DOI:
10.1039/C5GC00970G
(Paper)
Green Chem., 2016,
18, 307-314
Hybrid bipolar membrane electrodialysis/ultrafiltration technology assisted by a pulsed electric field for casein production
Received
6th May 2015
, Accepted 7th July 2015
First published on 28th July 2015
Abstract
Electrodialysis with bipolar membranes (EDBM) is an ecofriendly technology providing a wide spectrum of solutions for modern industry. The main advantage of EDBM is the absence of chemicals during the treatment, which makes it very attractive especially in the food and pharmaceutical sectors. The production of casein, the major milk protein, by means of EDBM is a very interesting approach in the sustainable development context due to the high product purity, no waste generation and the absence of hazardous reagents. Casein is widely used as a food additive in order to improve the food nutritional value as well as to create the desirable functional properties. Moreover, casein is a source of bioactive peptides having beneficial effects on human health. However, the major obstacle hampering the industrial application of EDBM for the production of casein with improved quality is precipitation of casein inside the EDBM stack and membrane scaling. Here we propose a hybrid technology comprising an EDBM module coupled with an ultrafiltration (UF) module. Our results show that the use of the UF module prior to EDBM allows complete prevention of casein precipitation inside the EDBM stack which plays a crucial role in the improvement of EDBM efficiency. In addition, we have found that electroacidification may be performed until pH 5.0 instead of the conventional value of 4.6, which allows a substantial decrease in membrane scaling. Finally, application of pulsed electric field mode allows inhibition of scale formation and OH− leakage, which hastens the EDBM process and increases the membrane lifetime.
1. Introduction
Proteins play an important role in the maintenance of the normal body composition and function throughout the life cycle. In addition, proteins are a source of bioactive peptides having beneficial effects on cardiovascular, nervous, gastrointestinal and immune systems preventing hypertension, diabetes, cancer and other diseases.1 Furthermore, modern trends are directed away from a high carbohydrate diet towards a high protein diet2–4 in order to prevent obesity and risks of cardiovascular diseases.5 To satisfy the demands for increased protein levels, modern industry proposes the use of protein ingredients. Caseins, the major proteins in milk, are widely used as food ingredients in order to increase the nutritional value of food as well as to provide functional benefits such as structure formation, foaming, heat stability, water binding and emulsification.6 There are two main casein types, rennet casein and acid casein, which are usually produced by industries. Rennet-induced casein coagulation comprises two stages: (1) application of a special enzyme for the hydrolysis of κ-casein with production of para-κ-casein and casein macropeptides and (2) coagulation of para-κ-casein by Ca2+. Acid-induced precipitation is based on a pH decrease until the isoelectric point of caseins is reached by addition of an acid, by fermentation or by application of cation-exchange resins.7 In addition to the conventional methods, several alternative methods have been reported, such as the use of ethanol, ultrafiltration followed by cryo-destabilization, the use of anionic polysaccharides, high-pressure CO2 precipitation, electrodialysis coupled with mineral acid addition, etc.7,8 Bazinet et al.8 reported the successful application of an ecofriendly membrane technology for casein production. The proposed approach is a variant of isoelectric casein precipitation without any use of chemicals by means of electrodialysis with bipolar membranes (EDBM). EDBM technology allows modification of pH via water dissociation at a bipolar membrane (BM) under the effect of an applied electric field resulting in the production of H+ and OH−. In the case of milk, electroacidification until pH = 4.6 results in the precipitation of casein with a small ash content due to the additional milk demineralization during EDBM. In spite of the attractiveness of EDBM, precipitation of casein inside the stack and scaling on the cation-exchange membrane affect the process performance hampering the industrial application of this technique.8 To answer this problem, Balster et al.9 proposed a complex approach to avoid clogging of the EDBM stack by caseins. This approach consists of a classical chemical acidification (for the first batch) of milk in a precipitator followed by separation of casein from whey. The whey flux is further directed to the EDBM stack for demineralization and neutralization. The acid generated in the acidification compartment of EDBM is then used in the precipitator for further milk acidification. Mier et al.10 placed an on-line basket centrifuge allowing the separation of whey from casein behind the EDBM cell and in front of the milk reservoir. In spite of the promising results of the above studies, the presence of scaling and organic fouling by whey proteins or by casein curd was reported.
