GlgE isoform I (GlgEI), a closely related homologue of Mtb GlgE. They also reported that GlgE utilizes M1P and that GlgEI has five domains.59 The α-retaining double displacement mechanism of GlgEI was proven by trapping the β-2-deoxy-2-fluoromaltosyl-enzyme intermediate using 2-deoxy-2-fluoro-α-maltosyl fluroride (20).62 The M1P binding sites of Mtb GlgE and Sco GlgEI differ only at the site represented by Mtb GlgE S303 and Sco GlgEI V279. Thanna et al. prepared a 2-deoxy-2,2-difluoro-α-maltosyl fluroride (21) which was crystalized to probe the active site of an Sco GlgEI-V279S mutant.3 The mutant has the same active site topology of Mtb GlgE and is readily crystalized making it tractable tool enzyme for structure based drug design. Recently, the crystal structures of Mtb GlgE was reported with maltose as ternary complex, maltohexaose as binary complex and also the crystal structures of Sco GlgEI-V279S with inhibitors α-maltose-C-phosphate (MCP, 17) and aza sugar 18.63 The crystal structure of maltohexaose showed a dominant site for α-glucan binding. The crystal structures show Mtb GlgE with five domains A, B, C, N and S. Domain A (insert I and II) and domain B define the overall active site where M1P binds. Domains A, N and S form an extended dimer interface between two GlgE subunits. Domain C and Domain S, the helix bundle, may help in the maltosyl-acceptor substrate binding.59Sco GlgEI and Mtb GlgE have a similar homodimer structure; however, they differ slightly in their monomer orientation. Mtb GlgE has a 23-fold lower affinity towards its acceptor substrate than Sco GlgEI.62,64 The α-retaining double displacement mechanism of GlgE is shown in Scheme 3. Asp418 acts as an initial nucleophile and attacks M1P to form a β-maltosyl enzyme intermediate 12. Glu423 donates a proton to the scissile oxygen atom. Then, Glu423 acts as a general base and assists proton abstraction from the 4-OH group on the non-reducing end of α-1,4-glucan. The 4-OH group attacks the β-maltosyl enzyme intermediate 15, extending the α-1,4-glucan chain by a maltosyl unit. The reaction proceeds through transition states 11 and 14.59,62
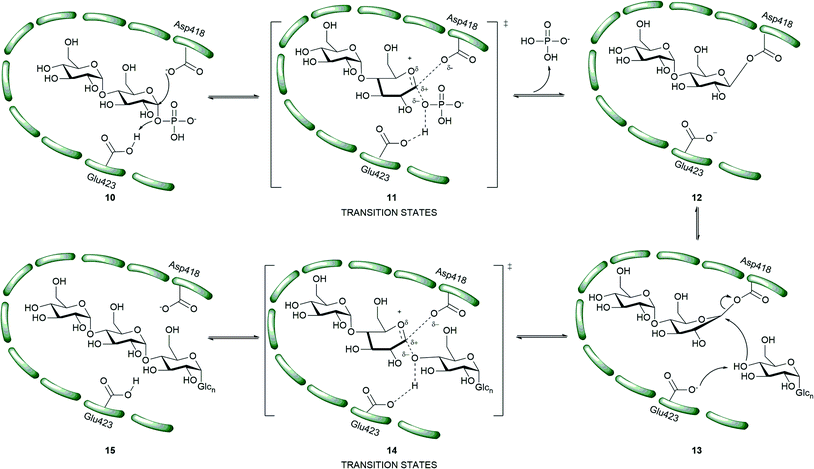 |
| Scheme 3 Mechanism of Mtb GlgE.55 | |
The first molecules to show inhibition of GlgE have been recently prepared and are mimics of maltose. Veleti et al. reported the first inhibitor, MCP (17); a non-hydrolyzable and isosteric substrate analog of M1P. MCP inhibits Mtb GlgE with an IC50 = 230 ± 24 μM.65 A second inhibitor from the same group is a poly-hydroxypyrrolidine-based inhibitor (18), a transition state mimic. The interaction of compound 18 with the GlgE active site is illustrated as 19. Aza sugar 18 inhibited Mtb GlgE with Ki = 237 ± 27 μM and Sco GlgE with a Ki = 102 ± 7 μM.64 Latter, Karl et al. used 2-deoxy-2-fluoro-α-maltosyl fluoride (20) as a covalent inhibitor to trap the Sco E423A mutant enzyme–intermediate complex.62 A similar molecule 2-deoxy-2,2-difluoro-α-maltoslyl fluoride (21), showed hydrogen bonding between the anomeric fluorine and E432 in GlgEI-V279S providing additional evidence for the role of E423 as a proton donor (Scheme 2).3 All the inhibitors reported to date (Fig. 3) have >100 μM, Ki and IC50 value, leaving much room to identify molecules with improved potency. These foundational studies are expected to lead to new compounds that will be used to determine the drugability of GlgE.
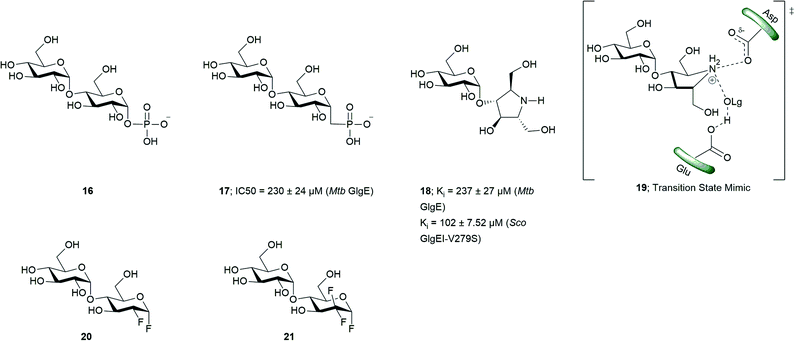 |
| Fig. 3 GlgE non-hydrolyzable and isosteric substrate analogs, transition state mimics and covalent inhibitors. | |
2.6 Enzymes involved in using trehalose as mycolyl carrier
2.6.1 Targeting Pks13.
Portevin et al. showed that Pks13 polyketide synthase is essential for the viability of mycobacteria using gene deletion studies in M. smegmatis.66,67 The MAs in Mtb are synthesized by the (FAS)-polyketide synthase (Pks) pathway, discussed in detail elsewhere.68 In this pathway the key last step, condensation of meromycoloyl-AMP (23) and 2-carboxyacyl-CoA, is catalyzed by the enzyme Pks13. Pks13 is encoded by the fadD32-Pks13-accD4 gene cluster and belongs to the type-1 polyketide synthase gene family.69 Long-chain fatty acyl-AMP ligase (FAAL32) is essential for synthesis and transferring of meromycolyl-AMP.70 Lea-Smith et al. proved that CmrA is essential and that it catalyzes the reduction reaction of β-ketoester of MAs. This was verified by performing a MALDI-TOF mass spectroscopy comparison study on a ΔCmrA deletion mutant and wild type of C. glutamicum and identifying that the ΔCmrA mutant glycolipid contained unreduced β-ketoester (MA). GC-MS analysis of sodium borodeuteride-reduced MAs confirmed the result.71 Pks13 catalyzes the condensation reaction between meromycoloyl-AMP (23) and 2-carboxyacyl-CoA (26) to produce α-alkyl β-ketoacids 29.72 Gavalda et al. recently reported that Pks13 would not release hydrophobic α-alkyl β-ketoacids 29 into bacterium instead, it transfers to trehalose via the Pks13 thiosesterase domain to produce the keto form of TMM (31, TMMk), which when reduced by CmrA to afford TMM (32).33
Bergeret et al. obtained high resolution crystals of apo, palmitoylated, and carboxypalmitoylated forms of 52-kDa Pks13 and confirmed that Pks13 has five domains: i) N-acyl carrier protein (N-ACP), ii) ketosynthase (KS) domain, iii) acyltransferase (AT), iv) C-acyl carrier protein (N-ACP), and v) thiosesterase (TE).73 The proposed mechanism for synthesis of TMM is as follows in Scheme 4.33,67,72 Pks13 has two ACP domains. The ACP located at the N-terminus and C-terminus are called N-ACP and C-ACP, respectively. Each ACP has a phosphopantetheinyl (P-pant) arm at Ser-55 of N-ACP and Ser-1266 of C-ACP, respectively. The meromycoloyl-AMP (23) produced by FAAL32 is transferred to P-pant arm of the N-ACP domain which is further transferred on to the KS domain to produce 25. Simultaneously, the carboxyacyl-CoA is transferred onto the AT domain to form 27, which further transfers onto the C-ACP domain to produce 28. The meromycoloyl on the KS domain 25 and carboxyacyl on the C-ACP domain 28 undergo a condensation reaction to produce α-alkyl β-ketoacids 29 linked to C-ACP arm through a thioester bond. The TE domain cleaves the thioester bond of 29 and forms a new bond at Ser-1533; forming 30. The TE domain with acyltransferase activity transfers compound 30 on to trehalose to produce the keto form of trehalose monomycolate (31, TMMk).33,72–74 CmrA reduces keto group on TMMk to hydroxyl to produce TMM.
