DOI:
10.1039/C5FO00704F
(Paper)
Food Funct., 2016,
7, 270-278
Long-chain polyunsaturated fatty acids amend palmitate-induced inflammation and insulin resistance in mouse C2C12 myotubes
Received
11th June 2015
, Accepted 14th September 2015
First published on 5th October 2015
Abstract
Intramuscular lipid accumulation results in inflammation, which is correlated with impaired insulin action in the skeletal muscle, an important organ for glucose uptake in the body. In this study, we explored the effects of docosahexaenoic acid (DHA), eicosapentaenoic acid (EPA), arachidonic acid (AA), and long-chain polyunsaturated fatty acids (PUFAs) on palmitic acid (PA)-induced inflammatory responses and insulin resistance in C2C12 myotubes. The mRNA expression of the pro-inflammatory cytokines interleukin-6 and tumor necrosis factor-α in PA-treated myotubes was suppressed by these three test long-chain PUFAs. Moreover, the addition of long-chain PUFAs decreased PA-induced insulin resistance as evidenced by increases in phosphorylated AKT and glucose uptake. In PA-treated myotubes, long-chain PUFAs improved glucose transporter 4 expression, basal glucose uptake without insulin, and the AMP-activated protein kinase pathway. Of note, the long-chain PUFAs obstructed the effects of PA on the activation of extracellular-signal-regulated kinase and protein kinase C-θ as well as nuclear factor-κB (NF-κB) and activator protein-1. The inhibitory effect of AA but not of DHA and EPA on PA-induced inflammation and impaired insulin action was cancelled in C2C12 myotubes transfected with a constitutively active mutant IκB kinase-β plasmid. These data suggest that long-chain PUFAs may be useful in the management of PA-induced inflammation and insulin resistance in myotubes. In addition to the NF-κB pathway, other mechanisms are involved in the health benefits of DHA and EPA in PA-treated myotubes.
Introduction
Chronic inflammation characterized by the increased production of inflammatory mediators contributes to the pathogenesis of various diseases and metabolic disorders, including insulin resistance and type 2 diabetes.1 Excessive levels of tumor necrosis factor-α (TNF-α) and interleukin-6 (IL-6) have been found in the circulation and in skeletal muscle of insulin resistant and diabetic subjects.2,3 In addition to hepatocytes and adipocytes,4,5 treatment with palmitic acid (PA), a common dietary long-chain saturated fatty acid, dramatically induces the expression of pro-inflammatory cytokines such as TNF-α and IL-6 in skeletal myotubes, which leads to the development of insulin resistance.6,7 A marked reduction in plasma free fatty acid and muscle insulin resistance is observed in TNF-α-deficient obese mice.8 These results suggest that chronic activation of the inflammatory response is important in the etiology of insulin resistance in skeletal muscle.
Data have shown that a sustained increase in free fatty acids, especially PA, can trigger the transcriptional activity of nuclear factor-κB (NF-κB), which serves as a key mediator of the host immune and inflammatory responses.6,7 In mammalian cells, the NF-κB protein family comprises five members, namely p65 (RelA), p50/p105 (NF-κB1), p52/p100 (NF-κB2), RelB, and c-Rel, which form homodimers or heterodimers with each other. In unstimulated cells, an inhibitor protein termed IκB (α, β, or ε) noncovalently binds to NF-κB sequestered in the cytoplasm. Following specific phosphorylation of IκB by IκB kinase (IKK), IκB is ubiquitinated and degraded, which leads to the activation and translocation of NF-κB into the nucleus and the transcriptional expression of pro-inflammatory mediators.9,10 C2C12 myotubes treated with NF-κB inhibitors show decreased PA-induced TNF-α and IL-6 expression.6,7 Furthermore, the induction of NF-κB activation by transfection with IκB-α siRNA decreases insulin-stimulated AKT phosphorylation, glucose transporter 4 (GLUT4) translocation, and glucose uptake in C2C12 skeletal myotubes.11 Moreover, transfection with p65 siRNA to reduce PA-induced NF-κB activation prevents PA-induced insulin resistance in myotubes.11 Notably, the specific inhibitor of extracellular-signal-regulated kinase (ERK) and protein kinase C-θ (PKC-θ) reduces PA-induced NF-κB activation, pro-inflammatory cytokine expression, and insulin resistance in C2C12 skeletal myotubes.6,7,12
AMP-activated protein kinase (AMPK) is a fuel-sensing enzyme that acts as an important switch in the regulation of fatty acid and glucose metabolism.13 Activated AMPK increases fatty acid β-oxidation and decreases lipid accumulation through the phosphorylation and inactivation of acetyl-CoA carboxylase (ACC). Moreover, activated AMPK increases the phosphorylation of the AKT substrate of 160 kDa (AS160) as well as the expression and translocation of GLUT4 and glucose uptake in skeletal muscle.13–16 Previous data have shown that oleic acid decreases PA-induced NF-κB activation and IL-6 expression as well as insulin resistance by increasing the activation of AMPK in myotubes.17 Of note is the fact that by elevating AMPK activation, phytochemicals increase insulin-independent glucose uptake in myotubes.18–20 Taken together, these studies suggest that the activation of the AMPK signaling pathway has the potential to reverse the effect of PA on the inflammatory response and glucose uptake in myotubes.
Arachidonic acid (AA) is an n-6 long-chain polyunsaturated fatty acid (PUFA), whereas eicosapentaenoic acid (EPA) and docosahexaenoic acid (DHA) are n-3 long-chain PUFAs that are abundant in marine fish. Previous studies have shown that EPA and AA can diminish the insulin resistance induced by a high-fat diet and that DHA has a hypoglycemic effect in KK-Ay mice with genetic non-insulin-dependent diabetes mellitus.21–23 Although the mechanisms of these effects have not been clarified, AA, EPA, and DHA ameliorate the inhibitory effect of PA on basal and insulin-stimulated glucose uptake in L6 myotubes.24 Although the anti-inflammatory potency of EPA and DHA has been well documented in macrophages and adipocytes,25,26 the effect of these n-3 long-chain PUFAs on PA-induced inflammation in myotubes is not clear. Accumulating results suggest that AA may exert either anti- or pro-inflammatory effects depending on the experimental setting. AA decreases PA-induced inflammation, apoptosis, and impaired insulin secretion in pancreatic β-cells.27 The aim of this study was to assess the effects and mechanisms of the long-chain PUFAs AA, EPA, and DHA on PA-induced inflammation and insulin resistance in C2C12 myotubes.