In this work, we propose an alternative approach comprising an ultrafiltration (UF) module prior to the EDBM module, electroacidification at a higher pH value (5.0) and application of a pulsed electric field (PEF). First of all, the UF module would allow prevention of casein curd formation inside the EDBM stack due to the retention of the milk protein fraction by the UF membrane with a low molecular weight cut-off. In fact, the UF permeate (MUF) containing no protein instead of milk is electroacidified in the EDBM stack and proceeds to the milk reservoir where isoelectric precipitation of caseins occurs (Fig. 1). Secondly, electroacidification until pH 5.0 instead of 4.6 would allow a decrease in scaling since a part of the Ca2+ (Mg2+) scaling ions remains bonded with casein micelles11,12 and free Ca2+ (Mg2+) ions migrate substantially at a pH lower than 5.0 due to the predominant migration of K+ ions at higher pH values.13 Thus, most part of scaling ions remains in the diluate compartment with acid medium which is unfavorable for scaling formation. Thirdly, the application of PEF to the EDBM module would hamper the fouling and scaling formation. Indeed, recent studies reported the prevention of scaling (over 85%) and protein fouling (up to 100%) by application of PEF.14–16 An additional benefit of PEF is the prevention of concentration polarization phenomenon (CP) on ion-exchange membranes. Indeed, the concentration polarization phenomenon is the emergence of a concentration gradient at the membrane/solution interface, which arises due to the ability of the membrane to transport more readily certain types of species under the effect of the transmembrane driving force. CP hampers the flux of species, which decreases the efficiency and increases the energy consumption of the process.16
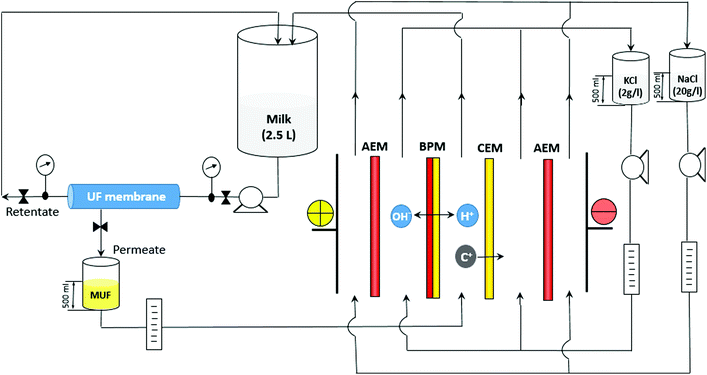 |
| Fig. 1 Configuration of the EDBM-UF system coupling an electrodialysis with a bipolar membrane (EDBM) module and an ultrafiltration (UF) module. C+ are migrating cations. | |
2. Experimental section
2.1 Configuration of electrodialysis with the bipolar membrane (EDBM) module and the ultrafiltration (UF) module
The EDBM (Fig. 1) module used was a laboratory scale cell (Model MP, 100 cm2 of effective surface) from ElectroCell Systems AB Company (Täby, Sweden). The cell consists of five compartments separated by two Neosepta AMX-SB anion-exchange membranes, one Neosepta CMX-SB cation-exchange membrane and one Neosepta BP-1 bipolar membrane: all these membranes manufactured by Tokuyama Soda Ltd (Tokyo, Japan) are food grade membranes. The three electrolytes: skim milk (EDBM) (2.5 L, 150 ml min−1) or ultrafiltrated milk fraction (MUF) (EDBM-UF), 2 g l−1 KCl (500 ml, 150 ml min−1) and 20 g l−1 NaCl (500 ml, 500 ml min−1) were circulated using three centrifuge pumps. The anode, a dimensionally-stable electrode (DSA) and the cathode, a 316 stainless steel electrode, were supplied with the MP cell. The UF module (Fig. 1) was equipped with a spiral wound membrane with a molecular weight cut-off of 10 kDa and a surface of 4200 cm2 (GE Water and Process Technologies, model PW1812T, Vista, USA). The UF system was run at a room temperature (22 ± 1 °C) under a pressure of 25 psi.
2.2 Protocol
A scheme of different modes of EDBM tested and research questions to be answered are shown in Fig. 2. EDBM was carried out as a batch process using a constant current density of 20 mA cm−2 generated by a Xantrex power supply (Model HPD 60-5SX; Burnaby, Canada). The electroacidification was stopped after the pH reached 5.4 due to the high global system resistance. For EDBM-UF, the permeate from the UF module (MUF) passed directly to the EDBM cell and electroacidification was stopped when the pH in the UF reservoir reached 4.6 or 5.0. These two pH values were chosen in order to evaluate the influence of pH of electroacidification on CMX-SB scaling. In addition to the continuous current mode of EDBM treatment, pulsed electric field (PEF) mode with pulse/pause lapses 2 s/0.5 s was tested to hamper the scaling formation. This pulse/pause duration was reported to be the optimal PEF mode allowing the best scaling inhibition among all PEF modes tested.16 Three replicates of each mode were performed. During each treatment, 1.5 ml samples of the acidified milk solution were taken at every 0.4 pH unit decrease. The time required to reach the final pH value, the anode/cathode voltage difference and the temperature were recorded as the treatment progressed. The concentration of soluble proteins in the supernatants of freshly acidified 1.5 mL samples after centrifugation (10
000g, 4 °C and 10 min) was determined. After electroacidification, photographs of the dismantled EDBM cell and CMX-SB membranes were taken. Membrane thickness, ash content, inductive coupled plasma analysis, scanning electron microscopy analysis, energy dispersive X-ray spectroscopy were carried out on CMX-SB in order to evaluate the quantity, structure and composition of membrane scaling.
