Performance of a composite bioactive membrane for H2 production and capture from high strength wastewater†
Received
27th April 2016
, Accepted 8th July 2016
First published on 8th July 2016
Abstract
In this study, a composite bioactive membrane was developed and tested to generate and capture hydrogen (H2) during the process of wastewater treatment. Hollow fiber membranes were coated with encapsulated acetogenic bacteria to simultaneously produce and capture H2 from waste feedstocks. Acetogens were encapsulated with cast poly(vinylalcohol) or electrospun microfibers. Under anaerobic conditions the poly(vinylalcohol) and electrospun composite membranes produced an average of 44.6 ± 11.3 mL H2 g−1 hexose (0.33 ± 0.08 mol H2 mol−1 hexose) and 21.2 ± 4.8 mL H2 g−1 hexose (0.16 ± 0.04 mol H2 mol−1 hexose), respectively, and captured 73 ± 12% and 57 ± 11%, respectively, of the total H2 produced in bioreactors fed synthetic high strength wastewater. The H2 capture efficiency of the electrospun composite membrane was improved by coating the modules with a thin film of polymeric silica gel, improving the H2 production to 28.3 ± 2.3 mL H2 per hexose (0.21 ± 0.02 mol H2 mol−1 hexose) and the H2 capture efficiency to 73 ± 15%. Final composite membranes were built by immobilizing bacteria directly onto the membrane surface, again improving H2 yields from high strength synthetic wastewater to a maximum of 48.4 ± 9.4 mL H2 g−1 hexose (0.36 ± 0.07 mol H2 mol−1 hexose) with a maximum H2 capture efficiency of 86 ± 9%. The optimized composite membranes were also capable of generating and capturing H2 from real wastewaters, with yields and capture efficiencies of 19.2 ± 3.0 mL H2 g−1 hexose (0.14 ± 0.02 mol H2 mol−1 hexose) and 99.1 ± 0.2%, and 46.0 ± 15.5 mL H2 g−1 hexose (0.34 ± 0.12 mol H2 mol−1 hexose) and 79 ± 19% when tested with a feed of sugar beet wastewater and dairy production wastewater, respectively. After further optimization, the composite membrane system could allow the extraction of high-quality energy from wastewater.
Water impact
This investigation will benefit society by decreasing wastewater treatment costs and energy use in centralized and decentralized applications. Additional benefits include the production of clean energy from marginal streams and reduction of waste strength upstream of conventional treatment trains. Reduction of chemical input, treatment energy requirements and overall carbon and energy footprint of the wastewater treatment process, are potential outcomes from the application of this technology.
|
Introduction
Despite the inherent chemical energy potential of wastewater, current wastewater treatment practices expend a considerable amount of energy to remove dissolved energy-dense compounds. Indeed, the water and wastewater treatment sectors account for 3–4% of the energy use in the United States,1 which is similar to that in other developed countries.2 A typical municipal wastewater treatment plant allots more than 50% of its total energy use to aeration,2 converting the reduced chemical energy within wastewater into CO2 and biomass. Opportunities to convert waste to energy in wastewater treatment are abundant, resulting in energy neutral or even energy generating treatment plants.3
Anaerobic digestion is used primarily to harvest energy in the form of methane biogas from high strength wastewaters. By inhibiting naturally occurring methanogens that consume hydrogen (H2) and acetate during anaerobic digestion, however, it is possible to redirect the degradation of the dissolved organic compounds in wastewater to produce H2. H2, when produced biologically, is regarded as a renewable and attractive clean energy source as a result of its high energy density and clean-burning properties. Nevertheless, technical considerations such as a need for stringent pH control and microbial competition limit the deployment of waste-to-H2 reactors.4 Previous systems designed for H2 production from wastewater have typically required pretreatment of the influent wastewater to deactivate H2-consuming methanogens. Pretreatment techniques include heating, acidifying, or autoclaving the waste,5–7 which reduces the net energy gained from the process and is not likely to be feasible at a realistic scale. The isolation and protection of H2-producing acetogens through encapsulation could provide a solution to this competition problem without the need for waste pretreatment. Many chemistries for microbial encapsulation or immobilization have been explored, including sol–gel polymers,8,9 silica gel nanoparticles,10 latex-coatings,11 and electrospun fibers,12–14 all while demonstrating cell viability. With such methods, the encapsulation of acetogens could enable the spatial control of these populations and could also separate and isolate them from methanogens. Indeed, methods of immobilizing acetogens for H2 production have been extensively studied.15 What has been missing from such studies, however, is an efficient mechanism for removing the H2 once it is produced.
