DOI:
10.1039/C5LC00867K
(Paper)
Lab Chip, 2016,
16, 112-119
Electroanalytical devices with pins and thread†
Received
23rd July 2015
, Accepted 27th October 2015
First published on 27th October 2015
Abstract
This work describes the adaptive use of conventional stainless steel pins—used in unmodified form or coated with carbon paste—as working, counter, and quasi-reference electrodes in electrochemical devices fabricated using cotton thread or embossed omniphobic RF paper to contain the electrolyte and sample. For some applications, these pin electrodes may be easier to modify and use than printed electrodes, and their position and orientation can be changed as needed. Electroanalytical devices capable of multiplex analysis (thread-based arrays or 96-well plates) were easily fabricated using pins as electrodes in either thread or omniphobic RF paper.
Introduction
Paper and thread have become increasingly attractive as substrates for the development of electroanalytical devices that are simple, portable, disposable and affordable.1 Others2–8 and we9,10 have described the development of microfluidic paper-based electroanalytical devices (EμPADs), and used this methodology to detect a wide variety of analytes, including small-molecule metabolites,6,9,11 metal ions,9 nucleic acids,2 and serum proteins.10 Thread-based microfluidic analytical devices (μTADs), first described by Shen,12 and by us,13 have since been used for several bioassays with colorimetric detection.12,14–17 Fewer thread-based than paper-based analytical devices have been integrated with electrochemical detection.18–21
One challenge in the development and accessibility of paper and thread-based electroanalytical devices is the ability to fabricate and integrate electrodes rapidly, efficiently and cost-effectively. The most widely used methods for fabrication of electrodes in paper-based2,4–11 and thread-based19,20 electroanalytical devices (e.g. screen printing, stencil printing, rotogravure, and chemical vapor deposition with shadow masking) require custom-patterned components such as screens, stencils, or masks to deposit conductive materials on the surface of a substrate.22–27 The fabrication of these custom-patterned components is time-consuming, and can be expensive. In the finished device, the position of the electrode cannot be altered after fabrication has been completed.
Existing strategies for the fabrication of electrodes are particularly challenging on non-planar substrates such as embossed hydrophobic,28,29 and omniphobic paper,29,30 and thread.12,13,16,17,19,20 The deposition of conductive materials on porous media such as paper or thread can alter its interfacial energy, porosity, tortuosity, and wicking. Inhomogeneity in the distribution of fluid can also affect the stability of the liquid electrode interface and influence the performance of the electroanalytical devices.31 These effects are most significant in the case of thread, where the fluid flow is confined to one dimension, and might be among the reasons behind the few examples of electroanalytical devices that utilize thread19,20 (in contrast to the widespread interest in those that utilize paper2–11).
We wished to develop a new class of electrodes that could expand the versatility of the paper and thread-based analytical systems developed by our group32,33 and by others.34–39 Adaptive use of prefabricated stainless steel pins—either unmodified, or coated with a thin layer of graphite ink—provides a simple solution to the problems of fabrication and integration of electrodes in a low-cost analytical device. Platinum, gold, copper and stainless steel wires have been used as electrodes in microfluidic electrochemical applications,3,40–43 but their integration with paper-based devices has so far been limited to the use of gold wires several microns in diameter reported by Fosdick et al.3 These gold microwire electrodes might be difficult to manipulate due to their small size, and require treatment with highly oxidizing solution (piranha etch) immediately prior to use; these safety concerns might make them challenging to use by minimally trained personnel, or at the point of care.
We show that stainless steel pins can be used as electrodes in systems fabricated using either omniphobic RF paper or thread; as electrodes, pins are sensitive and can be used to quantify metabolites (e.g., lactate in human plasma). Their macroscopic size and minimal requirements for cleaning and post-processing make them easy to handle in settings where resources are sparse. Surprisingly, because they offer readily accessible connection points to electrochemical readers and easily modifiable configurations, pin electrodes allow the fabrication of devices suitable for multiplexed analysis. We demonstrate the fabrication of thread-based arrays that can be used to detect different analytes in the same array, or to perform multiple measurements of the same analyte simultaneously, or in close succession. We also describe the fabrication of a 96-well plate in omniphobic RF paper that can be used to perform independent measurements in each well.