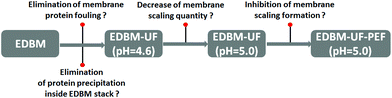 |
| Fig. 2 Scheme of the different EDBM configurations tested and research questions to be answered. | |
3. Results and discussion
3.1 Characterization of fouling
3.1.1 Casein fouling.
As expected during conventional EDBM of milk, casein precipitation inside the acidification compartment occurred (Fig. 3a). This is in agreement with the work of Bazinet et al.8 who reported the same precipitation effect. However, these authors used higher flow rates and modified spacers, which allowed the continuation of the EDBM treatments until pH 4.0. In the present work, a relatively low flow rate was applied in order to compare conventional EDBM and EDBM-UF where the maximum flow rate of the UF permeate was 150 ml min−1. At this flow rate, EDBM treatment was stopped after the pH in the milk reservoir reached 5.4 due to the complete clogging of the acidification compartment (Fig. 3a). Casein curd blocks the spacers between membranes and hinders the surface of CEM and BM making impossible to continue following acidification. Coupling of the EDBM cell with the ultrafiltration module seems to be a very promising solution. Indeed, Fig. 3(b) shows the absence of casein curd inside the EDBM stack at the end of electroacidification. In fact, the major protein fractions of milk including caseins and whey proteins were rejected by the ultrafiltration membrane with a molecular weight cut-off of 10 kDa. Therefore, the coupling approach allows prevention of organic fouling caused either by caseins or by whey proteins.
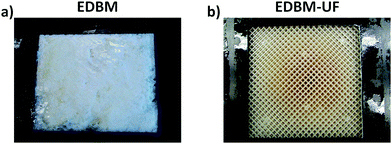 |
| Fig. 3 Photographs of spacers in the acidification compartment of EDBM: (a) conventional EDBM, (b) EDBM-UF. | |
3.1.2 Scaling.
3.1.2.1 Ash content and inductively coupled plasma (ICP) analysis.
The lowest mineral content was observed for the CMX-SB treated by the conventional EDBM procedure (Fig. 4a). However, in this condition electroacidification was performed just until pH = 5.4 and it is well known that at this pH part of Ca2+ and Mg2+ ions still remains bonded with casein micelles.11,12 Moreover, until pH 5.0 K+ ions migrate predominantly towards the alkaline compartment and most part of free Ca2+ and Mg2+ ions remains in the diluate compartment with acidic media, which is unfavorable for scaling formation.13 Therefore, less scaling on CMX-SB is well expected and corroborates the data of ICP analysis presenting smaller concentrations of Ca2+ and Mg2+ scaling ions for EDBM (pH = 5.4) in comparison with other EDBM treatments (Fig. 4b). Further, looking at ash and mineral contents after EDBM-UF at pH = 4.6 when all Ca2+ and Mg2+ ions are completely liberated from the casein micelles, one can see a drastic increase of scaling quantity (Fig. 4a and b). If EDBM-UF treatment is carried out until pH = 5.0, there is a substantial decrease in membrane scaling which becomes even more pronounced with application of PEF. In the case of EDBM-UF-PEF at pH = 5.0 the final ash content is close to the EDBM mode at pH = 5.4. Additionally, the differences in scaling between EDBM-UF (pH = 5.0) and EDBM-UF-PEF (pH = 5.0) are mainly due to the lower content of Ca2+ ions under the PEF treatment with 2 s/0.5 s lapses (Fig. 4b). This is in agreement with the study of Mikhaylin et al.16 who reported the inhibition of scaling by Ca compounds at this specific PEF mode.