Materials and methods
Materials
The C2C12 murine skeletal muscle cell line (BCRC number 60083) was purchased from the Bioresource Collection and Research Center (Hsinchu, Taiwan). Dulbecco's modified Eagle's medium (DMEM), Lipofectamine™ 2000, and Tri-Reagent™ were obtained from Invitrogen Corporation (Carlsbad, CA), and fetal bovine serum (FBS) and horse serum for cell culture were purchased from HyClone (Logan, UT). DHA, EPA, AA, and PA were from NuChek Prep, Inc. (Elysian, MN). Reagents for synthesizing complementary DNA were from Promega Corp. (Madison, WI), and real-time quantitative polymerase chain reaction (PCR) primers and TaqMan® Universal PCR Master Mix were from Applied Biosystems (Foster City, CA). The specific antibodies for GLUT4, IκB-α and p65 as well as for phosphorylated IKK α/β and total and phosphorylated PKC-θ were purchased from Santa Cruz Biotechnology (Santa Cruz, CA). The specific antibodies of poly-ADP ribose polymerase (PARP), total and phosphorylated of ERK1/2, AMPK, AKT and ACC, as well as phosphorylated AS160 were from Cell Signaling Technology Inc. (Beverly, MA). The biotin-labeled and unlabeled double-stranded NF-κB consensus oligonucleotides and a mutant double-stranded NF-κB oligonucleotide for the electrophoretic mobility shift assay (EMSA) were synthesized by MDBio Inc. (Taipei, Taiwan). The LightShift Chemiluminescent EMSA Kit, the SuperSignal West Pico kit, and chemiluminescence kits were from Pierce Chemical Corp. (Rockford, IL). [3H]2-Deoxyglucose, the Plasma Membrane Protein Extraction Kit, and the Great EscAPe™ SEAP Chemiluminescence Kit 2.0 were from PerkinElmer (Boston, MA), BioVision, Inc. (San Francisco, CA) and Clontech (Takara Bio Company, Mountain View, CA), respectively. All other chemicals were of the highest quality available.
Cell culture and treatment
The mouse C2C12 myoblasts were maintained in DMEM supplemented with penicillin (100 units per mL), streptomycin (100 μg mL−1), glutamine (2 mM), and 10% FBS at 37 °C under a humidified atmosphere of 5% CO2. When cells reached 80–90% confluence, the medium was switched to a differentiation medium containing DMEM and 2% horse serum, and the myoblasts were fused into myotubes after 6 days of incubation. Differentiated C2C12 myotubes were incubated in DMEM containing 2% fatty acid-free bovine serum albumin (Sigma Chemical Co.) in the presence or absence of 750 μM PA with or without 50 μM DHA, EPA and AA. Fatty acid stocks were dissolved in methanol and final concentrations were 20 μg μL−1 and 10 μg μL−1 for PA and long-chain PUFAs, respectively. Control cells were incubated in DMEM containing 2% fatty acid-free bovine serum albumin and 1% methanol vehicle.
Cell viability assay
The mitochondria-dependent reduction of 3-(4,5-dimethylthiazol-2yl)-2,5-diphenyltetrazolium bromide (MTT) was used to measure cell respiration as an indicator of cell viability.28 After treatment with fatty acids, C2C12 myotubes were incubated in DMEM containing 0.5 mg mL−1 MTT for 3 h. The medium was removed and isopropanol was added to dissolve the formazan. After centrifugation at 5000g for 5 min, 200 μL of supernatant fluid from each sample was added to 96-well plates, and the absorbance was read at 570 nm on a VersaMax Tunable Microplate Reader (Molecular Devices Corporation, Sunnyvale, CA).
Isolation of RNA and real-time reverse transcriptase-PCR (real-time RT-PCR)
Total RNA was extracted from C2C12 myotubes by using Tri-Reagent™ as described by the manufacturer. RNA extracts were suspended in RNase-free water and were frozen at −80 °C until analyzed. RNA was reverse transcribed with M-MMLV reverse transcriptase for the synthesis of complementary DNA. Complementary DNA was amplified with TaqMan® Universal PCR Master Mix primers and probes, and the reactions were measured using the StepOne System (Applied Biosystems). The primers and probes were purchased from Applied Biosystems: GLUT4 (Mm00436615_ml), IL-6 (Mm00446190_ml), TNF-α (Mm00443258_ml), and β-actin (Mm01205647_gl). Relative expression compared with the internal control β-actin was determined by using the 2−ΔΔCt method.29
Western blot
Total protein extracts were prepared by homogenizing myotubes in RIPA buffer. Plasma membrane protein extracts were prepared by using the Plasma Membrane Protein Extraction Kit following the manufacturer's instructions. Equal amounts of proteins were denatured and separated on SDS-polyacrylamide gels and then transferred to polyvinylidene difluoride membranes (New Life Science Product, Inc., Boston, MA). The blots were incubated sequentially with primary antibodies and horseradish peroxidase-conjugated secondary antibodies (Bio-Rad, Hercules, CA). Immunoreactive protein bands were developed by using the enhanced chemiluminescence kit, visualized by using a luminescent image analyzer (LAS-1000 plus, Fuji Photo Film Company, Japan), and quantified by using AlphaImager 2200 (Alpha Innotech Corp., San Leandro, CA).