Results and discussion
Design of the RF paper-based and thread-based devices incorporating pins as electrodes
Stainless-steel pins have several characteristics that make them attractive as candidates for adaptive use as electrodes in electrochemical devices. Stainless steel pins are inexpensive (less than $0.001 per pin when purchased from commercial retailers, and much less if purchased wholesale) and available nearly all over the globe. Stainless steel is highly conductive and stable electrochemically in neutral or mildly acidic or basic aqueous solutions.47,48 Different parts of a pin (the head, the shaft and the sharp tip) can be used for different purposes: the head can serve as an electrode in omniphobic paper-based devices, part of the stem can serve as an electrode in thread-based devices, the stem can be used for connection to the potentiostat, and the sharp tip can be used to anchor the pins in a mechanical support. To generate an electroactive surface area of the working electrode that is sufficiently large to be useful for analysis, we coated a stainless-steel pin with carbon ink prepared by mixing graphite paste and solvent thinner with a multi-walled carbon nanotube powder49 (details in the ESI†).
We have chosen paper and thread as a substrate for the fabrication of the electrochemical cells because they are widely available, inexpensive, lightweight, and flexible. We recently demonstrated that treatment with an organosilane in the gas-phase can be used to transform cellulose paper into a material resistant to wetting by a wide range of liquids, while leaving the characteristic properties of paper (flexibility, foldability, mechanical flexibility, porosity, large surface area, light weight, low cost) unaltered. The resulting “fluoroalkylated paper,” or “RF paper” is omniphobic (i.e. both hydrophobic and oleophobic), and thus resists wetting by liquids spanning a broader range of surface tensions than paper treated with non-fluorinated alkyl trichlorosilanes (“non-fluorinated paper”, or “RH paper”). The combination of old and new properties allowed the use of RF and RH papers in a variety of new applications—open-channel microfluidics,45,46 printed electronics and MEMS,30 and low-cost diagnostics.28 Here, we took advantage of these properties of omniphobic RF paper to develop a simple and flexible liquid storage system that provides sufficient mechanical support for the positioning of the pin electrodes, and can be manufactured inexpensively, using embossing44 and gas-phase silanization.29
We show in Fig. 1A the strategy used for the fabrication of an RF paper-based electrochemical cell in which the pins–serving as working electrode (WE), reference electrode (RE), and counter electrode (CE)—were inserted in an embossed well. The electrodes were placed 0.1 in (~2.53 mm) away from one another, using a transparency with precut holes as an alignment tool. Fig. 1B shows the design of an electrochemical cell, in which pins (WE, RE, CE) are surrounded by helical turns of thread. To allow the solution of analyte to contact the electrodes, we used a micropipette to add a drop of liquid either to the embossed omniphobic well or to the thread.
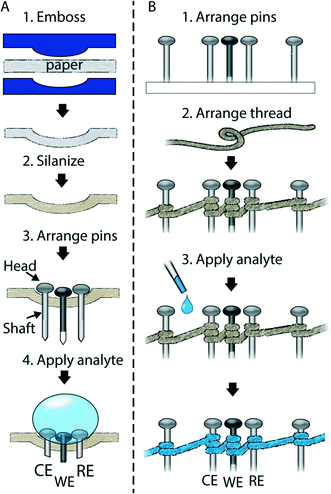 |
| Fig. 1 A) Schematic representation of the process used for the fabrication of electrochemical cells in embossed omniphobic RF paper. B) Schematic representation of the process used for the fabrication of an electrochemical cell with cotton thread. In both cases we use stainless steel pins as reference and counter electrodes (RE and CE), and a stainless steel pin coated with a graphite and carbon nanotube ink as working electrode (WE). | |
In the omniphobic paper-based device, the liquid rests on the surface of the well, and forms an interface with the surfaces of the heads of the pins. Here the area between the head and the liquid determines the area of the electrode-fluid interface – which is the geometrical electrode area (Fig. 2A1 and A2).