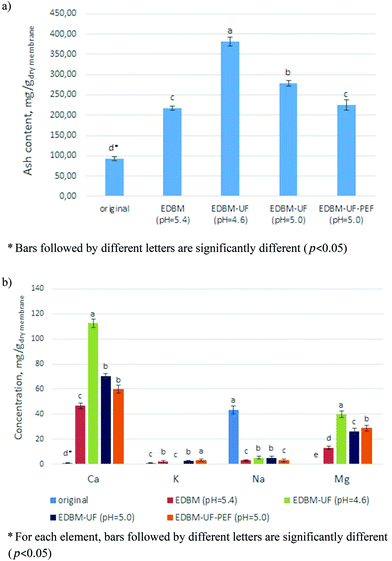 |
| Fig. 4 (a) Ash content and (b) ICP elemental analysis of original CMX-SB and CMX-SB after different EDBM treatments. | |
3.1.2.2 Scanning electron microscopy (SEM) and energy dispersive X-ray spectroscopy (EDS).
SEM and EDS images of nontreated CMX-SB (Fig. 5) showed the plane membrane surface, which does not contain any scaling ions. However, after all EDBM modes the CMX-SB surface was covered by Ca2+ and Mg2+ compounds (Fig. 5 and 6). This is in accordance with the data of ICP analysis and work of Bazinet et al.18 who reported that three types of CEM scaling after EDBM of skim milk are possible: calcium carbonate and calcium and magnesium hydroxides. The concentrate side of CMX-SB after EDBM and EDBM-UF (pH = 4.6) had a similar scaling structure comprising the scaling layer consisting of a mixture of Ca2+ and Mg2+ compounds and big agglomerates consisting of Ca2+ compounds (Fig. 5). The scaling layer and agglomerates do not correspond to the specific and well-known crystalline structure due to the influence of Mg2+ ions on the formation of Ca2+ crystals.19–22 Mg2+ ions can incorporate into the amorphous phase of calcium compounds significantly retarding its transformation into the crystalline phase or Mg2+ ions can be adsorbed onto the surface of calcite or portlandite crystals inhibiting their growth. When EDBM-UF treatment was stopped at pH 5.0, less Ca2+ ions were present in the MUF fraction in comparison with pH 4.6. This fact directly affects the scaling composition and structure. One can see the smaller peak of Ca on the EDS image and no big spherical agglomerates. Scaling for EDBM-UF (pH = 5.0) mostly consists of relatively small crystals presumably of portlandite nature.23 Application of PEF leads to the decrease in the Ca peak on the EDS. This is in accordance with the above discussed ICP analyses and work of Mikhaylin et al.16 who reported the inhibition of Ca2+ formation and growth at pulse/pause lapse 2 s/0.5 s. Additionally, on the SEM image, scaling is present in the form of an amorphous layer without big agglomerates and crystalline structures, which confirms the positive effect of PEF on the inhibition of scaling development.
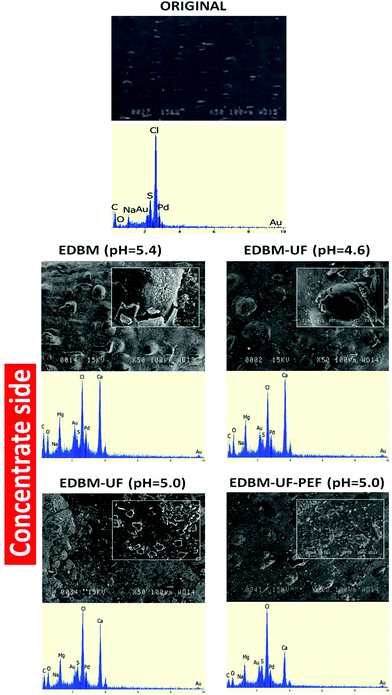 |
| Fig. 5 Scanning electron microscopy photographs and energy dispersive X-ray spectrograms of the original non-treated CMX-SB membrane and the concentrate side of the CMX-SB membrane after the different EDBM treatments. | |
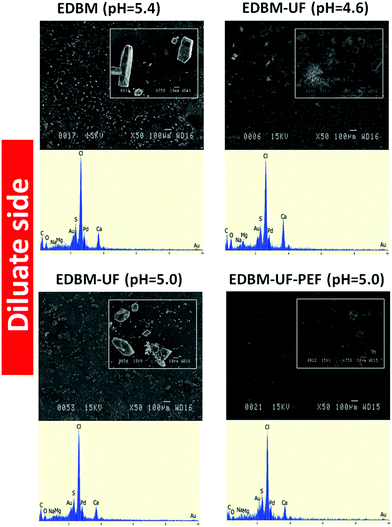 |
| Fig. 6 Scanning electron microscopy photographs and energy dispersive X-ray spectrograms of the diluate side of the CMX-SB membrane after the different EDBM treatments. | |
Concerning the diluate side directed to the acid stream, much less scaling was observed with predominance of Ca2+ compounds (Fig. 6). Indeed, acidic pH is unfavorable to the formation of Ca2+ and Mg2+ hydroxides due to the lack of OH− ions and to calcium carbonate due to a shift of the balance from carbonate ions towards hydrocarbonate ions and then towards the carbonic acid.24 However, with EDBM, EDBM-UF (pH = 5.0) and EDBM-PEF modes, the CMX-SB diluate side was covered by crystals of calcite nature and at EDBM-UF (pH = 4.6) scaling consisted of calcium carbonate crystals with the presence of amorphous calcium carbonate and/or hydroxide. The formation of calcium carbonate is induced by the leakage of OH− ions from the base compartment and the possible water splitting phenomenon.25 Hydroxyl ions from the base compartment or generated by water splitting deprotonate carbonic acid resulting in the production of carbonate ions, which are able to interact with Ca2+. For EDBM-UF (pH = 