Isolation of nuclear protein and EMSA
After being washed with cold PBS, C2C12 myotubes were scraped with ice-cold PBS and centrifuged. The pellets were resuspended in the hypotonic extraction buffer (10 mM HEPES, 10 mM KCl, 1 mM MgCl2, 1 mM EDTA, 0.5 mM dithiothreitol, 0.2 mM PMSF, 4 μg mL−1 leupeptin, 20 μg mL−1 aprotinin, and 0.5% NP-40) at 4 °C for 15 min and then centrifuged at 6000g for 15 min. The pellets were resuspended in 50 μL hypertonic extracted buffer (10 mM HEPES, 10 mM KCl, 1 mM MgCl2, 1 mM EDTA, 0.5 mM dithiothreitol, 0.2 mM PMSF, 4 μg mL−1 leupeptin, 20 μg mL−1 aprotinin, and 10% glycerol) and constantly shaken for 30 min at room temperature. The samples were then centrifuged at 10
000g for 15 min. The supernatant fluid containing nuclear proteins was collected and stored at −80 °C.
EMSA was performed according to our previous study.30 The LightShift Chemiluminescent EMSA Kit and synthetic biotin-labeled, double-stranded consensus oligonucleotides of NF-κB (5′-AGTTGAGGGGACTTTCCCAGGC-3′) were used to measure the effects of long-chain PUFAs on PA-induced NF-κB nuclear protein–DNA binding activity. Nuclear protein extracts in the reaction mixture containing poly(dI-dC), binding buffer, and biotin-labeled double-stranded oligonucleotides of NF-κB were incubated at room temperate for 30 min. In addition, an excess amount (100-fold molar excess) of the unlabeled double-stranded oligonucleotides and mutant double-stranded oligonucleotides of NF-κB (5′-AGTTGAGG
GACTTTCCCAGGC-3′) were used for the competition assay to confirm the specificity of binding. Nuclear protein–DNA complexes were separated from the unbound DNA probe on an 8% Tris/boric acid/EDTA-polyacrylamide gel and then transferred to nylon membranes. The membranes were treated with streptavidin–horseradish peroxidase, and the nuclear protein–DNA bands were developed by using a SuperSignal West Pico kit.
Plasmids and transient transfection
IKK-2 WT and IKK2-S177E S181E (IKK-2 SE) expression plasmids were purchased from Addgene (Cambridge, MA). The pSV-β-galactosidase control vector as well as NF-κB and AP-1-secreted embryonic alkaline phosphatase (SEAP) reporter plasmid were from Promega Co. and Dr Jaw-Ji Yang (Chung Shan Medical University, Taiwan), respectively. At 50–60% confluence, C2C12 myoblasts were used for transfection with Lipofectamine™ 2000 reagent. The transfected myoblasts were cultured in differentiation medium for 6 days and then treated as indicated in the figure legends.
Reporter gene assay
NF-κB and AP-1 transcriptional activity was determined by using the activity of the reporter enzyme SEAP by using the Great EscAPe™ SEAP chemiluminescence kit 2.0. SEAP activity was corrected on the basis of β-galactosidase activity by using the β-galactosidase enzyme assay system with reporter lysis buffer.
2-Deoxyglucose uptake assay
2-Deoxyglucose uptake measurements were carried out according to the method of Shi and Kandror with some modifications.31 Briefly, after experimental treatments, C2C12 myotubes were incubated in a serum-free medium for 4 h. Thereafter, the cells were incubated with or without 100 nM insulin in Krebs Ringer HEPES buffer without glucose (KRH (−) glucose; 121 mM NaCl, 4.9 mM KCl, 1.2 mM MgSO4, 0.33 mM CaCl2, 12 mM HEPES, pH 7.4) for 1 h. To measure glucose transport, the cells were incubated in KRH (−) glucose buffer containing 100 mM 2-deoxyglucose and 10 Ci mM−1 [3H]2-deoxyglucose for 5 min and then washed with ice-cold KRH (+) glucose buffer (121 mM NaCl, 4.9 mM KCl, 1.2 mM MgSO4, 0.33 mM CaCl2, 12 mM HEPES, 25 mM glucose, pH 7.4) to terminate the reaction. The cells were lysed in 0.1% SDS in KRH (−) glucose buffer and the radioactivity incorporated into the cells was measured by using a scintillation counter (MicroBeta, Perkin-Elmer, MA). The values of glucose uptake were corrected for nonspecific background by subtracting the radioactivity of the KRH (−) glucose buffer.
Statistical analysis
Data are expressed as means ± SD and were evaluated for statistical significance by one-way ANOVA and Tukey's multiple-range test by using the Statistical Analysis System (Cary, NC). A value of P < 0.05 was considered to be statistically significant.
Results
The long-chain PUFAs decreased PA-induced pro-inflammatory cytokine expression in C2C12 myotubes
Compared with the methanol vehicle control, treatment of C2C12 myotubes with 750 μM PA alone or with 50 μM DHA, EPA and AA did not have an adverse effect on the cell viability as assessed by mitochondrial reduction of MTT for a 24 h challenge (Table 1). Compared with the control, incubation of C2C12 myotubes with PA dramatically induced IL-6 and TNF-α mRNA expression. Co-treatment with the long-chain PUFAs, especially DHA, significantly (P < 0.05) inhibited PA-induced pro-inflammatory cytokine expression (Table 1).
Table 1 Effects of long-chain PUFAs on the MTT assay and PA-induced IL-6 and TNF-α mRNA expression in C2C12 myotubes
Treatmenta |
PA 750 μM |
50 μM |
— |
— |
DHA |
EPA |
AA |
C2C12 myotubes were treated with or without PA (750 μM) plus the vehicle control, DHA, EPA or AA (50 μM) for 16 h.