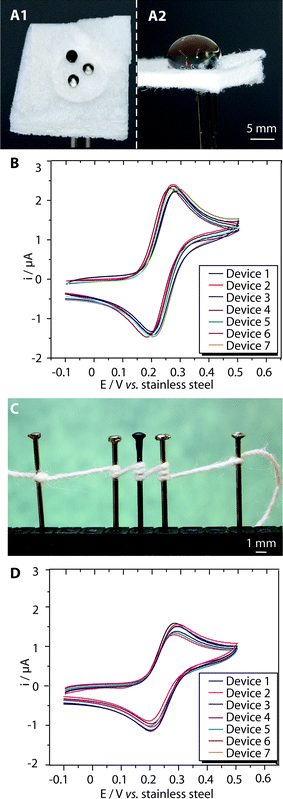 |
| Fig. 2 (A1, A2) Photographs of an electrochemical cell fabricated using embossed omniphobic RF paper and stainless steel pins as reference and counter electrodes (RE and CE), and a stainless steel pin coated with a graphite and carbon nanotube ink as working electrode (WE). The electrodes are placed at a distance of ~0.1 in (2.53 mm) away from one another. (C) Photograph of an electrochemical cell fabricated using cotton thread and stainless steel pins as reference and counter electrodes, and a stainless steel pin coated with a graphite and carbon nanotube ink as working electrode. The electrodes are placed at a distance of ~0.1 in (2.53 mm) away from one another. Cyclic voltammograms recorded in a 500 μM solution of FcCO2H in 1× PBS, pH 7.6 at a scan rate of 100 mV s−1 using: (B) seven independent embossed omniphobic RF paper devices, and (D) seven independent thread-and-pin arrays. | |
On thread, the liquid wicks along the thread and forms a cylindrical interface with the shaft of each pin (Fig. 2C), and the area of the thread in contact with the shaft determines the area of the electrode–fluid interface. The approximate geometrical areas of the paper and thread interfaces are 5 mm2 and 4 mm2, respectively.
Evaluation
We evaluated the performance of the pins as electrodes in thread-based and omniphobic RF paper-based electrochemical cells by recording the cyclic voltammograms (CVs) at a scan rate of 100 mV s−1 in a solution of a redox probe with well-characterized electrochemical behavior (ferrocene carboxylic acid, FcCO2H). Fig. 2B and D show the variation in the CVs of the solution of FcCO2H (at 100 μM in 1×PBS, pH 7.6) for paper-based and thread-based cells, each recorded using seven different devices. The device-to-device variation in the performance of the electrodes, measured from the anodic peak current ipa, was in both cases, less than 10% as indicated by the relative standard deviation, RSD, (defined as the percentage ratio of the standard deviation to the mean of the distribution) of 6.3% (for an average current
= 2.2 μA) and 9.4% (
= 1.6 μA), for paper and thread-based cells, respectively.
We found that by controlling the number of helical turns, and the spacing between the turns, of the thread surrounding each pin electrode, we were able to control the area of the electrode–electrolyte interface and reduce the device-to-device variation (see the ESI† for details). For the thread-based cells we did not find the results to vary with the tension of the thread, at least within the range of tensions that allowed the formation of stable electrode–electrolyte interfaces between the thread and the pin electrodes and allowed the measurement of a stable current (although we did not explicitly measure this tension). We believe that the electrolyte interface between the thread and the pin, an area that is defined largely by the wetting properties of the two surfaces, determines the device-to-device variation.
To determine whether the electrochemical processes at the pin electrode–liquid interface in RF paper and thread-based devices are diffusion-controlled, we recorded cyclic voltammograms of 500 μM FcCO2H in PBS, pH 7.6, at scan rates between 10 and 300 mV s−1. The anodic and cathodic peak currents (ipa and ipc) were linearly proportional to the square root of the scan rate in both RF paper (R2 = 0.991 and 0.992, respectively) and thread-based cells (R2 = 0.986 and 0.989, respectively) (see Fig. 3C). These results indicate that, in both cases, the rate of the electrochemical reaction at the surface of the pin electrode is governed by the diffusion of FcCO2H to the surface of the electrode. These results are in agreement with results of finite-element simulations confirming that, for short diffusion distances and high scan rates, the geometry of the electrode (cylindrical or hemispherical, depending on whether the shaft or the head of the pin forms an interface with the solution of analyte) does not influence the process. Thus, under our experimental conditions, the electrochemical cells fabricated using pin electrodes and either thread or RF paper can reproduce the classical diffusion-limited electrochemical processes reported in EμPADs incorporating screen-printed electrodes.