Because H2 production is less favorable when the H2 partial pressure is high, removing excess H2 from the liquid phase as it is produced is critical. Some approaches to removing H2 include absorption of H2 in metals (e.g., Pd and LaNi5) or stripping H2 by boiling, recirculating a gas stream through the reactor (e.g., N2, CO2, steam), or allowing evaporation at a surface.16 These approaches, again, are all likely to result in significant operational costs at the scale required. By providing a high surface area for gas transfer, hollow fiber membranes offer a modular, energy efficient method to capture and remove H2 from water.17–20 Some studies have used hollow fiber membranes for H2 removal in acetogenic reactors,21 reducing the partial pressure of H2, and consequently improving the H2 production rate (volume of H2 per day) and H2 yields (volume of H2 per g hexose or mol H2 mol−1 hexose).22–24 To maximize H2 capture, however, it is also necessary to prevent the growth of methanogenic bacteria, which would consume H2.
In this study, simultaneous H2 production and capture from wastewater, building upon the concepts of membrane gas transfer and microbial encapsulation, is reported. Encapsulated acetogenic bacteria and hollow fiber membranes are used to create a composite membrane module wherein H2 producing bacteria are immobilized in close proximity to hollow fiber membranes that enable gas collection and removal as it is produced (Fig. 1). To our knowledge, this is the first technology that allows simultaneous and efficient production and capture of H2 from wastewater. To achieve both proof-of-concept and optimization of the technology, the composite membrane module was built using different encapsulation methods and material chemistries. The membranes were then tested in synthetic wastewater to demonstrate their potential for H2 production and capture. Further, the membranes were tested with real high strength wastewaters from dairy and sugar beet production to demonstrate the application of the technology with actual waste streams.
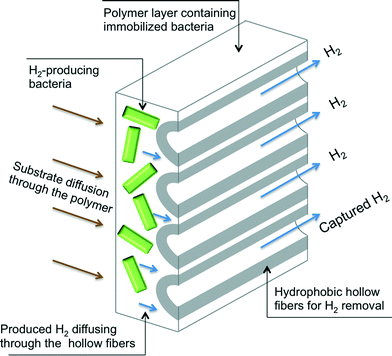 |
| Fig. 1 Conceptual schematic of the composite bioactive membrane. | |
Materials and methods
2.1. Feedstock and microbial seed
Synthetic wastewater was prepared as described in Klatt and LaPara (2003) and modified to increase chemical oxygen demand (COD) content.25
Dairy production wastewater was obtained from the permeate line of a microfiltration unit in a local dairy production plant and contained 3.72% lactate. To avoid overloading the reactors, the dairy wastewater was diluted 10-fold, to an average soluble COD of 7.8 ± 0.3 g L−1, before feeding the reactors. The sugar beet wastewater was collected from a retention pond in a local sugar beet production facility. The sugar beet wastewater had a COD of 37 g L−1 and was also diluted 10-fold before feeding.
An acetogenic seed culture was obtained by heat-treating a sample of municipal anaerobic sludge at 95 °C for 40 minutes. Serum bottles containing synthetic wastewater were inoculated with heat-treated sludge and incubated at 36 °C for 24 hours. Incubated cultures were washed with DI water twice and concentrated through centrifugation. Additional seed cultures enriched on the dairy or sugar beet wastewater were also obtained by inoculating serum bottles containing the target waste with heat-treated sludge, and further allowing them to acclimate for a period of 30 days.
2.2. Bioreactor and membrane construction
The experimental set-up consisted of a 2.75 L completely mixed anaerobic reactor containing a submerged composite membrane module (Fig. 2). The reactor was continuously fed with a peristaltic pump (Masterflex 7520-25, Cole Palmer, Vernon Hills, IL) and the hydraulic residence time was maintained at 18 h. The average influent flow rate (Q) was 2.5 ± 0.2 mL min−1. Influent and effluent pH were monitored daily and the reactor's pH was adjusted to 4.5–5.5 using NaHCO3. Influent and effluent COD were monitored 3 times per week. The membrane module was plumbed into a gas line fed by compressed ultra high purity N2 that flowed into and out of the module continuously to sweep out the biologically produced gas (e.g., H2). The gas flow rate (Qg), measured daily, was controlled manually with a gas flow meter and a needle valve and maintained at 10 mL min−1. The gas loss to the headspace (Qg-off) was measured daily using volume displacement. The composition (H2 and CH4 content) of Qg-off and the dissolved gas exiting the reactor was measured daily by taking a 5 mL sample of effluent with a gas-tight syringe. Air (1 mL) was injected to the syringe and the air/liquid mixture was shaken and allowed to equilibrate for more than 10 minutes. A 200 μL sample of the headspace was analyzed using gas chromatography with thermal conductivity detection (GC-TCD) (see below). The flow rate and composition of Qg-out was monitored daily. The system was operated at room temperature (22 ± 1.5 °C).