4.6) OH− leakage seems to be severe, leading to a higher scaling content on the diluate side among all EDBM modes. Severe OH− leakage is due to the high scaling content on the concentrate side, which blocked the positively charged ion-exchange sites decreasing the membrane permselectivity.26 In contrast, the EDBM-UF-PEF mode shows just traces of scaling. This fact is due to the lower scaling content observed on the concentrate side with this mode in comparison with EDBM (pH = 4.6) and to the effect of PEF, which inhibits scaling formation and decreases the concentration polarization which means a decrease of water splitting.16
3.2 Evolution of pH and global system resistance
The evolution of pH during EDBM treatment is different from all EDBM-UF treatments (Fig. 7). When milk directly passes to the EDBM stack (conventional EDBM), it is possible to see the plateau region followed by a linear decrease of pH. However, when permeate from the UF module (MUF) passes to the EDBM stack (EDBM-UF), there is no such plateau. The delay in acidification during conventional EDBM treatment is related to the relatively low flow rate of skim milk and consequently relatively low circulation of H+ produced at the bipolar membrane. Bazinet et al.8 observed the same delay phenomenon. However, these authors report the disappearance of this delay in acidification at an increased flow rate due to the better mixing of H+ and skim milk in the bulk reservoir. In the present work, application of the UF module apparently allows better mixing of acidified recirculated permeate (MUF) and retentate. Hence, pH of milk during EDBM-UF decreases right after the beginning of electroacidification and no plateau was observed. It is worth to note that the buffer capacity of skim milk retentate seems to be close to that of the initial skim milk due to the low volumetric concentration factor (1.25
:
1).27 Therefore, changes in buffer capacity, which may affect pH evolution of milk, may be neglected.
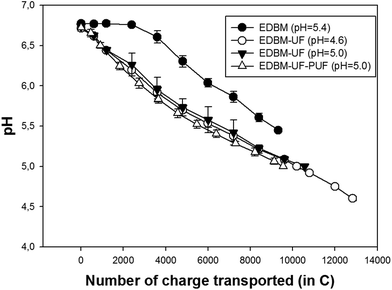 |
| Fig. 7 pH evolution during electroacidification in different EDBM modes. | |
Comparing EDBM-UF treatments, the same trends in pH evolution were observed. However, application of PEF mode seems to be advantageous in comparison with continuous current mode. Indeed, after reaching 6.5, pH decreases more readily for EDBM-UF-PEF treatment and there is a lesser amount of charge transported needed to reach the final pH value. This fact can be explained by two influences of PEF: (1) influence on BM performance and (2) influence on CEM performance. Firstly, the influence of PEF on BM performance seems to be rather minimal because H+ generation depends on the applied current and at the same amount of charge transported the same amount of H+ should be generated. However, additional investigations are needed for better comprehension of H+/OH− generation on BM under PEF. Secondly, the influence of PEF on CEM performance seems to be predominant because it is known that PEF decreases the concentration polarization17,28 and hampers membrane scaling.14,16 Both the above mentioned PEF effects prevent the migration of OH− ions into the acid compartment. A decrease in concentration polarization by PEF means a decrease in OH− generated by water-splitting on the CEM surface directed to the acid stream. Consequently, inhibition of scaling by PEF helps to maintain the high value of CEM permselectivity and to decrease the OH− leakage from the base compartment.14 Thus, the PEF mode, preventing OH− migration into the acid compartment, which leads to the neutralization of generated H+, hastens the electroacidification of MUF in comparison with continuous current mode.