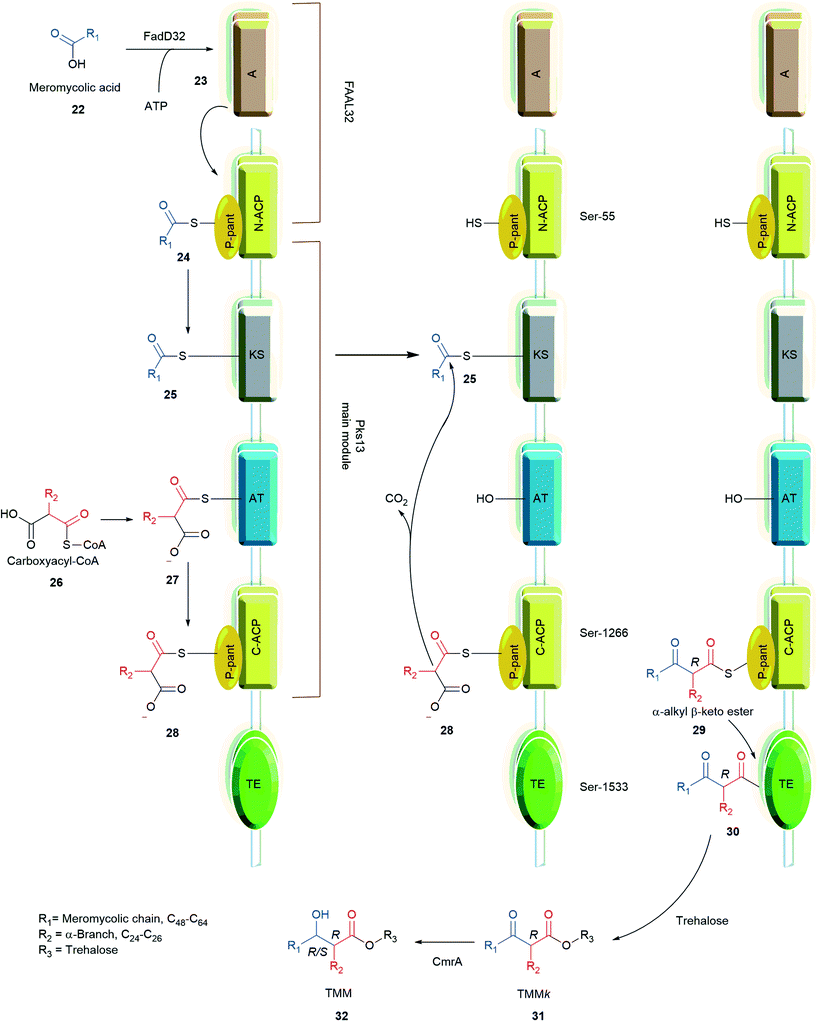 |
| Scheme 4 Mechanism of the Pks13 condensation reaction.33,72 | |
Wilson et al. screened a publicly available a 1113 compound library which was previously identified to be active against Mtb by whole-cell based assays.66,75,76 By performing a growth inhibition assay on the compound library they identified thiophene-based compounds 33–35, Fig. 4 most active with MICs as low as 0.5 μM for 33. When tested against a Pks13 F79S resistant mutant the identified inhibitors showed very low or total loss of potency and overexpression of wild-type Pks13 showed thiophene-based compound resistance which confirms Pks13 as the molecular target. By computational docking studies the inhibitor binding site is anticipated to be in N-ACP domain of Pks13.66 The inhibitors on co-administering with H/INH showed sterilizing activity in growth inhibition studies.66 Pks13, with its prominent role in MAs synthesis is essential for Mtb virulence. The discovery of high potency inhibitors that kill Mtb suggest Pks13 is a drugable target to develop Mtb therapeutic agents.