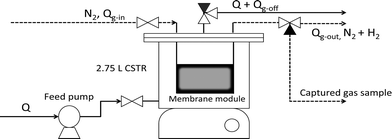 |
| Fig. 2 Schematic of the experimental set-up. Q is the influent liquid flow, Qg-off the flow rate of gas exiting the reactor, Qg-in and Qg-out is the sweep gas flow through the membranes. Note that the solid line refers to the liquid flow and the dashed is the gas flow through and out the membrane module. | |
2.2.1. Membrane construction.
Each composite membrane module consisted of a support/gas transfer layer and a bioactive layer. In all cases the support/gas transfer layer consisted of a woven mat (active area 10 cm × 7 cm) of microporous (0.3 μm pore size) hydrophobic polyethylene hollow fibers (340 μm ID, 390 μm OD, model EHF390; Mitsubishi Rayon, New York, NY). The hollow fibers were potted into a silicone tube, which acted as a manifold to distribute gas through the fibers. An example membrane module is shown in Fig. S1.† The silicone tube was plumbed into the N2 feed line or the exit gas line using plastic fittings.
2.2.1.1 Encapsulation using cast PVA.
The bioactive layer for membrane 1 (M1) modules consisted of the acetogenic seed culture cast in polyvinyl alcohol (PVA) (8.3% (w/v) aqueous solution of PVA; Elvanol 71-30 DuPont; Wilmington, DE). Concentrated microbial seed (approximately 4.7 mg in 1 mL) was mixed into 30 mL of the PVA solution, after which it was cast onto the support/gas transfer layer. The cast PVA was allowed to dry for 24 hours after which it was cross-linked in a solution of 1% (w/v) aqueous boric acid for 4 minutes. The thickness of the dry PVA coat was ~1 mm. A negative control (abiotic) membrane module was also constructed that was identical to module M1 except that the PVA layer did not contain cells.
2.2.1.2 Encapsulation using electrospun microfibers.
The bioactive layers for membrane modules 2 and 3 (M2 and M3) consisted of the acetogenic seed culture encapsulated in electrospun microfibers as described in Klein et al. (2009).13 Briefly, a core polymeric solution containing the seed culture and a shell polymer solution were co-spun using a spinneret with two coaxial capillaries. The core solution consisted of 5 wt% polyethylene oxide 600 K in water. One mL of concentrated seed (approximately 0.3 mg dry weight equivalent) was combined with 10 ml of core solution. The shell solution was 9 wt% polycaprolactone (PCL) 80 K and 1 wt% polyethylene glycol (PEG) 6 K dissolved in a mixture of chloroform and dimethylformamide, 9
:
1 (w/w). The microfibers were electrospun over the hollow fiber membrane mat for approximately 2 hours on each side, forming a uniform film with a thickness of approximately 0.2 mm.
In module M3, a third layer of silica gel was added on top of the electrospun layer. The silica gel was composed of tetraethyl orthosilicate (TEOS)-cross-linked silica nanoparticles (TM40) at a 3
:
1 ratio (v/v), as described in Mutlu et al. (2013).26 The silica gel solution was sprayed on the electrospun layers to a thickness of approximately 0.26 to 0.39 mm before gelling occurred. A negative control (abiotic) membrane module was also constructed that was identical to module M2 except that the electospun layer did not contain cells.
2.2.1.3 Encapsulation using poly(dopamine) (PDA) and a polymeric sealing coat.
An additional set of membrane modules (M4a, M4b, and M5) were created by immobilizing the acetogenic seed culture directly onto the membrane surface using PDA (H8502-25G, Sigma Aldrich, St. Louis, MO). The acetogen layer was followed by an additional polymeric layer to act as a seal to protect the organisms and prevent them from releasing back into the reactor bulk. For these three modules, a 2 g L−1 PDA solution was prepared by dissolving dopamine hydrochloride in 10 mM Tris solution (pH 8.5). The bare hollow fiber membrane mats were dip-coated with a thin film of PDA (<50 nm) to provide an adhesive surface for the cells. Defined volumes of a concentrated seed culture were then sprayed onto each side of the PDA-coated surface. After air-drying, M4a and M4b were dip-coated with silica gel. The silica gel was identical to that described in section 2.2.1.2. Module M4a contained approximately 0.2 mg of cell mass, while module M4b contained 0.4 mg of cell mass. For module M5, PVA, containing no cells but otherwise identical to that described above (section 2.2.1.1), was cast over the cells to seal the biological layer. Approximately 0.4 mg of cell mass was immobilized on module M5.
2.3. Analytical methods
Gas flow rates were monitored volumetrically using an inverted graduated cylinder and a timer. COD values were measured in diluted samples using Hach HR COD digestion vials (Hach Company, Loveland, CO). The lower detection limit was 20 mg COD L−1. Measurements of total solids (TS) were performed according to Standard Method 2540.27 Biogas composition was measured using GC-TCD (model 6890; Agilent Technologies, Santa Clara, CA) equipped with a packed column, Supelco molecular sieve 13 × 45/60, 10 ft × 1/8 in × 2.1 mm (Sigma-Aldrich, St. Louis, MO). N2 was used as carrier gas at 20 mL min−1. A gas sample was taken with a locking gas-tight syringe and 200 μL was injected for measurement.