Data are the means ± SD of at least four separate experiments and are expressed as the percentage of the culture treated with PA alone. Values in the same row with different superscript letters are significantly different (P < 0.05).
|
MTTb |
100.0 ± 0.0 |
94.2 ± 1.5 |
99.5 ± 7.8 |
97.5 ± 3.6 |
97.6 ± 0.7 |
IL-6b |
3.4 ± 0.5c |
100.0 ± 0.0a |
4.3 ± 0.9c |
6.0 ± 1.3c |
25.0 ± 3.1b |
TNF-αb |
3.1 ± 1.4c |
100.0 ± 0.0a |
3.9 ± 0.8c |
7.0 ± 1.2b |
6.1 ± 0.2b |
The long-chain PUFAs impeded PA-induced activation of PKC-θ and ERK in C2C12 myotubes
Previous studies have shown that activation of PKC-θ and ERK is involved in inflammation and IR in PA-treated myotubes.6,7 To determine whether long-chain PUFAs modulated PA-induced activation of PKC-θ and ERK, we tested the phosphorylation of these kinases by western blotting. Compared with the control, PA treatment induced the phosphorylation of PKC-θ and ERK1 and this induction of phosphorylation of ERK1 and PKC-θ was significantly (P < 0.05) reduced by co-treatment with long-chain PUFAs (Fig. 1).
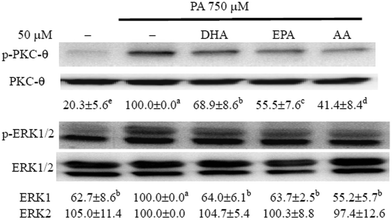 |
| Fig. 1 The long-chain PUFAs impeded PA-induced activation of PKC-θ and ERK in C2C12 myotubes. C2C12 myotubes were preincubated with 50 μM DHA, EPA or AA for 12 h and then treated with either vehicle (methanol) control or 750 μM PA for 8 h (PKC-θ activation) or 12 h (ERK activation). Cells were lysed and western blotting was performed to measure the phosphorylated and total protein expression of PKC-θ and ERK 1/2. The ratio of immunointensity between the total and phosphorylated proteins is expressed as a percentage of the culture treated with PA alone. Data are the means ± SD of four separate experiments and values not sharing the same letter are significantly different (P < 0.05). | |
The long-chain PUFAs obstructed PA-induced insulin resistance in C2C12 myotubes
The insulin-stimulated AKT phosphorylation, GLUT4 translocation and glucose uptake were assessed to study the effects of long-chain PUFAs on PA-induced insulin resistance. Insulin-enhanced AKT phosphorylation, plasma membrane GLUT4 content and glucose uptake were reduced when C2C12 myotubes were treated with 750 μM PA. Co-treatment with long-chain PUFAs canceled the effect of PA on insulin responsiveness (Fig. 2).
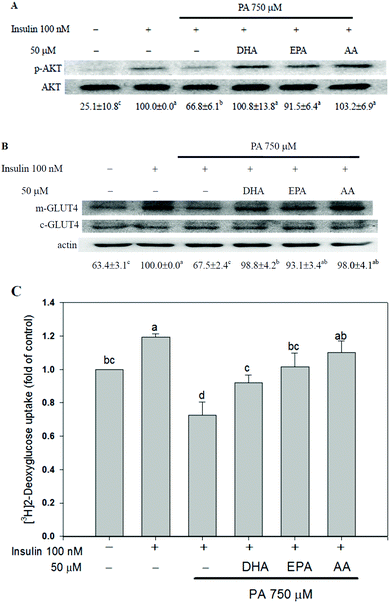 |
| Fig. 2 The long-chain PUFAs obstructed PA-induced insulin resistance in C2C12 myotubes. C2C12 myotubes were treated with or without PA (750 μM) plus the vehicle (methanol) control, DHA, EPA or AA (50 μM) for 16 h followed by stimulation with insulin (100 nM) for 15 min (AKT), 45 min (plasma membrane GLUT4 protein, m-GLUT4) or 60 min ([3H]2-deoxyglucose uptake). The protein expression of phosphorylated AKT was measured by western blotting and is expressed as a percentage of the culture treated with insulin alone after adjustment with total protein (phosphorylated AKT) or cytosolic GLUT4 (c-GLUT4) and actin (for plasma membrane GLUT4) (A and B). [3H]2-Deoxyglucose uptake was measured as described in the Materials and methods section and is expressed as fold induction over basal (non-insulin-stimulated) glucose uptake (C). Data are the means ± SD of three separate experiments and values not having the same letter are significantly different (P < 0.05). | |
The long-chain PUFAs decreased PA-induced activation of NF-κB and AP-1 in C2C12 myotubes
In C2C12 myotubes, the long-chain PUFAs abolished the PA-induced NF-κB activation as evidenced by decreases in PA-induced IκB-α degradation, NF-κB nuclear protein DNA-binding activity, and NF-κB transcriptional activity (Fig. 3). Moreover, PA-induced increase of AP-1 transcriptional activity was significantly (P < 0.05) inhibited by co-treatment with long-chain PUFAs (Fig. 3C).