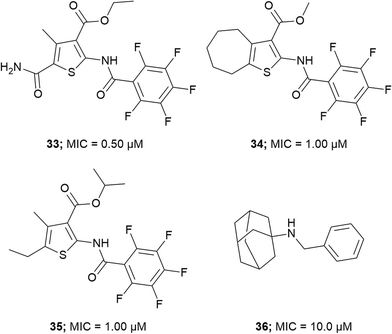 |
| Fig. 4 Pks13 thiophene-based and adamantyl amine based inhibitors. | |
2.6.2 Targeting MmpL3.
Gene knockout and growth inhibition studies have shown that MmpL3 is essential for Mtb viability.77,78 MmpL3 transports MA in the form of TMM across the Mtb plasma membrane to the periplasm.79,80 MmpL3 also imports heme groups.81 MmpL belongs to the resistance-nodulation-cell division (RND) superfamily.77 MmpL proteins are classified into two phylogenetic clusters with three soluble domains (D1, D2 and D3). Cluster II contains MmpL3 and MmpL11.82 The crystal structure of Mtb MmpL11-D2 was solved and has homology with the periplasmic porter subdomain of RND transporters, and it shares 13% amino acid sequence with MmpL3-D2.82 The compound BM212 (1-{[1,5-bis(4-chlorophenyl)-2-methyl-1H-pyrrol-3-yl]methyl}-4-methylpiperazine) a pyrrole derivative 37 was the first inhibitor reported against MmpL3, Fig. 5.83 BM212 was identified by whole cell screening. In order to identify the molecular target for BM212 mutant strains of M. smegmatis, M. bovis BCG and Mtb H37Rv, resistant to BM212 were developed. Whole-genome sequencing of these mutant strains showed mutation in the mmpL3 gene (L215S) indicating MmpL3 as the target.83 Similarly, the inhibitor AU1235 (38), an adamantyl-urea based compound, was identified by cell-based screening. Genome sequencing of AU1235 resistant mutant showed mutation in G253F of mmpL3 gene.78 Further, overexpression of MmpL3 showed an increase in resistance towards AU1235 supporting MmpL3 as drug traget.78 Analogs for BM212 and adamantyl-urea were developed with improved pharmacokinetic properties.84,85 The related compound SQ109 (N′-(2-adamantyl)-N-[(2E)-3,7-dimethylocta-2,6-dienyl]ethane-1,2-diamine, 39) is an inhibitor that completed Phase IIa trials and was originally prepared as analog of Emb.86 Tahlan et al. later found the mode of action of SQ109 to be different for Emb.79 In their studies, Mtb cells treated with SQ109 showed cell shortening, cell widening, a decrease in TDM, and an increase in TMM concentration. The drop in TDM and increase in TMM concentration is not due to inhibition of Ag85s, as in vitro assays on purified Ag85s showed no inhibition.79 The genome sequencing of SQ109 resistant mutants generated using SQ109 analogs showed mutation in the mmpL3 gene, suggesting MmpL3 as target for SQ109.79,80 Other notable inhibitors identified using similar genetic mutation studies include: N-(2,4-dichlorobenzyl)-1-propyl-1H-benzo[d]imidazole-amine (C215, 40),86 tetrahydropyrazolo([1,5-a]pyrimidine-3-carboxamide (THPP, 41),87N-benzyl-6′-7′-dihydrospiro[piperidine-4,4′-thienol[3,2-c]pyran] (SPIROS, 42),87 and 2418 (43) an indolecarboxamide.88–91 Compounds 37, 38, and 41 show broad spectrum antibacterial and antifungal activity, even against pathogens devoid of mycolic acids.90 Recent studies have established that SQ109 and related compounds lead to collapse of the proton motive force. SQ109 inhibits enzymes involved in menaquinone synthesis resulting in inhibition of ATP synthesis.92 The inhibitory effect of compounds 37, 38, 39, 41, and 43 on MmpL3 is due to their ability to dissipate the transmembrane electrochemical proton gradient, the energy source used by MmpL3 to transport lipids.34
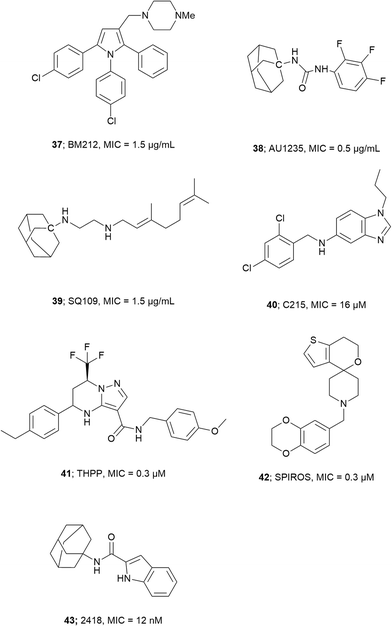 |
| Fig. 5 Important inhibitors of MmpL3. | |
The ability of some the discussed compounds to target multiple enzymes also diminishes the probability of developing resistant mutants towards the respective inhibitors.34,93 MmpL3 is a high priority target in the fight against TB.81
2.6.3 Targeting the Antigen 85 complex.
The Antigen 85 complex (Ag85s) is considered to be a possible target to develop anti-tubercular drugs against. Ag85s constitute a family of three abundantly secreted proteins Ag85A, Ag85B, and Ag85C, which are encoded by fbpA, fbpB and fbpC respectively.94 The three enzymes possess mycolyltransferase activity and share 68–80% identity in the amino acid sequence.35,95 Individual knockout studies on the genes fbpA, fbpB and fbpC showed that the fbpC deletion mutant has 40% less mycolate moieties on the cell wall; whereas, deletion of mutants from fbpA and fbpB resulted in significant decrease in TDM.95,96 The decrease in mAG and TDM will decrease Mtb virulence and drug resistance. This makes the Ag85s complex a novel drug target.95–97 The three Mtb Ag85 isoforms have preferred substrates.57 Studies from Jackson et al. showed that Ag85C participates in transferring a mycoloyl residue onto arabinogalactan, forming mAG. Individual studies by Nguyen et al. and Backus et al. on M. smegmatis and Mtb showed that Ag85A more efficiently mycolates TMM to form TDM.97,98 TMM is an MA donor for Ag85s and all three Ag85s share highly similar active topology.99 Sanki et al. showed that Ag85C can regioselectively transfer acyl groups from model acyl donors to a variety of substrates resembling the terminus of the arabinogalactan.100
The X-ray crystal structure of each homolog of Mtb Ag85s has been determined.99,101,102 These structural studies show that Ag85s have an α/β-hydrolase polypeptide fold, and have catalytic triads formed by Ser124, Glu228, and His260 (Ag85C numbering). The charge on the tetrahedral intermediate in the reaction is stabilized by an oxyanion hole formed by residues, Leu40 and Met125.99 The ping-pong mechanism of Ag85s (Scheme 5) goes as follows: Ser124 from the catalytic triad acts as a nucleophile, which attacks on the carbonyl of TMM. This forms the covalent intermediate 45 which is further hydrolyzed by the hydroxyl of TMM, trehalose, AG, or H2O to form TDM, TMM, mAG, or free MAs, respectively. An activity assay of an Ag85 S124A mutant showed no TDM formation proving the importance of residue for catalysis.103 X-ray crystallographic studies on covalently modified Ag85C with ebselen by Favrot et al. showed that Ebselen binds to C209 of active site which disrupts the hydrogen bond network within the active site which is essential for enzyme activity.103
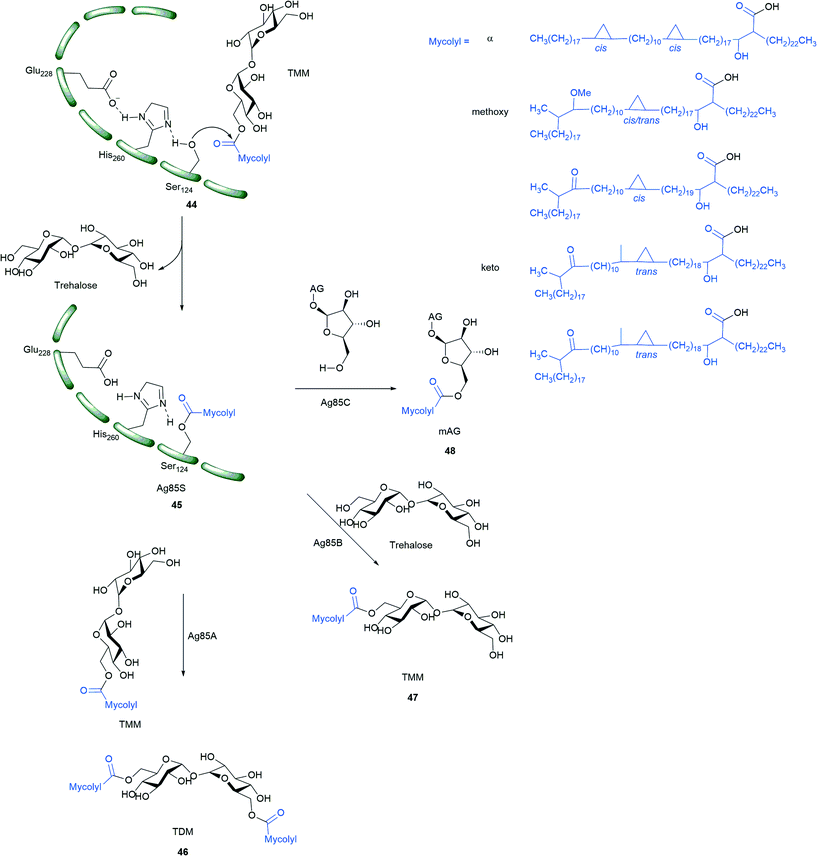 |
| Scheme 5 Mechanism of Ag85s (Ag85A, Ag85B, Ag85C). TMM is the MA donor for the three reactions catalyzed by Ag85s; mAG = mycolyl-arabinogalactan, AG = arabinogalactan, TMM = trehalose monomycolate, TDM = trehalose dimycolate; compound in blue represent alpha, methoxy and keto MAs.8,9 | |
Trehalose and AG analogs.