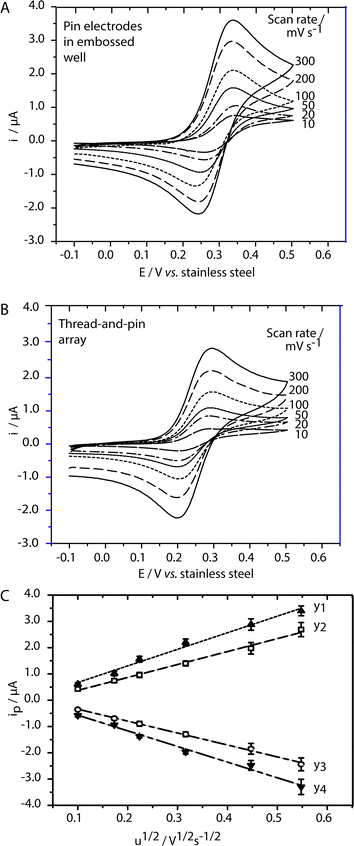 |
| Fig. 3 Cyclic voltammograms of 500 μM FcCO2H in 1× PBS (pH 7.6) in electrochemical cells fabricated using (A) embossed omniphobic RF paper and (B) thread, at various scan rates ascending along y-axis: 10, 20, 50, 100, 200, 300 mV s−1. (C) The plot of anodic and cathodic peak currents vs. the square root of the scan rate (ν1/2). CV experiments conducted on an omniphobic RF paper device (up-pointing triangle ▲: anodic peak current, down-pointing triangle ▼: cathodic peak current) and in a thread device (squares □: anodic peak current, circles ○: cathodic peak current). The dashed lines represent linear regressions with equations: y1 = 6x μA V−1/2/s1/2 + 0.1 μA [R2 = 0.991], y2 = 5x μA V−1/2 s1/2 − 0.05 μA [R2 = 0.986], y3 = −4x; μA V−1/2 s1/2 + 0.05 μA [R2 = 0.989], y3 = −6x μA V−1/2 s1/2 − 0.05 μA [R2 = 0.992]. Error bars correspond to standard deviation of measurements performed using seven independent devices. | |
These results also confirmed that the stainless steel was electrochemically stable and did not make any noticeable contribution to the electrochemical processes that occurred at the working electrode. We chose stainless steel pins, rather than pins made from less inert materials such as copper or silver, because they were highly stable under our experimental conditions (i.e. we did not observe any electrochemical processes typical of the oxidation of iron). The stability of stainless steel electrodes has also been reported by others.47,48 In addition, the surface of the stainless steel pins intended to serve as working electrodes was coated with three layers of carbon ink, further reducing the risk of oxidation.
Applications in clinical diagnostics: analysis of L-lactate in human serum
We evaluated the feasibility of using RF paper-based and thread-based devices to measure the concentration of a clinically relevant analyte, L-lactate, in human serum. The range of L-lactate concentrations relevant for diagnosis is between 0.5 and 15–20 mM in serum. We used chronoamperometry to perform this demonstration of principle because it is a simple and frequently used technique that provides a quantitative result. Cyclic voltammetry (CV) is less useful for accurate quantitation of electroactive species than chronoamperometric or pulse voltammetric techniques, because the correction for the capacitive current in CV is typically ambiguous.50 Chronoamperometry measures current as a function of time at constant applied voltages, and starts with a large capacitive current that decays within the first few seconds. Faradaic current, which is proportional to the concentration of the analyte, becomes dominant, and decays according to the Cottrell equation (eqn (1)), where n is the number of electrons, F is Faraday's constant, A is the area of the electrode, D is the diffusion coefficient of analyte, C is the concentration of analyte, and t is time. |  | (1) |
In the lactate assay, potassium ferricyanide, K3[Fe(CN)6], served as a mediator (eqn (2)–(4)):
|  | (2) |
|  | (3) |
|  | (4) |
For each mole of lactate that is oxidized, two moles of [Fe(CN)
6]
3− are reduced to [Fe(CN)
6]
4−; the latter can be quantified using chronoamperometry at an applied voltage of 0.4 V
vs. a stainless-steel quasi-reference electrode.
Fig. 4 shows the calibration curves for the measurement of
L-lactate, for values between 1.1 mM (the value initially present in the serum) and 20 mM (with additional lactate spiked in the serum). The sensitivity is 0.08 μA mM
−1 for the R
F paper- and 0.06 μA mM
−1 for the thread-based devices.