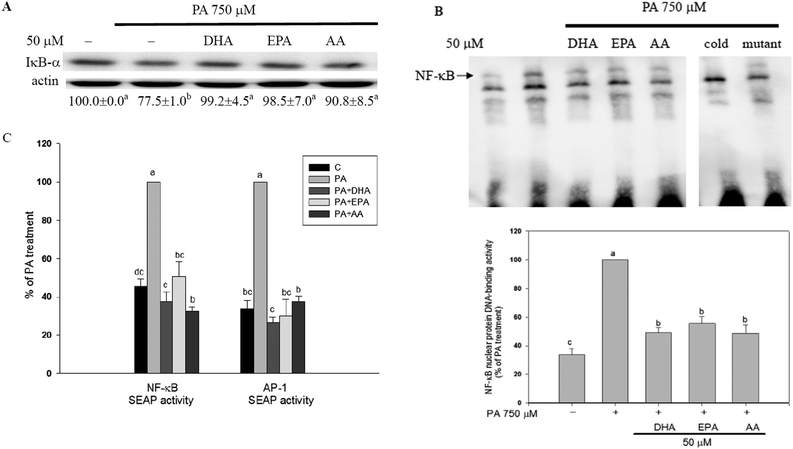 |
| Fig. 3 The long-chain PUFAs decreased PA-induced activation of NF-κB and AP-1 in C2C12 myotubes. C2C12 myotubes were preincubated with 50 μM DHA, EPA or AA for 12 h and then treated with either the vehicle (methanol) control or 750 μM PA for 12 h. Western blot analysis was used to measure the protein content of IκB-α in the cytosolic factions (A). EMSA experiments were carried out by using the LightShift Chemiluminescent EMSA kit. The unlabeled double-stranded oligonucleotides and mutant double-stranded oligonucleotides of NF-κB were added for the competition assay and the specificity assay, respectively. Bands were detected by using streptavidin–horseradish peroxidase and developed by using a SuperSignal West Pico kit (B). C2C12 myoblasts were transiently transfected with pSV-β-galactosidase as well as pNF-κB-SEAP or pAP-1-SEAP reporter gene for 24 h and then cultured in differentiation media for 6 days followed by treatment with or without PA (750 μM) plus the vehicle (methanol) control, DHA, EPA or AA (50 μM) for 16 h. The activity of the reporter enzyme SEAP was measured by using the Great EscAPe™ SEAP chemiluminescence kit 2.0 and the β-galactosidase activity was measured by using the β-Galactosidase Enzyme Assay System with Reporter Lysis Buffer from Promega Corp. (C). Data are the means ± SD of four separate experiments and are expressed as a percentage of the culture treated with the vehicle (methanol) control (for IκB-α) or PA alone (for the EMSA assay and SEPA activity) after adjustment with actin (for IκB-α) or β-galactosidase activity (for SEAP activity). Values not sharing the same letter are significantly different (P < 0.05). | |
The IKK-2 SE plasmid driving the expression of a constitutively active IKK-β (S177E, S181E) results in autophosphorylation of IKK-β and then NF-κB activation independently of upstream signaling events. When constitutively active mutant IKK-β (IKK-2 SE) plasmid was transfected into C2C12 myotubes, the inhibitory ability of the long-chain PUFAs against PA-induced increases in IKK-β phosphorylation, IκB-α degradation and nuclear p65 expression was blocked (Fig. 4A). The protective potency of AA but not EPA and DHA against PA-induced inflammation and impaired insulin action was lessened (Fig. 4B and C).
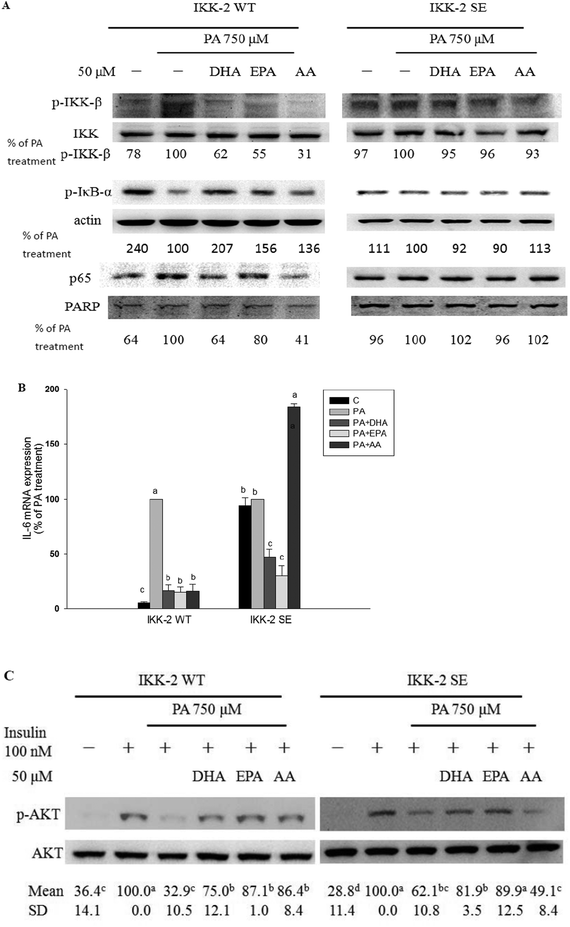 |
| Fig. 4 Effects of long-chain PUFAs on PA-induced inflammation and impairment of insulin response in C2C12 myotubes transfected with constitutively active mutant IKK-β. C2C12 myoblasts were transiently transfected with IKK-2 WT and IKK-2 SE for 24 h and then cultured in differentiation media for 6 days. C2C12 myotubes were preincubated with 50 μM DHA, EPA or AA for 12 h and then treated with either the vehicle (methanol) control or 750 μM PA for 12 h to measure levels of phosphorylated IKK-α/β, IκB-α and nuclear p65 protein. Data are expressed as a percentage of the culture treated with PA alone (A). C2C12 myotubes were treated with or without PA (750 μM) plus the vehicle (methanol) control, DHA, EPA or AA (50 μM) for 16 h to measure IL-6 mRNA expression (B). C2C12 myotubes were treated with or without PA (750 μM) plus the vehicle (methanol) control, DHA, EPA or AA (50 μM) for 16 h followed by stimulation with insulin (100 nM) for 60 min to measure insulin-stimulated phosphorylated AKT (C). The amounts of protein expression were adjusted to total protein, actin, or PARP. Data are the means ± SD of three separate experiments and are expressed as a percentage of the culture treated with PA alone or insulin alone. Within treatments with the same plasmid transfection, values not sharing the same letter are significantly different (P < 0.05). | |
The long-chain PUFAs augmented AMPK-related events and glucose uptake in PA-treated C2C12 myotubes
AMPK activation is involved in the increase of glucose uptake and fatty acid β-oxidation in skeletal muscle.14–16 Compared with the methanol vehicle control, cells treated with PA significantly (P < 0.05) decreased phosphorylation of AMPK, ACC and AS160 as well as GLUT4 mRNA expression and basal glucose uptake (without insulin treatment). In the presence of long-chain PUFAs, the inhibitory effect of the PA was annulled (Fig. 5).