The search for Ag85s inhibitors began with Belisle et al. in 1997, Fig. 6. Belisle using antagonist 6-azido-6-deoxy-α,α′-trehalose (50) inhibited Ag85C by 60% and showed that Ag85s are essential and possible targets for antimycobacterial drug discovery.35 Compounds 51–57 are trehalose analogs (Fig. 6) and inhibit Ag 85 s. Rose et al. assayed 53–57 against Mtb.104 Wang et al. assayed 51–52 against M. smegmatis.105 Sanki et al. synthesized 5-S-alkyl-5-thio-D-arabinofuranoside AG analogs related to glycoside 58 and assayed them against M. smegmatis.106 Sanki et al. also synthesized a library of arabinose and trehalose-based inhibitors 60–62 and assayed growth inhibition against M. smegmatis ATCC 14468, a non-pathogenic surrogate of Mtb. Compound 60 showed weak inhibition against Ag85C with an IC50 = 25 mM; however, no growth inhibition was observed.107 Warrier et al. identified compound 66 using a growth inhibition assay, the compound showed a MIC of 100 μM.108 Latter, Ibrahim et al. synthesized thiophenyl-thioarabinofuranosides and assayed them against Ag85C using a methylumbelliferyl buryrate fluorescence assay. The most potent thiophenyl-thioarabinofuranoside, compound 67, showed a Ki = 18.2 μM and was co-crystalized with Ag85C and the X-ray complex solved which showed the compound bound in the enzyme active site.109
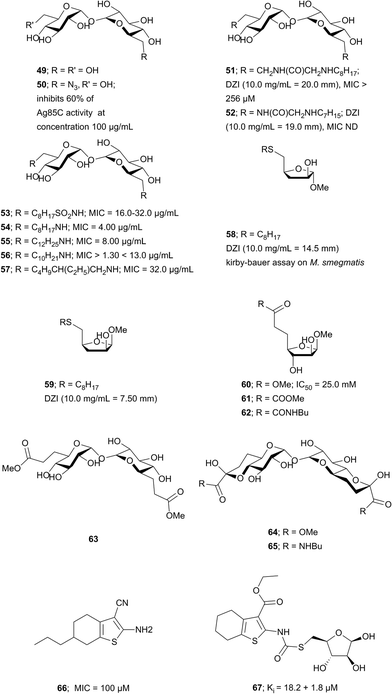 |
| Fig. 6 Ag85C inhibitors structurally related to trehalose and arabinogalactan. | |
Transition state analogs.
Transition state analogs of the tetrahedral intermediate formed during Ag85 catalysis are shown in Fig. 7. Gobec et al. synthesized phosphate based TTSA compounds 68–73 and screened against Mtb Ag85C using an assay developed by Belisle et al.110 Kovac et al. prepared sulfonate 74 similar to Gobec et al. and obtained an IC50 = 4.3 μM.111 The assays used for evaluating inhibition of above trehalose and AG analogs and transition state analogs are discussed below. In summary, there is still a need to develop more potent and selective inhibitors of Ag85s.
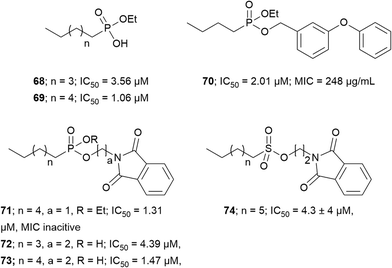 |
| Fig. 7 Inhibitors mimicking the tetrahedral transition state of Ag85s. | |
Assay development.
Elamin et al. developed a colorimetric assay which uses trehalose as a substrate and quantifies the glucose by catalytic activity of Antigen 85A.112 Boucau et al. developed an assay with a Z′ value of 0.81 ± 0.06. In that assay, a p-nitrophenyl-β-D-glucoside with an octanoyl fatty acid was used as the substrate. Ag85s transfer the fatty acid onto a substrate, leaving p-nitrophenyl-β-D-glucoside which is further cleaved by β-glucosidase to release p-nitrophenyl chromophore. Barry et al. performed a label-free assay using electrospray ionization mass spectroscopy (ESI-MS). This assay uses compounds 76 and 49 as substrates in presence of Ag85 to produce 75. The assay uses the concentration of substrate 76 to monitor the reaction progress. A decrease in 76 concentration leads to decrease in the total ion count. Barry et al. confirmed that three enzymes in Ag85s have three different preferred substrates using this assay.113
Covalent inhibitors.
Barry et al. designed compound 77 as a covalent inhibitor to trap an enzyme–inhibitor complex (Fig. 8). The proposed mechanism is that Ser124 attacks the fluorine on 77 by nucleophilic substitution to obtain a covalent enzyme intermediate.113 Favrot et al. identified ebselen (78) by high-throughput screening. Ebselen inhibits Ag85C by covalently binding to Cys209 near the active site and disturbs the hydrogen bond. By subjecting the enzyme–inhibitor complex (Ag85C-EBS) to tryptic digestion and a MALDI study, the ebselen was proven to be covalently bound to Cys209 by a selenenylsulfide bond.103 Favrot et al. also solved an X-ray crystal structures of Ag85C with covalent, allosteric inhibitors: i) Ebselen (78), ii) p-chloromercuribenzoic acid (79), iii) iodoacetamide (80), and iv) Ag85 cysteine to glycine (G209C) mutant. All four showed that covalent modification of C209 lead to enzyme inactivation. Similarly, modification at catalytic triad results in inactivation of Ag85C.114
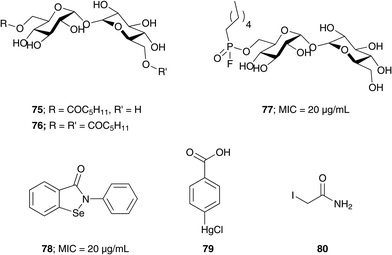 |
| Fig. 8 Covalent inhibitors of the Ag85 complex. | |
The Ag85 complex is believed to be a good target for development of drugs due to its key role in cell wall synthesis. By gene deletion experiments it has been shown that mutants with single fbp genes deleted lose viability up to 40%.96,115 Deleting all the genes produces a non-viable organism.31,115 Since Ag85s share a high degree of similarity in their active site topology, it is expected that a single molecule could inhibit all three homologs. The essentiality of Ag85s for Mtb survival make them good potential targets.
2.3.4 Targeting LpqY-SugA-SugB-SugC.
LpqY-SugABC transports free trehalose from the periplasmic space to cytosol of Mtb.36 In LpqY-SugABC permease: LpqY acts as solute binding protein, SugA and SugB are transmembrane proteins, and SugC is an ATP-hydrolyzing protein. The genes coding for the LpqY-SugABC transporter are organized as an operon in Mtb genome (Rv1235-Rv1238).116 Sassetti et al. by gene mutation studies in mice model showed that the four genes Lpqy-SugA-SugB-SugC are important for Mtb growth and Rengarajan et al. by transposon mutation studies in macrophages showed that LpqY-SugABC are critical for Mtb growth.117,118 LpqY-SugABC is specific for trehalose, and it plays a key role in Mtb virulence by harvesting the trehalose from extracellular sources as well as recycles the trehalose released from Ag85 catalyzed MA transfer.