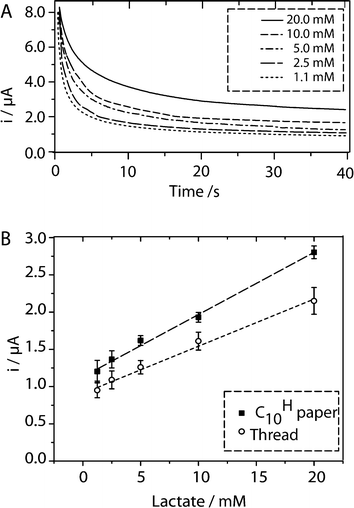 |
| Fig. 4 (A) Chronoamperograms recorded on thread for the mixture of the enzymatic assay for the determination of lactate, at concentrations between 1.1–20 mM. The chronoamperograms were recorded at 0.4 V versus a stainless steel quasi-reference pin electrode. (B) The calibration plots of the currents recorded after 40 s as a function of concentration of lactate on thread-and-pins arrays and in wells embossed in omniphobic RF paper. The long-dash line represent a fit to the equation: y = 0.08x + 1.13 (R2 = 0.994) whereas the short-dash line represents a fit to the equation: y = 0.06x + 0.91 (R2 = 0.987), for concentrations of lactate between 1.1–20 mM. Error bars correspond to standard deviation of measurements performed using 7 independent devices. | |
Approaches to multiplexing: electrochemical 96 well plate.
We prepared a 96-well plate capable of carrying out parallel analyses of different analytes, using embossed omniphobic RF paper as a substrate, and pins as electrodes. Fig. 5 shows that different wells can be used to perform independent analyses—cyclic voltammetry for the analysis of solutions of FcCO2H and hydroquinone, respectively.
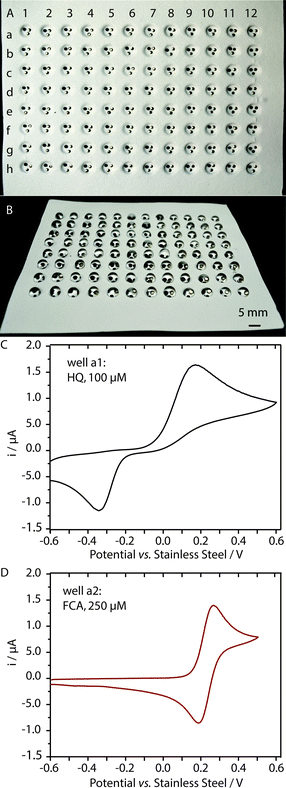 |
| Fig. 5 Photography of a 96 well plate fabricated using embossed omniphobic RF paper and pin electrodes. (A) before and (B) after 50 μL drops of an aqueous solution are added to each well. (C and D) Independent voltammograms recorded in different wells (a1 and a2) of the plate: a 100 μM solution of hydroquinone (HQ), and a 250 μM solution of FCA, both in 1× PBS, pH 7.6, recorded at a scan rate of 100 mV s−1; the presence of a different analyte in a neighboring well does not interfere with measurements. | |
Approaches to multiplexing: thread-based arrays of pin electrodes
We produced linear arrays of electrodes (carbon-coated stainless steel pins as working electrodes, and stainless steel pins as either counter or quasi-reference electrodes) that form interfaces with the liquid wicking along the same thread. The electrochemical cells within the thread-based arrays can be either linked or independent, such that each cell in a multiplex device can be used to perform, in rapid succession or simultaneously, independent measurements for one or several solutions of analyte along the same thread.
Fig. 6 shows chronoamperograms for the same solution of analyte, recorded, in succession, using each of the seven WEs positioned along a single thread. In each measurement, the two adjacent stainless steel pins served as CE and RE, such as each two successive cells share one stainless steel pin that serves as a counter electrode in the former and as reference electrode in the latter.