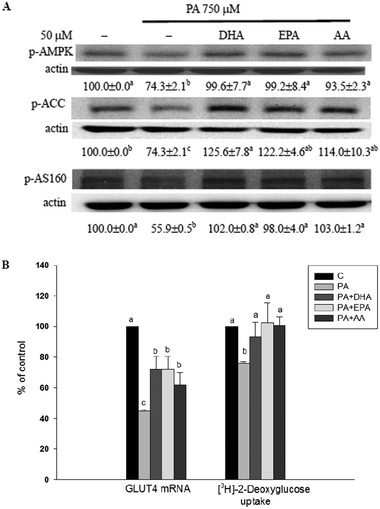 |
| Fig. 5 The long-chain PUFAs augmented AMPK-related events and basal glucose uptake in PA-treated C2C12 myotubes. C2C12 myotubes were treated with or without PA (750 μM) plus the vehicle (methanol) control, DHA, EPA or AA (50 μM) for 16 h (for phosphorylated ACC, AS160 and basal glucose uptake) and for 12 h (for phosphorylated AMPK). Western blot analysis was used to measure the protein content of phosphorylated AMPK, ACC, and AS160 adjusted to actin (A). Real-time RT-PCR was used to measure GLUT4 mRNA expression adjusted to β-actin and the [3H]2-deoxyglucose level was measured for glucose uptake (B). Data are the means ± SD of four separate experiments and are expressed as a percentage of the culture treated with the vehicle (methanol) control. Within the same test target, values not sharing the same letter are significantly different (P < 0.05). | |
Discussion
Skeletal muscle is the major target of insulin action and accounts for 80% to 90% of peripheral glucose disposal.32 It is well documented that elevated plasma free fatty acids could decrease insulin sensitivity and glucose uptake in skeletal muscle which is associated with the development of type 2 diabetes.33 Moreover, PA-induced pro-inflammatory cytokine expression is associated with the development of insulin resistance in myotubes.2,3 Previous data have shown that PA-induced pro-inflammatory cytokine expression is associated with the development of insulin resistance in myotubes.2,3 Compared with lean controls, obese and type 2 diabetic subjects have higher IL-6 levels in plasma, and these higher levels show a remarkable correlation with insulin resistance.34 Additionally, activation of the intracellular kinases related to inflammatory signaling, such as ERK and PKC-θ, is associated with insulin resistance in various experimental models including PA-treated C2C12 myotubes.6,7 Previous data from our and other laboratories have shown that the n-6 PUFAs linoleic acid, gamma linolenic acid and AA inhibit the PA-induced activation of mitogen-activated protein kinases and PKC-θ as well as impairment of glucose uptake in C2C12 myotubes.24,30,35 Our present data showed that DHA, EPA, and AA dramatically obstructed both PA-induced TNF-α and IL-6 expression as well as phosphorylation of PKC-θ and ERK in C2C12 myotubes. Moreover, these test long-chain PUFAs ameliorated the PA-induced decrease in glucose uptake in basal and insulin-stimulated C2C12 myotubes.
The transcription factor NF-κB is crucial for the induction of pro-inflammatory cytokine expression and plays an important role in the pathogenesis of diseases related to chronic inflammation.9,10 Recent data from our laboratories have shown that by inhibiting NF-κB activation, n-3 and n-6 18-carbon PUFAs can diminish PA-induced pro-inflammatory cytokine expression and insulin resistance in C2C12 myotubes.30 The data presented herein showed that the n-3 and n-6 long-chain PUFAs could effectively inhibit PA-induced activation of the NF-κB pathway which is involved in the PA-induced inflammation and insulin resistance in C2C12 myotubes.6,7,11 When we transfected cells with a constitutively active mutant IKK-β (IKK-2 SE) plasmid, the ability of the long-chain PUFAs to block NF-κB activation was impeded. In this setting, the ability of the n-6 long-chain PUFA, AA, to inhibit the effects of PA on increased IL-6 expression and decreased insulin-stimulated AKT phosphorylation was cancelled. However, the protective ability of the n-3 long-chain PUFAs, EPA and DHA, remained unchanged. These results suggest that apart from the NF-κB pathway, some other unknown mechanisms are involved in the inhibitory actions of EPA and DHA. Recent data have shown that DHA decreases PA-induced ceramide production which is associated with the inhibitory effect of PA on insulin-stimulated AKT phosphorylation.36 Our data have shown that long-chain PUFAs decrease the PA-induced transcriptional activity of AP-1, which is involved in the induction of pro-inflammatory cytokine expression in PA-treated 3T3 L1 adipocytes and hepatocytes.4,37 Further study is needed to explore the involvement of modulating ceramide production and AP-1 activation in the health benefits of n-3 long-chain PUFAs against PA-induced inflammation and insulin resistance in myotubes.
It is well established that AMPK activation is involved in the increase of glucose uptake in skeletal muscle in both the absence and presence of insulin stimulation. Activation of AMPK not only increases GLUT4 expression but also reduces the pro-inflammatory effects of PA in myotubes.12,15 Our results demonstrated that long-chain PUFAs could counteract the PA-induced insulin resistance and GLUT4 expression. Notably, supplementation with long-chain PUFAs limited the effect of PA on reducing the phosphorylation of AMPK and its downstream mediators such as ACC and AS160. It is worth studying the role of AMPK activation in the effects of long-chain PUFAs on glucose uptake and inflammation in PA-treated myotubes.