The evolution of global system resistance has a similar trend at the beginning of all EDBM treatments (Fig. 8). The decrease in system resistance during electroacidification was previously explained by Bazinet et al.8 Generation of highly conductive H+ ions and migration of cations across the CEM towards the compartment where OH− ions are produced at the BM induced the overall decrease in system resistance. The following increase of system resistance is due to the presence of fouling and/or scaling.8,10 In the case of conventional EDBM, the sharp increase in system resistance after a certain number of charges was transported is due to the casein precipitation inside the spacers of the EDBM stack (Fig. 3). Comparing EDBM-UF treatments, one can observe the higher system resistance when PEF is applied. This can be connected with a better demineralization of MUF under PEF. Better demineralization means the loss of K+, which are the predominant ions electromigrating from the acid compartment at the beginning of electroacidification until a certain pH. When the concentration of K+ ions becomes too low, the migration of other cations becomes easier. However, the cation migration is not sufficient to counterbalance the generated H+ ions, which leads to the electromigration of H+ out of the acid compartment and decrease in the current efficiency of EDBM.13
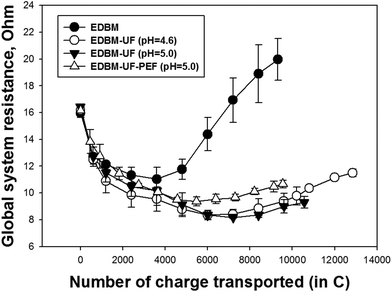 |
| Fig. 8 Global system resistance evolution during electroacidification in different EDBM modes. | |
3.3 Soluble protein content
Analysis of supernatants of the electroacidified milk samples (Table 1) shows that at pH 4.6, 22.4% of protein remain soluble. According to the literature, this protein fraction corresponds to the whey proteins, which represent around 20% of total proteins.8,29 Thus, the precipitated fraction, which is clearly visible (Fig. 9), represents caseins. These results demonstrate the viability of EDBM-UF as a method for casein precipitation by electroacidification until pH 4.6. Furthermore, Fig. 9 shows casein precipitation even at pH 5.0, which is confirmed by LECO nitrogen analysis indicating 20.0–25.7% of soluble proteins (Table 1). The complete precipitation of caseins at pH 5.0 can be explained by the retention of the mineral fraction by the UF membrane and its demineralization during EDBM treatment, which affects the stability of casein micelles. Generally, casein micelles are considered as fluffy particles with κ-casein proteins on their surface.30 This surface κ-casein is present in the form of a salted polyelectrolyte brush stabilizing casein micelles. A change in ionic strength may lead to the collapse of the polyelectrolyte brush and destabilization of casein micelles. Therefore, demineralization during EDBM decreases the ionic strength of milk solution leading to the easier destabilization of casein micelles and consequently to the shift of the isoelectric point of caseins towards more alkaline pH. This is in agreement with results obtained by Bazinet et al.31 who observed the opposite effect when the isoelectric point of caseins was shifted towards acidic pH values by an increase in milk ionic strength by salt addition.
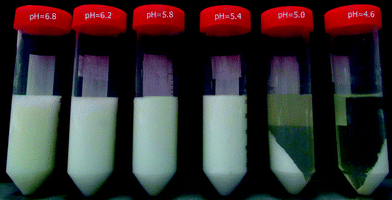 |
| Fig. 9 Skim milk after EDBM-UF treatment at different pH values. | |
Table 1 Soluble protein content under different EDBM modes (%)
Mode/pH |
6.8 |
6.2 |
5.8 |
5.4 |
5.0 |
4.6 |
Mean values at the same line followed by different letters are significantly different (p < 0.05).
|
EDBM |
99.8 ± 0.2a a |
92.8 ± 1.5b |
89.1 ± 1.3c |
72.7 ± 2.6d |
— |
— |
EDBM-UF |
99.8 ± 0.3a |
93.4 ± 0.4b |
90.5 ± 4.3b |
78.3 ± 1.9c |
25.7 ± 3.2d |
22.4 ± 0.9d |
EDBM-UF |
99.8 ± 0.2a |
92.5 ± 1.5b |
89.3 ± 8.2b |
75.3 ± 7.3c |
23.4 ± 3.6d |
— |
EDBM-UF-PEF |
99.8 ± 0.2a |
91.0 ± 2.5b |
90.1 ± 9.2b |
71.6 ± 2.8c |
20.0 ± 3.4d |
— |
4. Conclusion
The results obtained in this study demonstrate for the first time the effectiveness of a new approach for precipitation of caseins from bovine skim milk. This approach comprises application of electrodialysis with bipolar membranes coupled with the ultrafiltration module (EDBM-UF). EDBM-UF allows casein production without the use of chemicals and waste generation.