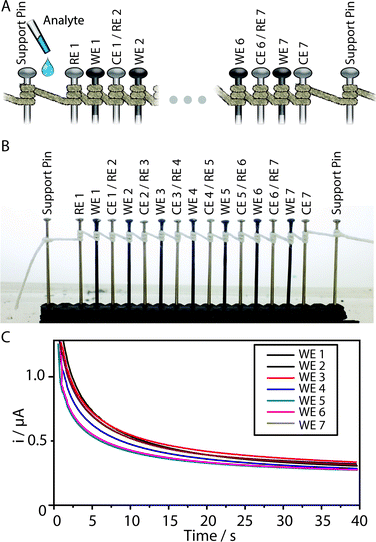 |
| Fig. 6 (A) Schematic and (B) photograph of a device comprising multiple alternating stainless steel pins and carbon-coated stainless steel pins and a single thread that forms three helical turns around each pin. The device can be used for multiple measurements (in rapid succession) of the same analyte on thread. (C) Chronoamperograms at 0.4 V recorded with each of the seven cells along the thread, using in each case the two adjacent stainless steel pins as CE and RE. Solution: 100 mM potassium ferrocyanide in 1× PBS pH 7.6. | |
By introducing hydrophobic barriers along the thread, we can form independent electrochemical cells capable of performing different analyses along the same thread. Fig. 7 shows square-wave voltammograms recorded with each of the three electrochemical cells along a single thread, for three solutions with different concentrations of analyte: 10 μM FcCO2H in PBS, pH 7.6; buffer only, PBS, pH 7.6; and 100 μM FcCO2H in PBS, pH 7.6. There is no observable interference between neighboring cells.
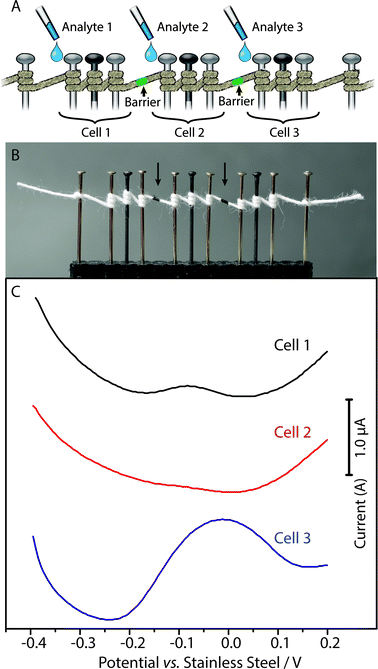 |
| Fig. 7 (A) A schematic and (B) a photograph of a device comprising three distinct electrochemical cells formed from a single thread; arrows indicate the presence of a small amount of polymer (cyanoacrylate) that serves as a boundary between consecutive cells. The device can be used for measurement of three different analytes (in rapid succession or simultaneously). (C) Square-wave voltammograms recorded with each electrochemical cell, for: (1) left: 10 μM FCA in PBS, pH 7.6; (2) middle: buffer only, PBS, pH 7.6; (3) right: 100 μM FCA in PBS, pH 7.6. The center cell, where the thread is wet with buffer only, has no observable interference with neighboring cells. | |
Conclusion
One unmet need in the development of low-cost electroanalytical devices is a method to assemble and reconfigure devices “on-the fly”, to meet the needs of specific applications and settings. The strategy we describe in this paper is based on electrodes that are by themselves nearly as ubiquitous, portable, inexpensive, and easily storable as are paper and thread. The combination of stainless-steel pins—untreated or coated with a thin layer of carbon ink—and embossed omniphobic RF paper or cotton thread, provides the basis for the fabrication of simple, versatile and low-cost electroanalytical devices. Our pin-based devices have four advantages: i) they are inexpensive, lightweight and portable, ii) they rely on stainless steel pins are mass-produced at very low cost and readily available, iii) they are compatible with biological samples, iv) they eliminate the need for patterning electronic vias through the microfluidic substrate. The pins go through the paper, allowing three-dimensional electronic connections to a potentiostat or another instrument. This property is especially useful for multi-well electrochemical plates/substrates, where we believe the devices can interface with existing instrumentation for multiplexed electrochemical readout.
With further development, the paper and thread-based devices fabricated using this method have the potential to provide new functional options in clinical diagnostics, environmental monitoring, and microfluidic and electronic systems.
Acknowledgements
M. T. Fernández-Abedul thanks the Spanish Ministry of Economy and Competitiveness (project MICINN CTQ2011-25814) for funding her stay at Harvard University. A. Ainla thanks the Swedish Research Council (VR) for a postdoctoral fellowship. A. C. Glavan acknowledges funding by the Bill & Melinda Gates Foundation, award # 51308 and DTRA award # HDTRA1-14-C-0037. M. M. Hamedi acknowledges support from Marie Curie IOF FP7 for project nanoPAD (Grant Agreement Number 330017).
References
- E. J. Maxwell, A. D. Mazzeo and G. M. Whitesides, MRS Bull., 2013, 38, 309–314 CrossRef CAS.