Evidence has accumulated suggesting that PA induces inflammation and insulin resistance in myotubes as a result of modulating the cell membrane function, fatty acyl-CoA metabolites, gene expression, and enzyme activity.38,39 Our data have demonstrated for the first time that both n-3 and n-6 long-chain PUFAs have potent anti-inflammatory effects in PA-treated myotubes. Moreover, the long-chain PUFAs annulled both the effect of PA on the AMPK pathway as well as insulin resistance in myotubes. Interestingly, the importance of inhibiting NF-κB activity for the effects of the long-chain PUFAs differed between the n-3 and n-6 long-chain PUFAs. Our findings have provided additional evidence supporting a beneficial role of long-chain PUFAs in lipid-induced insulin resistance.
Declarations of interest
The authors report no declarations of interest.
Acknowledgements
This research was funded by Chung Shan Medical University Hospital under grant CSH-2014-C-005. The ABI 7000 Real Time PCR System was performed in the Instrument Center of Chung Shan Medical University.
References
- K. E. Wellen and G. S. Hotamisligil, Inflammation, stress, and diabetes, J. Clin. Invest., 2005, 115, 1111–1119 CAS.
- M. Saghizadeh, J. M. Ong, W. T. Garvey, R. R. Henry and P. A. Kern, The expression of TNF α by human muscle—relationship to insulin resistance, J. Clin. Invest., 1996, 97, 1111–1116 CrossRef CAS PubMed.
- A. D. Pradhan, J. E. Manson, N. Rifai, J. E. Buring and P. M. Ridker, C-reactive protein, interleukin 6, and risk of developing type 2 diabetes mellitus, JAMA, J. Am. Med. Assoc., 2001, 286, 327–334 CrossRef CAS PubMed.
- S. Joshi-Barve, S. S. Barve, K. Amancherla, L. Gobejishvili, D. Hill and M. Cave,
et al., Palmitic acid induces production of proinflammatory cytokine interleukin-8 from hepatocytes, Hepatology, 2007, 46, 823–830 CrossRef CAS PubMed.
- K. M. Ajuwon and M. E. Spurlock, Palmitate activates the NF-kappaB transcription factor and induces IL-6 and TNFalpha expression in 3T3-L1 adipocytes, J. Nutr., 2005, 135, 1841–1846 CAS.
- M. Jove, A. Planavila, R. M. Sanchez, M. Merlos, J. C. Laguna and M. Vazquez-Carrera, Palmitate induces tumor necrosis factor-alpha expression in C2C12 skeletal muscle cells by a mechanism involving protein kinase C and nuclear factor-kappaB activation, Endocrinology, 2006, 147, 552–561 CrossRef CAS PubMed.
- M. Jove, A. Planavila, J. C. Laguna and M. Vazquez-Carrera, Palmitate-induced interleukin 6 production is mediated by protein kinase C and nuclear-factor kappaB activation and leads to glucose transporter 4 down-regulation in skeletal muscle cells, Endocrinology, 2005, 146, 3087–3095 CrossRef CAS PubMed.
- K. T. Uysal, S. M. Wiesbrock, M. W. Marino and G. S. Hotamisligil, Protection from obesity-induced insulin resistance in mice lacking TNF-alpha function, Nature, 1997, 389, 610–614 CrossRef CAS PubMed.
- F. Chen, V. Castranova, X. Shi and L. M. Demers, New insights into the role of nuclear factor-kappaB, a ubiquitous transcription factor in the initiation of diseases, Clin. Chem., 1999, 45, 7–17 CAS.
- P. J. Barnes and M. Karin, Nuclear factor–kappaB: a pivotal transcription factor in chronic inflammatory diseases, N. Engl. J. Med., 1997, 336, 1066–1071 CrossRef CAS PubMed.
- J. Zhang, W. Wu, D. Li, Y. Guo and H. Ding, Overactivation of NF-kappaB impairs insulin sensitivity and mediates palmitate-induced insulin resistance in C2C12 skeletal muscle cells, Endocrine, 2010, 37, 157–166 CrossRef CAS PubMed.
- C. J. Green, K. Macrae, S. Fogarty, D. G. Hardie, K. Sakamoto and H. S. Hundal, Counter-modulation of fatty acid-induced pro-inflammatory nuclear factor kappaB signalling in rat skeletal muscle cells by AMP-activated protein kinase, Biochem. J., 2011, 435, 463–474 CrossRef CAS PubMed.
- Y. C. Long and J. R. Zierath, AMP-activated protein kinase signaling in metabolic regulation, J. Clin. Invest., 2006, 116, 1776–1783 CrossRef CAS PubMed.
- M. D. Bruss, E. B. Arias, G. E. Lienhard and G. D. Cartee, Increased phosphorylation of Akt substrate of 160 kDa (AS160) in rat skeletal muscle in response to insulin or contractile activity, Diabetes, 2005, 54, 41–50 CrossRef CAS PubMed.
- D. Zheng, P. S. MacLean, S. C. Pohnert, J. B. Knight, A. L. Olson and W. W. Winder,
et al., Regulation of muscle GLUT-4 transcription by AMP-activated protein kinase, J. Appl. Physiol., 2001, 91, 1073–1083 CAS.
- E. J. Kurth-Kraczek, M. F. Hirshman, L. J. Goodyear and W. W. Winder, 5 -AMP-activated protein kinase activation causes GLUT4 translocation in skeletal muscle, Diabetes, 1999, 48, 1667–1671 CrossRef CAS PubMed.
- L. Salvado, T. Coll, A. M. Gomez-Foix, E. Salmeron, E. Barroso and X. Palomer,
et al. Oleate prevents saturated-fatty-acid-induced ER stress, inflammation and insulin resistance in skeletal muscle cells through an AMPK-dependent mechanism, Diabetologia, 2013, 56, 1372–1382 CrossRef CAS PubMed.
- C. E. Park, M. J. Kim, J. H. Lee, B. I. Min, H. Bae and W. Choe,
et al., Resveratrol stimulates glucose transport in C2C12 myotubes by activating AMP-activated protein kinase, Exp. Mol. Med., 2007, 39, 222–229 CrossRef CAS PubMed.