Based on the fact that Lpqy-SugABC transports extracellular trehalose into the cytosol of Mtb, Swarts et al. developed fluorescent probe to study live Mtb cells, in which azide-modified trehalose (TreAz) analogs were incorporated into the Mtb mycolyl membrane through the TUPs, which on treating with fluorescein-conjugated strained alkyne (biarylazacyclooctynone, BARAC) produces fluorescein labeled Mtb.119 The fluorescein labeled Mtb can be used for real-time study of glycolipid distribution, trafficking, dynamics and changes in Mtb physiology on exposing to inhibitors.119
LpqY-SugABC serve as interesting drug target as inhibition of LpqY-SugABC leads to accumulation of by-product trehalose produced in Ag85s catalyzed mycolyl transfer reaction in periplasm, disrupting the trehalose utilization cycle.36 This eventually leads to the carbon starvation in Mtb followed by cell death.
Conclusions
This review provides an overview of work related to enzymes of the TUPs. A great deal of progress has been made understanding these enzyme pathways; however, drugs which can treat TB in a rapid time span are still lacking. By combining medicinal chemistry, structural biology, structure-based drug design, and novel synthetic methodologies, potent new drugs with minimal side effects may be realized. The TUPs contain a number of potential drug targets such as OtsA/B, GlgE, Pks13, MmpL3 and the Ag85 complex. Inhibiting one or more of these enzymes may lead to novel drugs which can cure active, latent, and MDR-TB.
Acknowledgements
We thank the Professor Donald R. Ronning (D. D. R.) for helpful discussion. The presented work from our group was supported in part by a DeArce Memorial Fund grant to S. J. S. from The University of Toledo and a grant from the National Institutes of Health (grant number AI105084) to S. J. S and D. R. R.
References
-
W. H. Organization, The Global tuberculosis Report, 2014, 2014, France Search PubMed.
- W. Thierry, H. Falk, A.-B. Caroline, W. Florian, K. Tanja, K. Kristin, S. Dick van, R.-G. Sabine, L. Camille, B. Sylvain, M. Axel, S. Philip and N. Stefan, PLoS Pathog., 2008, 4, 1–10 Search PubMed.
- S. Thanna, J. J. Lindenberger, V. V. Gaitonde, D. R. Ronning and S. J. Sucheck, Org. Biomol. Chem., 2015, 13, 7542–7550 CAS.
- S. B. Erdemli, R. Gupta, W. R. Bishai, G. Lamichhane, L. M. Amzel and M. A. Bianchet, Structure, 2012, 20, 2103–2115 CrossRef CAS PubMed.
- H. F. Chambers, D. Moreau, D. Yajko, C. Miick, C. Wagner, C. Hackbarth, S. Kocagöz, E. Rosenberg, W. K. Hadley and H. Nikaido, Antimicrob. Agents Chemother., 1995, 39, 2620–2624 CrossRef CAS PubMed.
- R. L. Hunter, N. Venkataprasad and M. R. Olsen, Tuberculosis, 2006, 86, 349–356 CrossRef CAS PubMed.
- R. L. Hunter, M. R. Olsen and C. Jagannath, Ann. Clin. Lab. Sci., 2006, 36, 371–386 CAS.
- L. Favrot and D. R. Ronning, Expert Rev. Anti-Infect. Ther., 2012, 10, 1023–1036 CrossRef CAS PubMed.
- E. U. Francis, A. K. Sanki, J. Boucau, D. R. Ronning and S. J. Sucheck, Med. Res. Rev., 2010, 30, 290–326 Search PubMed.
-
V. V. Gaitonde and S. J. Sucheck, in Carbohydrate Chemistry: State of the Art and Challenges for Drug Development, ed. C. Laura, Imperial College press, 2015, ch. 18, pp. 439–476 Search PubMed.
- M. Sani, E. N. Houben, J. Geurtsen, J. Pierson, K. de Punder, M. van Zon, B. Wever, S. R. Piersma, C. R. Jiménez, M. Daffé, B. J. Appelmelk, W. Bitter, N. van der Wel and P. J. Peters, PLoS Pathog., 2010, 6, 1–10 Search PubMed.
- P. J. Brennan and D. C. Crick, Curr. Top. Med. Chem., 2007, 7, 475–488 CrossRef CAS PubMed.
- B. A. Dmitriev, S. Ehlers, E. T. Rietschel and P. J. Brennan, Int. J. Med. Microbiol., 2000, 290, 251–258 CrossRef CAS PubMed.
-
M. Ullrich, Bacterial polysaccharides: current innovations and future trends, Horizon Scientific Press, 2009 Search PubMed.
- V. Jarlier and H. Nikaido, FEMS Microbiol. Lett., 1994, 123, 11–18 CrossRef CAS PubMed.
- L. G. Dover, A. M. Cerdeño-Tárraga, M. J. Pallen, J. Parkhill and G. S. Besra, FEMS Microbiol. Rev., 2004, 28, 225–250 CrossRef CAS PubMed.
- M. Jackson, G. Stadthagen and B. Gicquel, Tuberculosis, 2007, 87, 78–86 CrossRef CAS PubMed.
- S. Rafał, K. Konrad, J.-D. Beata and D. Magdalena, Diagn. Microbiol. Infect. Dis., 2013, 76, 298–305 CrossRef PubMed.
- E. Schroeder, O. D. Souza, D. Santos, J. Blanchard and L. Basso, Curr. Pharm. Biotechnol., 2002, 3, 197225 Search PubMed.
- I. Eri, I. Tetsuaki, S. M. Yasu, T. Kenji, Y. Hisakata, T. Osamu, K. Taroh, A. Shizuo, Y. Yasunobu and Y. Sho, J. Exp. Med., 2009, 206, 2879–2888 CrossRef PubMed.
- M. Daffe and P. Draper, Adv. Microb. Physiol., 1997, 39, 131–203 CrossRef.
- R. Ryll, Y. Kumazawa and Y. Ikuya, Microbiol. Immunol., 2001, 45, 801–811 CrossRef CAS PubMed.
- L. Shi, H. Zhang, Y. Qiu, Q. Wang, X. Wu, H. Wang, X. Zhang and D. Lin, Acta Biochim. Biophys. Sin., 2013, 45, 837–844 CrossRef CAS PubMed.
- A. Juan Carlos, Arch. Microbiol., 2000, 174, 217–224 CrossRef.
- K. A. De Smet, A. Weston, I. N. Brown, D. B. Young and B. D. Robertson, Microbiology, 2000, 146, 199–208 CrossRef CAS PubMed.
- K. A. L. Smet, A. Weston, I. N. Brown, D. B. Young and B. D. Robertson, Microbiology, 2000, 146, 199–208 CrossRef PubMed.
- R. Kalscheuer, K. Syson, U. Veeraraghavan, B. Weinrick, K. E. Biermann, Z. Liu, J. C. Sacchettini, G. Besra, S. Bornemann and W. R. Jacobs, Nat. Chem. Biol., 2010, 6, 376–384 CrossRef CAS PubMed.
- L. Jun, G. Xiaotao, S. Neil, C. Weimin, D. Yu, X. Xiaoling, L. Xuemei and R. Zihe, Sci. Rep., 2014, 4, 6418 CrossRef PubMed.
- T. Sambou, P. Dinadayala, G. Stadthagen, N. Barilone, Y. Bordat, P. Constant, F. Levillain, O. Neyrolles, B. Gicquel, A. Lemassu, M. Daffé and M. Jackson, Mol. Microbiol., 2008, 70, 762–774 CrossRef CAS PubMed.
- P. Dinadayala, T. Sambou, M. Daffé and A. Lemassu, Glycobiology, 2008, 18, 502–508 CrossRef CAS PubMed.
- M. C. Gagliardi, A. Lemassu, R. Teloni, S. Mariotti, V. Sargentini, M. Pardini, M. Daffé and R. Nisini, Cell. Microbiol., 2007, 9, 2081–2092 CrossRef CAS PubMed.