- J. C. Cunningham, N. J. Brenes and R. M. Crooks, Anal. Chem., 2014, 86, 6166–6170 CrossRef CAS PubMed.
- S. E. Fosdick, M. J. Anderson, C. Renault, P. R. DeGregory, J. A. Loussaert and R. M. Crooks, Anal. Chem., 2014, 86, 3659–3666 CrossRef CAS PubMed.
- R. F. Carvalhal, M. S. Kfouri, M. H. Piazetta, A. L. Gobbi and L. T. Kubota, Anal. Chem., 2010, 82, 1162–1165 CrossRef CAS PubMed.
- C. K. Tang, A. Vaze and J. F. Rusling, Anal. Methods, 2014, 6, 8878–8881 RSC.
- W. Dungchai, O. Chailapakul and C. S. Henry, Anal. Chem., 2009, 81, 5821–5826 CrossRef CAS PubMed.
- A. Apilux, W. Dungchai, W. Siangproh, N. Praphairaksit, C. S. Henry and O. Chailapakul, Anal. Chem., 2010, 82, 1727–1732 CrossRef CAS PubMed.
- M. Santhiago, C. S. Henry and L. T. Kubota, Electrochim. Acta, 2014, 130, 771–777 CrossRef CAS.
- Z. Nie, C. A. Nijhuis, J. Gong, X. Chen, A. Kumachev, A. W. Martinez, M. Narovlyansky and G. M. Whitesides, Lab Chip, 2010, 10, 477–483 RSC.
- Z. Nie, F. Deiss, X. Liu, O. Akbulut and G. M. Whitesides, Lab Chip, 2010, 10, 3163–3169 RSC.
- H. Liu, Y. Xiang, Y. Lu and R. M. Crooks, Angew. Chem., 2012, 124, 7031–7034 CrossRef.
- X. Li, J. Tian and W. Shen, ACS Appl. Mater. Interfaces, 2009, 2, 1–6 Search PubMed.
- M. Reches, K. A. Mirica, R. Dasgupta, M. D. Dickey, M. J. Butte and G. M. Whitesides, ACS Appl. Mater. Interfaces, 2010, 2, 1722–1728 CAS.
- X. Li, D. R. Ballerini and W. Shen, Biomicrofluidics, 2012, 6, 011301–011313 CrossRef PubMed.
- D. R. Ballerini, X. Li and W. Shen, Biomicrofluidics, 2011, 5, 014105 CrossRef PubMed.
- G. Zhou, X. Mao and D. Juncker, Anal. Chem., 2012, 84, 7736–7743 CrossRef CAS PubMed.
- D. R. Ballerini, X. Li and W. Shen, Anal. Bioanal. Chem., 2011, 399, 1869–1875 CrossRef CAS PubMed.
- M. Hamedi, R. Forchheimer and O. Inganas, Nat. Mater., 2007, 6, 357–362 CrossRef CAS PubMed.
- N. C. Sekar, S. A. Mousavi Shaegh, S. H. Ng, L. Ge and S. N. Tan, Electrochem. Commun., 2014, 46, 128–131 CrossRef CAS.
- Y.-C. Wei, L.-M. Fu and C.-H. Lin, Microfluid. Nanofluid., 2013, 14, 583–590 CrossRef CAS.
- T. Choudhary, G. P. Rajamanickam and D. Dendukuri, Lab Chip, 2015, 15, 2064–2072 RSC.
- J. P. Hart and S. A. Wring, TrAC, Trends Anal. Chem., 1997, 16, 89–103 CrossRef CAS.
- X. Xu, S. Zhang, H. Chen and J. Kong, Talanta, 2009, 80, 8–18 CrossRef CAS PubMed.
- F. Eder, H. Klauk, M. Halik, U. Zschieschang, G. Schmid and C. Dehm, Appl. Phys. Lett., 2004, 84, 2673–2675 CrossRef CAS.
- D. Tobjork and R. Osterbacka, Adv. Mater., 2011, 23, 1935–1961 CrossRef PubMed.
- O. Dominguez Renedo, M. A. Alonso-Lomillo and M. J. Arcos Martinez, Talanta, 2007, 73, 202–219 CrossRef PubMed.
- D. M. Cate, J. A. Adkins, J. Mettakoonpitak and C. S. Henry, Anal. Chem., 2014, 87, 19–41 CrossRef PubMed.