✓ The main advantage of the proposed approach is the complete inhibition of protein precipitation in the EDBM stack and at the surfaces of CEM and BM.
✓ Furthermore, it was found that complete precipitation of caseins occurred at pH 5.0, which is interesting in terms of scaling inhibition. Indeed, at pH 5.0: (1) a part of calcium and magnesium is still present in the colloidal form binding with casein micelles, which means less free Ca2+ (Mg2+) ions migrating via the base compartment and less CEM scaling, (2) a substantial part of Ca2+ (Mg2+) ions remains in the diluate solution due to the predominant migration of K+ ions. This was confirmed by results of ash content and ICP analysis.
✓ The final step in the improvement of the EDBM technique is the application of PEF (EDBM-UF-PEF). From the best of author's knowledge, the present work demonstrates for the first time the application of PEF to EDBM. Indeed, PEF hampers the formation of scaling and prevents the leakage of OH− ions from the base stream, which leads to a better performance of EDBM treatment and to a longer membrane lifetime.
Further research will focus on the improvement of the UF module in order to obtain a higher flow rate of the permeate allowing a better performance of EDBM treatment. Moreover, the addition of KCl during treatment seems to be a prospective step allowing inhibition of Ca2+ (Mg2+) migration and consecutively scaling inhibition.
5. Materials and methods
5.1 Materials
The raw material used in this study was commercial fresh pasteurized and homogenized skim milk (Quebon, Natrel, Longueuil, Canada). NaCl and KCl (ACS grade) were obtained from Laboratoire MAT (Quebec, Canada).
5.2 Methods
5.2.1 Scanning electron microscopy (SEM) and energy dispersive X-ray spectroscopy (EDS).
Images of the CEMs (dried under vacuum at 80 °C for 16 h) were taken with a scanning electron microscope JEOL (Japan Electro Optic Laboratory, model JSM840A, Peabody, Massachusetts, USA) equipped with an energy dispersive spectrometer (EDS) (Princeton Gamma Tech., Princeton, New Jersey, USA). The EDS conditions were 15 kV accelerating voltage with a 13 mm working distance. The samples were coated with a thin layer of gold/palladium in order to make them electrically conductive and to improve the quality of the microscopy photographs.14
5.2.2 Ash content.
The ash content of CMX-SB membranes was determined according to the AOAC method no. 945-46. Approximately 1.5 g of dried CMX-SB sample was added to the cooled crucibles, and the mass was recorded. The sample was then ashed at 550 °C for 16 hours and weighed again when it reached room temperature.
5.2.3 Cation concentration determination.
Magnesium, calcium, sodium and potassium concentrations were determined by using Inductively Coupled Plasma (ICP-OES, Optima 4300, Dual View, Perkin-Elmer, Shelton, CT, USA). The wavelengths used for these elements were: 285.219, 317.933, 589.592 and 766.490 nm, respectively.13 The cation analyses were carried out in radial view.
5.2.4 Soluble protein determination.
The protein concentration determination was done using an FP-428 LECO apparatus (LECO Corporation, Saint Joseph, MI). The instrument was calibrated each time with ethylenediaminetetraacetic acid (EDTA) as the nitrogen standard.8
5.2.5 Statistical analyses.
The data of soluble protein content, ash content and ICP analyses were subjected to an analysis of variance using SAS software (SAS version 9.3, 2011). LCD and Waller–Duncan post hoc tests were used.
Acknowledgements
The financial support of the Natural Sciences and Engineering Research Council of Canada (NSERC) is acknowledged. The authors want to thank Mr André Ferland from Faculté des Sciences et de Génie (Université Laval) for his technical assistance with electron microscopy.
References
- H. Korhonen and A. Pihlanto, Int. Dairy J., 2006, 16, 945–960 CrossRef CAS.
- A. Astrup and N. R. W. Geiker, Nutr. Metab. Cardiovasc. Dis., 2014, 24, 220–223 CrossRef CAS PubMed.
- H. von Bibra, G. Wulf, M. St John Sutton, A. Pfützner, T. Schuster and P. Heilmeyer, Int. J. Cardiol. Metab. Endocr., 2014, 2, 11–18 Search PubMed.
- P. M. Clifton, D. Condo and J. B. Keogh, Nutr. Metab. Cardiovas. Dis., 2014, 24, 224–235 CrossRef CAS PubMed.
-
D. L. Phillips, University of Tennessee Honors Thesis Projects, 2014 Search PubMed.
-
H. Singh, Encyclopedia of Dairy Sciences, 2011, pp. 887–893 Search PubMed.
-
D. M. Mulvihill and M. P. Ennis, in Advanced Dairy Chemistry—1 Proteins, ed. P. F. Fox and P. L. H. McSweeney, Springer, US, 2003, ch. 32, pp. 1175–1228 Search PubMed.