- D. Kaur, M. E. Guerin, H. Škovierová, P. J. Brennan and M. Jackson, Adv. Appl. Microbiol., 2009, 69, 23–78 CAS.
- S. Gavalda, F. Bardou, F. Laval, C. Bon, W. Malaga, C. Chalut, C. Guilhot, L. Mourey, M. Daffé and A. Quémard, Chem. Biol., 2014, 21, 1660–1669 CrossRef CAS PubMed.
- W. Li, A. Upadhyay, F. L. Fontes, E. J. North, Y. Wang, D. C. Crans, A. E. Grzegorzewicz, V. Jones, S. G. Franzblau, R. E. Lee, D. C. Crick and M. Jackson, Antimicrob. Agents Chemother., 2014, 58, 6413–6423 CrossRef PubMed.
- J. T. Belisle, V. D. Vissa, T. Sievert, K. Takayama, P. J. Brennan and G. S. Besra, Science, 1997, 276, 1420–1422 CrossRef CAS PubMed.
- R. Kalscheuer, B. Weinrick, U. Veeraraghavan, G. S. Besra and W. R. Jacobs, Proc. Natl. Acad. Sci. U. S. A., 2010, 107, 21761–21766 CrossRef CAS PubMed.
- N. M. Helen, R. S. Graham, V. M. Vladimir, S. A. Alexander, H. Richard, S. B. M. Mark, C. D. Paul, B. Y. Douglas and D. R. Brian, J. Biol. Chem., 2005, 280, 14524–14529 CrossRef PubMed.
- C. M. Sassetti, D. H. Boyd and E. J. Rubin, Mol. Microbiol., 2003, 48, 77–84 CrossRef CAS PubMed.
- M. M. Tzvetkov, C. Klopprogge, O. Zeldan and W. Liebl, Microbiology, 2003, 149, 1659–1673 CrossRef CAS PubMed.
- P. J. Woodruff, B. L. Carlson, B. Siridechadilok, M. R. Pratt, R. H. Senaratne, J. D. Mougous, L. W. Riley, S. J. Williams and C. R. Bertozzi, J. Biol. Chem., 2004, 279, 28835–28843 CrossRef CAS PubMed.
- S. T. Cole, K. Eiglmeier, J. Parkhill, K. D. James, N. R. Thomson, P. R. Wheeler, N. Honore, T. Garnier, C. Churcher, D. Harris, K. Mungall, D. Basham, D. Brown, T. Chillingworth, R. Connor, R. M. Davies, K. Devlin, S. Duthoy, T. Feltwell, A. Fraser, N. Hamlin, S. Holroyd, T. Hornsby, K. Jagels, C. Lacroix, J. Maclean, S. Moule, L. Murphy, K. Oliver, M. A. Quail, M. A. Rajandream, K. M. Rutherford, S. Rutter, K. Seeger, S. Simon, M. Simmonds, J. Skelton, R. Squares, S. Squares, K. Stevens, K. Taylor, S. Whitehead, J. R. Woodward and B. G. Barrell, Nature, 2001, 409, 1007–1011 CrossRef CAS PubMed.
- V. K. Edavana, I. Pastuszak, J. D. Carroll, P. Thampi, E. C. Abraham and A. D. Elbein, Arch. Biochem. Biophys., 2004, 246, 250–257 CrossRef PubMed.
- M. R. Stam, E. G. J. Danchin, C. Rancurel, P. M. Coutinho and B. Henrissat, Protein Eng., Des. Sel., 2006, 19, 555–562 CrossRef CAS PubMed.
- B. L. Cantarel, P. M. Coutinho, C. Rancurel, T. Bernard, V. Lombard and B. Henrissat, Nucleic Acids Res., 2009, 37, D233–D238 CrossRef CAS PubMed.
- R. Roy, V. Usha, A. Kermani, D. J. Scott, E. I. Hyde, G. S. Besra, L. J. Alderwick and K. Fütterer, ACS Chem. Biol., 2013, 8, 2245–2255 CrossRef CAS PubMed.
- T. Nishimoto, M. Nakano, T. Nakada, H. Chaen, S. Fukuda, T. Sugimoto, K. Masashi and Y. Tsujisaka, Biosci., Biotechnol., Biochem., 1996, 60, 640–644 CrossRef CAS PubMed.
- Z. Ran, T. P. Yuan, H. Shouming, L. Michael, D. B. Gary, D. E. Alan and G. W. Stephen, J. Biol. Chem., 2011, 286, 35601–35609 CrossRef PubMed.
- F. Maih, H. Koliwer-Brandl, M. Rejzek, R. A. Field, R. Kalscheuer and S. Bornemann, Chem. Biol., 2013, 20, 487–493 CrossRef PubMed.
- H. Tournu, A. Fiori and P. V. Dijck, PLoS Pathog., 2013, 9, e1003447 CAS.
- R. Zhang, Y. T. Pan, S. He, M. Lam, G. D. Brayer, A. D. Elbein and S. G. Withers, J. Biol. Chem., 2011, 286, 35601–35609 CrossRef CAS PubMed.
- G. Chandra, F. C. Keith and S. Bornemann, Microbiology, 2011, 157, 1565–1572 CrossRef CAS PubMed.
- A. D. Elbein, Y. T. Pan, I. Pastuszak and D. Carroll, Glycobiology, 2003, 13, 17R–27R CrossRef CAS PubMed.
- G. M. Seibold and B. J. Eikmanns, Microbiology, 2007, 153, 2212–2220 CrossRef CAS PubMed.
- A. Nobre, S. Alarico, A. Maranha, V. Mendes and N. Empadinhas, Microbiology, 2014, 160, 1547–1570 CrossRef CAS PubMed.
- F. Joana, M. Ana, M. Vitor, P. P. J. Barbosa, E. Nuno and M.-R. Sandra, Sci. Rep., 2015, 5, 8026 CrossRef PubMed.
- V. Mendes, A. Maranha, P. Lamosa, M. S. da Costa and N. Empadinhas, BMC Biochem., 2010, 11, 1–10 CrossRef PubMed.
- M. Jarling, T. Cauvet, M. Grundmeier, K. Kuhnert and H. Pape, J. Basic Microbiol., 2004, 44, 360–373 CrossRef CAS PubMed.
- J. E. Griffin, J. D. Gawronski, M. A. Dejesus, T. R. Ioerger, B. J. Akerley and C. M. Sassetti, PLoS Pathog., 2011, 7, 1–9 Search PubMed.
- S. Karl, E. M. S. Clare, R. Martin, A. F. Shirley, N. Alap, J. B. Celia, A. F. Robert, F. C. Keith, M. L. David and B. Stephen, J. Biol. Chem., 2011, 286, 38298–38310 CrossRef PubMed.
- K. Rainer, S. Karl, V. Usha, W. Brian, E. B. Karolin, L. Zhen, C. S. James, B. Gurdyal, B. Stephen and R. J. William Jr., Nat. Chem. Biol., 2010, 6, 376–384 CrossRef PubMed , Check this.
- R. Kalscheuer and W. R. Jacobs Jr., Drug News Perspect., 2010, 23, 619–624 CrossRef CAS PubMed.
- S. Karl, E. M. S. Clare, M. R. Abdul, S. Gerhard, T. Minhong, T. Anne, I. S. Dmitri, G. W. Stephen, M. L. David and B. Stephen, Biochemistry, 2014, 53, 2494–2504 CrossRef PubMed.
- J. J. Lindenberger, S. K. Veleti, B. N. Wilson, S. J. Sucheck and D. R. Ronning, Sci. Rep., 2015, 5, 12830 CrossRef CAS PubMed.