- A. C. Glavan, D. C. Christodouleas, B. Mosadegh, H.-D. Yu, B. S. Smith, J. Lessing, M. T. Fernández-Abedul and G. M. Whitesides, Anal. Chem., 2014, 86, 11999–12007 CrossRef CAS PubMed.
- A. C. Glavan, R. V. Martinez, A. B. Subramaniam, H. J. Yoon, R. M. D. Nunes, H. Lange, M. M. Thuo and G. M. Whitesides, Adv. Funct. Mater., 2014, 24, 60–70 CrossRef CAS.
- J. Lessing, A. C. Glavan, S. B. Walker, C. Keplinger, J. A. Lewis and G. M. Whitesides, Adv. Mater., 2014, 26, 4677–4682 CrossRef CAS PubMed.
- C. Renault, M. J. Anderson and R. M. Crooks, J. Am. Chem. Soc., 2014, 136, 4616–4623 CrossRef CAS PubMed.
- A. W. Martinez, S. T. Phillips, M. J. Butte and G. M. Whitesides, Angew. Chem., Int. Ed., 2007, 46, 1318–1320 CrossRef CAS PubMed.
- A. W. Martinez, S. T. Phillips, E. Carrilho, S. W. Thomas, H. Sindi and G. M. Whitesides, Anal. Chem., 2008, 80, 3699–3707 CrossRef CAS PubMed.
- E. M. Fenton, M. R. Mascarenas, G. P. Lopez and S. S. Sibbett, ACS Appl. Mater. Interfaces, 2009, 1, 124–129 CAS.
- H. Liu and R. M. Crooks, J. Am. Chem. Soc., 2011, 133, 17564–17566 CrossRef CAS PubMed.
- H. Liu, X. Li and R. M. Crooks, Anal. Chem., 2013, 85, 4263–4267 CrossRef CAS PubMed.
- D. Zang, L. Ge, M. Yan, X. Song and J. Yu, Chem. Commun., 2012, 48, 4683–4685 RSC.
- X. X. Yang, O. Forouzan, T. P. Brown and S. S. Shevkoplyas, Lab Chip, 2012, 12, 274–280 RSC.
- P. Yager, T. Edwards, E. Fu, K. Helton, K. Nelson, M. R. Tam and B. H. Weigl, Nature, 2006, 442, 412–418 CrossRef CAS PubMed.
- Y. Liu, J. A. Vickers and C. S. Henry, Anal. Chem., 2004, 76, 1513–1517 CrossRef CAS PubMed.
- M. Castaño-Díaz, M. T. Fernández-Abedul and A. Costa-García, J. Chromatogr. A, 2006, 291–299 CrossRef PubMed.
- M. Wojciechowski and J. Balcerzak, Anal. Chim. Acta, 1990, 237, 127–133 CrossRef CAS.
- M. T. Fernández-Abedul and A. Costa-García, Anal. Chim. Acta, 1996, 328, 67–71 CrossRef.
- H. Becker and C. Gartner, Electrophoresis, 2000, 21, 12–26 CrossRef CAS PubMed.
- A. C. Glavan, R. V. Martinez, E. J. Maxwell, A. B. Subramaniam, R. M. D. Nunes, S. Soh and G. M. Whitesides, Lab Chip, 2013, 13, 2922–2930 RSC.
- M. M. Thuo, R. V. Martinez, X. Liu, M. B. J. Atkinson, J. F. Bloch and G. M. Whitesides, Chem. Mater., 2014, 26, 4230–4237 CrossRef CAS.
- H. Ayoub, V. Lair, S. Griveau, P. Brunswick, F. Bedioui and M. Cassir, Electroanalysis, 2012, 24, 1324–1333 CrossRef CAS.
- A. U. Malik, P. C. Mayan Kutty, N. A. Siddiqi, I. N. Andijani and S. Ahmed, Corros. Sci., 1992, 33, 1809–1827 CrossRef CAS.
- M. T. Fernández-Abedul and A. Costa-García, Anal. Bioanal. Chem., 2008, 390, 293–298 CrossRef PubMed.
-
A. J. Bard and L. R. Faulkner, Electrochemical Methods, John Wiley & Sons, New York, 2001 Search PubMed.
Footnote |
† Electronic supplementary information (ESI) available. See DOI: 10.1039/c5lc00867k |
|
This journal is © The Royal Society of Chemistry 2016 |