- L. Bazinet, F. Lamarche, D. Ippersiel and J. Amiot, J. Agric. Food Chem., 1999, 47, 5291–5296 CrossRef CAS PubMed.
- J. Balster, I. Pünt, D. F. Stamatialis, H. Lammers, A. B. Verver and M. Wessling, J. Membr. Sci., 2007, 303, 213–220 CrossRef CAS.
- M. P. Mier, R. Ibañez and I. Ortiz, Biochem. Eng. J., 2008, 40, 304–311 CrossRef CAS.
- Y. Le Graet and G. Brulé, Le Lait, 1993, 73, 51–60 CrossRef CAS.
- P. Walstra, J. Dairy Sci., 1990, 73, 1965–1979 CrossRef CAS.
- L. Bazinet, D. Ippersiel, C. Gendron, J. Beaudry, B. Mahdavi, J. Amiot and F. Lamarche, J. Membr. Sci., 2000, 173, 201–209 CrossRef CAS.
- N. Cifuentes-Araya, G. Pourcelly and L. Bazinet, J. Colloid Interface Sci., 2011, 361, 79–89 CrossRef CAS PubMed.
- B. Ruiz, P. Sistat, P. Huguet, G. Pourcelly, M. Araya-Farias and L. Bazinet, J. Membr. Sci., 2007, 287, 41–50 CrossRef CAS.
- S. Mikhaylin, V. Nikonenko, G. Pourcelly and L. Bazinet, J. Membr. Sci., 2014, 468, 389–399 CrossRef CAS.
- N. A. Mishchuk, L. K. Koopal and F. Gonzalez-Caballero, Colloids Surf., A, 2001, 176, 195–212 CrossRef CAS.
- L. Bazinet, D. Montpetit, D. Ippersiel, J. Amiot and F. Lamarche, J. Colloid Interface Sci., 2001, 237, 62–69 CrossRef CAS PubMed.
- T. Chen, A. Neville and M. Yuan, Chem. Eng. Sci., 2006, 61, 5318–5327 CrossRef CAS.
- R. A. Berner, Geochim. Cosmochim. Acta, 1975, 39, 489–504 CrossRef CAS.
- E. Loste, R. M. Wilson, R. Seshadri and F. C. Meldrum, J. Cryst. Growth, 2003, 254, 206–218 CrossRef CAS.
- Y. Zhang and R. A. Dawe, Chem. Geol., 2000, 163, 129–138 CrossRef CAS.
- C. Rodriguez-Navarro, E. Hansen and W. S. Ginell, J. Am. Ceram. Soc., 1998, 81, 3032–3034 CrossRef CAS.
-
G. Nehrke, Calcite precipitation from aqueous solution: transformation from vaterite and role of solution stoichiometry, Universität Utrecht, Niederlande, 2007 Search PubMed.
- N. Cifuentes-Araya, G. Pourcelly and L. Bazinet, J. Colloid Interface Sci., 2012, 372, 217–230 CrossRef CAS PubMed.
- M. Bleha, G. Tishchenko, V. Šumberová and V. Kůdela, Desalination, 1992, 86, 173–186 CrossRef CAS.
- S. Srilaorkul, L. Ozimek, F. Wolfe and J. Dziuba, Can. Inst. Food Sci. Technol. J., 1989, 22, 56–62 CrossRef CAS.
- V. V. Nikonenko, N. D. Pismenskaya, E. I. Belova, P. Sistat, P. Huguet, G. Pourcelly and C. Larchet, Adv. Colloid Interface Sci., 2010, 160, 101–123 CrossRef CAS PubMed.
-
J. A. O'Mahony and P. F. Fox, in Advanced Dairy Chemistry, ed. P. L. H. McSweeney and P. F. Fox, Springer, US, 2013, ch. 2, pp. 43–85 Search PubMed.
-
C. G. De Kruif and C. Holt, in Advanced Dairy Chemistry—1 Proteins, ed. P. F. Fox and P. L. H. McSweeney, Springer, US, 2003, ch. 5, pp. 233–276 Search PubMed.
- L. Bazinet, D. Ippersiel, C. Gendron, B. Mahdavi, J. Amiot and F. Lamarche, J. Dairy Res., 2001, 68, 237–250 CrossRef CAS PubMed.
|
This journal is © The Royal Society of Chemistry 2016 |
Click here to see how this site uses Cookies. View our privacy policy here.