- S. K. Veleti, J. J. Lindenberger, S. Thanna, D. R. Ronning and S. J. Sucheck, J. Org. Chem., 2014, 79, 9444–9450 CrossRef CAS PubMed.
- S. K. Veleti, J. J. Lindenberger, D. R. Ronning and S. J. Sucheck, Bioorg. Med. Chem., 2014, 22, 1404–1411 CrossRef CAS PubMed.
- R. Wilson, P. Kumar, V. Parashar, C. Vilchèze, R. Veyron-Churlet, J. S. Freundlich, S. W. Barnes, J. R. Walker, M. J. Szymonifka, E. Marchiano, S. Shenai, R. Colangeli, W. R. Jacobs Jr., M. B. Neiditch, L. Kremer and D. Alland, Nat. Chem. Biol., 2013, 9, 499–506 CrossRef CAS PubMed.
- D. Portevin, C. De Sousa-D'Auria, C. Houssin, C. Grimaldi, M. Chami, M. Daffé and C. Guilhot, Proc. Natl. Acad. Sci. U. S. A., 2004, 101, 314–319 CrossRef CAS PubMed.
- H. Marrakchi, M. A. Laneelle and M. Daffe, Chem. Biol., 2014, 21, 67–85 CrossRef CAS PubMed.
- T. Shimakata, M. Iwaki and T. Kusaka, Arch. Biochem. Biophys., 1984, 229, 329–339 CrossRef CAS PubMed.
- O. A. Trivedi, P. Arora, V. Sridharan, R. Tickoo, D. Mohanty and R. S. Gokhale, Nature, 2004, 428, 441–445 CrossRef CAS PubMed.
- D. J. Lea-Smith, J. S. Pyke, D. Tull, M. J. McConville, R. L. Coppel and P. K. Crellin, J. Biol. Chem., 2007, 282, 11000–11008 CrossRef CAS PubMed.
- S. Gavalda, M. Léger, B. van der Rest, A. Stella, F. Bardou, H. Montrozier, C. Chalut, O. Burlet-Schiltz, H. Marrakchi, M. Daffé and A. Quémard, J. Biol. Chem., 2009, 284, 19255–19264 CrossRef CAS PubMed.
- F. Bergeret, S. Gavalda, C. Chalut, W. Malaga, A. Quémard, J.-D. D. Pedelacq, M. Daffé, C. Guilhot, L. Mourey and C. Bon, J. Biol. Chem., 2012, 287, 33675–33690 CrossRef CAS PubMed.
- M. Léger, S. Gavalda, V. Guillet, B. van der Rest, N. Slama, H. Montrozier, L. Mourey, A. Quémard, M. Daffé and H. Marrakchi, Chem. Biol., 2009, 16, 510–519 CrossRef PubMed.
- J. A. Maddry, S. Ananthan, R. C. Goldman, J. V. Hobrath, C. D. Kwong, C. Maddox, L. Rasmussen, R. C. Reynolds, J. A. Secrist, M. I. Sosa, E. L. White and W. Zhang, Tuberculosis, 2009, 89, 354–363 CrossRef CAS PubMed.
- S. Ananthan, E. R. Faaleolea, R. C. Goldman, J. V. Hobrath, C. D. Kwong, B. E. Laughon, J. A. Maddry, A. Mehta, L. Rasmussen, R. C. Reynolds, J. A. Secrist, N. Shindo, D. N. Showe, M. I. Sosa, W. J. Suling and E. L. White, Tuberculosis, 2009, 89, 334–353 CrossRef CAS PubMed.
- P. Domenech, M. B. Reed and C. E. Barry, Infect. Immun., 2005, 73, 3492–3501 CrossRef CAS PubMed.
- E. G. Anna, P. Ha, A. K. B. G. Vijay, S. S. Michael, J. N. Elton, H. Tamara, J. Victoria, G. Veronica, E. M. B. Sarah, K. Jana, C. Sivagami Sundaram, M. Christophe, J. L. Anne, E. L. Richard, R. M. Michael and J. Mary, Nat. Chem. Biol., 2012, 8, 334–341 CrossRef PubMed.
- K. Tahlan, R. Wilson, D. B. Kastrinsky, K. Arora, V. Nair, E. Fischer, S. W. Barnes, J. R. Walker, D. Alland, C. E. Barry and H. I. Boshoff, Antimicrob. Agents Chemother., 2012, 56, 1797–1809 CrossRef CAS PubMed.
- G. V. Rayasam, Expert Opin. Ther. Targets, 2014, 18, 247–256 CrossRef CAS PubMed.
- M. V. Tullius, C. A. Harmston, C. P. Owens, N. Chim, R. P. Morse, L. M. McMath, A. Iniguez, J. M. Kimmey, M. R. Sawaya, J. P. Whitelegge, M. A. Horwitz and C. W. Goulding, Proc. Natl. Acad. Sci. U. S. A., 2011, 108, 5051–5056 CrossRef CAS PubMed.
- C. Nicholas, T. Rodrigo, L. Yuqi, C. Joe, B. Gaëlle, P. W. Julian and W. G. Celia, Chem. Biol., 2015, 22, 1098–1107 CrossRef PubMed.
- L. V. Rosa, G. Poce, J. O. Canseco and S. Buroni, Antimicrob. Agents Chemother., 2012, 56, 324–331 CrossRef PubMed.
- E. J. North, M. S. Scherman, D. F. Bruhn, J. S. Scarborough, M. M. Maddox, V. Jones, A. Grzegorzewicz, L. Yang, T. Hess, C. Morisseau, M. Jackson, M. R. McNeil and R. E. Lee, Bioorg. Med. Chem., 2013, 21, 2587–2599 CrossRef CAS PubMed.
- G. Poce, R. H. Bates, S. Alfonso, M. Cocozza, G. C. Porretta, L. Ballell, J. Rullas, F. Ortega, A. De Logu, E. Agus, V. La Rosa, M. R. Pasca, E. De Rossi, B. Wae, S. G. Franzblau, F. Manetti, M. Botta and M. Biava, PLoS One, 2013, 8, e56980 CAS.
- K. A. Sacksteder, M. Protopopova and C. E. Barry, Future Microbiol., 2012, 7, 823–837 CrossRef CAS PubMed.
- J. T. Subramanyam, D. Giulia, S. Sreevalli, J. Lalit kumar, N. Ashwini, G. Supreeth, S. Gajanan, M. Sreenivasaiah, M. Meenakshi, A. Disha, B. Gayathri, K. Parvinder, B. Deepa, N. Chandan, R. Jitendar, C. N. N. Kumar, S. Radha, B. Francesca, V. Marcello, M. Riccardo, C. H. Ruben, T. C. Stewart, P. Manoranjan, D. M. Shankar, R. Vasanthi, R. G. Sandeep and D. Neela, Bioorg. Med. Chem. Lett., 2015, 25, 3234–3245 CrossRef PubMed.
- R. R. Kondreddi, J. Jiricek, S. P. S. Rao, S. B. Lakshminarayana, L. R. Camacho, R. Rao, M. Herve, P. Bifani, N. L. Ma, K. Kuhen, A. Goh, A. K. Chatterjee, T. Dick, T. T. Diagana, U. H. Manjunatha and P. W. Smith, J. Med. Chem., 2013, 56, 8849–8859 CrossRef CAS PubMed.
- O. K. Onajole, M. Pieroni, S. K. Tipparaju, S. Lun, J. Stec, G. Chen, H. Gunosewoyo, H. Guo, N. C. Ammerman, W. R. Bishai and A. P. Kozikowski, J. Med. Chem., 2013, 56, 4093–4103 CrossRef CAS PubMed.