- S. Tsuda, T. Egawa, X. Ma, R. Oshima, E. Kurogi and T. Hayashi, Coffee polyphenol caffeic acid but not chlorogenic acid increases 5′AMP-activated protein kinase and insulin-independent glucose transport in rat skeletal muscle, J. Nutr. Biochem., 2012, 23, 1403–1409 CrossRef CAS PubMed.
- H. M. Eid, L. C. Martineau, A. Saleem, A. Muhammad, D. Vallerand and D. A. Benhaddou-Andaloussi,
et al., Stimulation of AMP-activated protein kinase and enhancement of basal glucose uptake in muscle cells by quercetin and quercetin glycosides, active principles of the antidiabetic medicinal plant Vaccinium vitis-idaea, Mol. Nutr. Food Res., 2010, 54, 991–1003 CAS.
- N. S. Kalupahana, K. Claycombe, S. J. Newman, T. Stewart, N. Siriwardhana and N. Matthan,
et al., Eicosapentaenoic acid prevents and reverses insulin resistance in high-fat diet-induced obese mice via modulation of adipose tissue inflammation, J. Nutr., 2010, 140, 1915–1922 CrossRef CAS PubMed.
- M. Wu, X. Wang, Q. Duan and T. Lu, Arachidonic acid can significantly prevent early insulin resistance induced by a high-fat diet, Ann. Nutr. Metab., 2007, 51, 270–276 CrossRef CAS PubMed.
- T. Shimura, T. Miura, M. Usami, E. Ishihara, K. Tanigawa and H. Ishida,
et al., Docosahexanoic acid (DHA) improved glucose and lipid metabolism in KK-Ay mice with genetic non-insulin-dependent diabetes mellitus (NIDDM), Biol. Pharm. Bull., 1997, 20, 507–510 CAS.
- K. Sawada, K. Kawabata, T. Yamashita, K. Kawasaki, N. Yamamoto and H. Ashida, Ameliorative effects of polyunsaturated fatty acids against palmitic acid-induced insulin resistance in L6 skeletal muscle cells, Lipids Health Dis., 2012, 11, 36 CrossRef CAS PubMed.
- B. Xue, Z. Yang, X. Wang and H. Shi, Omega-3 polyunsaturated fatty acids antagonize macrophage inflammation via activation of AMPK/SIRT1 pathway, PLoS One, 2012, 7, e45990 CAS.
- A. Prostek, M. Gajewska, D. Kamola and B. Bałasińska, The influence of EPA and DHA on markers of inflammation in 3T3-L1 cells at different stages of cellular maturation, Lipids Health Dis., 2014, 13, 3 CrossRef PubMed.
- D. C. Keane, H. K. Takahashi, S. Dhayal, N. G. Morgan, R. Curi and P. Newsholme, Arachidonic acid actions on functional integrity and attenuation of the negative effects of palmitic acid in a clonal pancreatic β-cell line, Clin. Sci., 2011, 120, 195–206 CrossRef CAS PubMed.
- F. Denizot and R. Lang, Rapid colorimetric assay for cell growth and survival. Modifications to the tetrazolium dye procedure giving improved sensitivity and reliability, J. Immunol. Methods, 1986, 89, 271–277 CrossRef CAS PubMed.
- K. J. Livak and T. D. Schmittgen, Analysis of relative gene expression data using real-time quantitative PCR and the 2(-Delta Delta C(T)) Method, Methods, 2001, 25, 402–408 CrossRef CAS PubMed.
- P. Y. Chen, J. Wang, Y. C. Lin, C. C. Li, C. W. Tsai and T. C. Liu,
et al., 18-Carbon polyunsaturated fatty acids ameliorate palmitate-induced inflammation and insulin resistance in mouse C2C12 myotubes, J. Nutr. Biochem., 2015, 26, 521–531 CrossRef CAS PubMed.
- J. Shi and K. V. Kandror, Study of glucose uptake in adipose cells, Methods Mol. Biol., 2008, 456, 307–315 Search PubMed.
- S. B. Biddinger and C. R. Kahn, From mice to men: insights into the insulin resistance syndromes, Annu. Rev. Physiol., 2006, 68, 123–158 CrossRef CAS PubMed.
- K. E. Wellen and G. S. Hotamisligil, Inflammation, stress, and diabetes, J. Clin. Invest., 2005, 115, 1111–1119 CAS.
- P. A. Kern, S. Ranganathan, C. L. Li, L. Wood and G. Ranganathan, Adipose tissue tumor necrosis factor and interleukin-6 expression in human obesity and insulin resistance, Am. J. Physiol.: Endocrinol. Metab., 2001, 280, E745–E751 CAS.
- A. Kadotani, Y. Tsuchiya, H. Hatakeyama, H. Katagiri and M. Kanzaki, Different impacts of saturated and unsaturated free fatty acids on COX-2 expression in C2C12 myotubes, Am. J. Physiol.: Endocrinol. Metab., 2009, 297, E1291–E1303 CrossRef CAS PubMed.
- F. Capel, C. Acquaviva, E. Pitois, B. Laillet, J. P. Rigaudière and C. Jouve,
et al., DHA at nutritional doses restores insulin sensitivity in skeletal muscle by preventing lipotoxicity and inflammation, J. Nutr. Biochem., 2015, 26, 949–959 CrossRef CAS PubMed.
- J. K. Higa and J. Panee, Bamboo extract reduces interleukin 6 (IL-6) overproduction under lipotoxic conditions through inhibiting the activation of NF-κB and AP-1 pathways, Cytokine, 2011, 55, 18–23 CrossRef CAS PubMed.
- B. Vessby, Dietary fat and insulin action in humans, Br. J. Nutr., 2000, 83(Suppl 1), S91–S96 CAS.
- U. Risérus, Fatty acids and insulin sensitivity, Curr. Opin. Clin. Nutr. Metab. Care, 2008, 11, 100–105 CrossRef PubMed.
|
This journal is © The Royal Society of Chemistry 2016 |