- S. P. S. Rao, S. B. Lakshminarayana, R. R. Kondreddi, M. Herve, L. R. Camacho, P. Bifani, S. K. Kalapala, J. Jiricek, N. L. Ma, B. H. Tan, S. H. Ng, M. Nanjundappa, S. Ravindran, P. G. Seah, P. Thayalan, S. H. Lim, B. H. Lee, A. Goh, W. S. Barnes, Z. Chen, K. Gagaring, A. K. Chatterjee, K. Pethe, K. Kuhen, J. Walker, G. Feng, S. Babu, L. Zhang, F. Blasco, D. Beer, M. Weaver, V. Dartois, R. Glynne, T. Dick, P. W. Smith, T. T. Diagana and U. H. Manjunatha, Sci. Transl. Med., 2013, 5, 214ra168 CrossRef PubMed.
- S. Lun, H. Guo, O. K. Onajole, M. Pieroni, H. Gunosewoyo, G. Chen, S. K. Tipparaju, N. C. Ammerman, A. P. Kozikowski and W. R. Bishai, Nat. Commun., 2013, 4 Search PubMed.
- K. Li, L. A. Schurig-Briccio, X. Feng, A. Upadhyay, V. Pujari, B. Lechartier, F. L. Fontes, H. Yang, G. Rao, W. Zhu, A. Gulati, J. H. No, G. Cintra, S. Bogue, Y.-L. Liu, K. Molohon, P. Orlean, D. A. Mitchell, L. Freitas-Junior, F. Ren, H. Sun, T. Jiang, Y. Li, R.-T. Guo, S. T. Cole, R. B. Gennis, D. C. Crick and E. Oldfield, J. Med. Chem., 2014, 57, 3126–3139 CrossRef CAS PubMed.
- B. Rebeca, B. Apoorva and A. A. José, Biochem. Pharmacol., 2015, 96, 159–167 CrossRef PubMed.
- G. Harth, B. Y. Lee, J. Wang, D. L. Clemens and M. A. Horwitz, Infect. Immun., 1996, 64, 3038–3047 CAS.
- J. Mary, R. Catherine, L. Marie-Antoinette, G. Christophe, L. W. Christine, E. Danielle, G. Brigitte and D. Mamadou, Mol. Microbiol., 1999, 31, 1573–1587 CrossRef.
- L. Y. Armitige, C. Jagannath, A. R. Wanger and S. J. Norris, Infect. Immun., 2000, 68, 767–778 CrossRef CAS PubMed.
- L. Nguyen, S. Chinnapapagari and C. J. Thompson, J. Bacteriol., 2005, 187, 6603–6611 CrossRef CAS PubMed.
- K. M. Backus, M. A. Dolan, C. S. Barry, M. Joe, P. McPhie, H. I. Boshoff, T. L. Lowary, B. G. Davis and C. E. Barry, J. Biol. Chem., 2014, 289, 25041–25053 CrossRef CAS PubMed.
- D. R. Ronning, T. Klabunde, G. S. Besra, V. D. Vissa, J. T. Belisle and J. C. Sacchettini, Nat. Struct. Biol., 2000, 7, 141–146 CrossRef CAS PubMed.
- A. K. Sanki, J. Boucau, D. R. Ronning and S. J. Sucheck, Glycoconjugate J., 2009, 26, 589–596 CrossRef CAS PubMed.
- D. R. Ronning, V. Vissa, G. S. Besra, J. T. Belisle and J. C. Sacchettini, J. Biol. Chem., 2004, 279, 36771–36777 CrossRef CAS PubMed.
- H. A. Daniel, H. Gunter, A. H. Marcus and E. David, J. Mol. Biol., 2001, 307, 671–681 CrossRef PubMed.
- L. Favrot, A. E. Grzegorzewicz, D. H. Lajiness, R. K. Marvin, J. Boucau, D. Isailovic, M. Jackson and D. R. Ronning, Nat. Commun., 2013, 4, 2748 Search PubMed.
- J. D. Rose, J. A. Maddry, R. N. Comber, W. J. Suling, L. N. Wilson and R. C. Reynolds, Carbohydr. Res., 2002, 337, 105–120 CrossRef CAS PubMed.
- J. Wang, B. Elchert, Y. Hui, J. Y. Takemoto, M. Bensaci, J. Wennergren, H. Chang, R. Rai and C.-W. T. W. Chang, Bioorg. Med. Chem., 2004, 12, 6397–6413 CrossRef CAS PubMed.
- A. K. Sanki, J. Boucau, P. Srivastava, S. S. Adams, D. R. Ronning and S. J. Sucheck, Bioorg. Med. Chem., 2008, 16, 5672–5682 CrossRef CAS PubMed.
- A. K. Sanki, J. Boucau, F. E. Umesiri, D. R. Ronning and S. J. Sucheck, Mol. BioSyst., 2009, 5, 945–956 RSC.
- T. Warrier, M. Tropis, J. Werngren, A. Diehl, M. Gengenbacher, B. Schlegel, M. Schade, H. Oschkinat, M. Daffe, S. Hoffner, A. N. Eddine and S. H. Kaufmann, Antimicrob. Agents Chemother., 2012, 56, 1735–1743 CrossRef CAS PubMed.
- D. A. Ibrahim, J. Boucau, D. H. Lajiness, S. K. Veleti, K. R. Trabbic, S. S. Adams, D. R. Ronning and S. J. Sucheck, Bioconjugate Chem., 2012, 23, 2403–2416 CrossRef CAS PubMed.
- S. Gobec, I. Plantan, J. Mravljak, R. A. Wilson, G. S. Besra and D. Kikelj, Bioorg. Med. Chem. Lett., 2004, 14, 3559–3562 CrossRef CAS PubMed.
- A. Kovac, R. A. Wilson, G. S. Besra, M. Filipic, D. Kikelj and S. Gobec, J. Enzyme Inhib. Med. Chem., 2006, 21, 391–397 CrossRef CAS PubMed.
- A. A. Elamin, M. Stehr, W. Oehlmann and M. Singh, J. Microbiol. Methods, 2009, 79, 358–363 CrossRef CAS PubMed.
- C. S. Barry, K. M. Backus, C. E. Barry and B. G. Davis, J. Am. Chem. Soc., 2011, 133, 13232–13235 CrossRef CAS PubMed.
- L. Favrot, D. H. Lajiness and D. R. Ronning, J. Biol. Chem., 2014, 289, 25031–25040 CrossRef CAS PubMed.
- M. Jackson, C. Raynaud, M.-A. Lanéelle, C. Guilhot, C. Laurent-Winter, D. Ensergueix, B. Gicquel and M. Daffé, Mol. Microbiol., 1999, 31, 1573–1587 CrossRef CAS PubMed.
- K. Rainer and K.-B. Hendrik, Microbiol. Spectrum, 2014, 2, 1–15 Search PubMed.
- C. M. Sassetti and E. J. Rubin, Proc. Natl. Acad. Sci. U. S. A., 2003, 100, 12989–12994 CrossRef CAS PubMed.
- J. Rengarajan, B. R. Bloom and E. J. Rubin, Proc. Natl. Acad. Sci. U. S. A., 2005, 102, 8327–8332 CrossRef CAS PubMed.
- B. M. Swarts, C. M. Holsclaw, J. C. Jewett, M. Alber, D. M. Fox, M. S. Siegrist, J. A. Leary, R. Kalscheuer and C. R. Bertozzi, J. Am. Chem. Soc., 2012, 134, 16123–16126 CrossRef CAS PubMed.
|
This journal is © The Royal Society of Chemistry 2016 |