2.4. Data analysis
The total H2 production in the reactor is expressed as: |  | (1) |
where QH-tot is the total H2 produced in the reactor, φH-off is the measured volume fraction of H2 exiting the reactor, Qg-off is the flow rate of gas exiting the reactor, φH-diss is the measured volume fraction of H2 dissolved in the effluent, Q is the effluent flow rate, kH is the specific dimensionless Henry's law constant, φH-out is the measured volume fraction of H2 captured by the membrane, and Qg-out is the flow rate of sweep gas through the membranes. All flow rates are reported in units of mL day−1.
The performance of the membrane module was evaluated based on the H2 production/capture rate (φH-outQg-out). To calculate the H2 yield (YH), the H2 production/capture rate is normalized to the sugar or hexose content in the feed wastewater (eqn (2)).
| 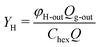 | (2) |
where
Chex is the hexose/sugar concentration of the feedstock,
Q is the feed flow rate, and
YH is the H
2 capture yield in mL g
−1 hexose (or mol H
2 mol
−1 hexose). Additionally, the H
2 capture efficiency of each module (
η) is calculated as described in
eqn (3).
|  | (3) |
Values of H2 yield from the literature were compared to the values determined here. Eqn (4) was derived from the temperature coefficient (Q10) expression28 and was used to account for temperature differences among studies in the literature.
|  | (4) |
where
YT is the observed yield,
T is the operating temperature of the study in question, and
Y22 is the estimated yield at 22 °C. For most biological processes,
Q10 values between 2 to 3 are used.
28 A value of 2.5 was used in this study.
The contribution of H2 from the mixed liquor to the membrane was calculated using eqn (5). At steady state, the flux (j, mol time−1) of H2 from the mixed liquor to the membrane lumen can be as described as follows:
| 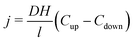 | (5) |
where
D is the diffusion coefficient of the membrane polymer (m
2 s
−1),
H (dimensionless) is the partition coefficient between the membrane and adjacent solution (
i.e., mixed liquor),
l is the membrane thickness,
Cup is the H
2 concentration upstream of the membrane (
i.e., mixed liquor), and
Cdown is the downstream H
2 concentration. For reasons described below,
Cup was assumed to be the saturation concentration of H
2 gas in water (0.8 mM). In this study, ultra pure N
2 gas was used as sweep gas, thus the concentration of H
2 downstream of the membrane (
i.e., lumen) is zero (
Cdown = 0). Therefore,
eqn (5) can be simplified as:
| 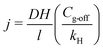 | (6) |
By assuming that the uncoated polyethylene hollow fibers were stripping H
2 from the bulk liquid saturated with H
2 gas, a “worst-case scenario” ratio of H
2 produced by the encapsulated bacteria compared to that stripped from bulk solution could be calculated. This value provides the maximum stripping that can occur in a given situation and thereby provides the most conservative value for the biologically produced H
2 within the composite membrane. For the polyethylene hollow fibers,
D and
H values were 4.74 × 10
−11 m
2 s
−1 and 1.73 × 10
−1, respectively.
29 The membrane thickness and area were 50 μm and 0.015 m
2, respectively.
Results
3.1. Proof-of-concept of the composite bioactive membrane for H2 production and capture
Results from experiments with modules M1 and M2, used to compare two different immobilization methods and polymer chemistries (i.e., cast PVA and electrospun fibers), are shown in Fig. 3 and 4. M1 was operated for more than 30 days, reaching stable operation and steady H2 production after a 4 day acclimation period. Operational variables such as influent pH and Qg-in were varied between 6.5–8.5 and 2–8 mL min−1, respectively, to identify their effect on membrane performance (Fig. 3). With influent pH held constant, a higher Qg-in of 8 mL min−1, compared to 2 mL min−1, resulted in a higher H2 yield (p < 0.05). At a constant gas flow rate, a feed pH of 8.5, compared to 6.5, also resulted in a higher H2 yield (p < 0.05). During stable operation, module M1 produced 290.1 ± 59.5 mL H2 day−1 with an average yield of 44.6 ± 11.3 mL H2 g−1 hexose (0.33 ± 0.08 mol H2 mol−1 hexose). H2 was also produced in the bioreactor and lost with the reactor's liquid effluent, with only 73 ± 12% of the produced H2 captured by the composite membrane module. Module M1 contained 4.7 mg of encapsulated bacteria, producing approximately 61.6 mL H2 d−1 mg−1 biomass.
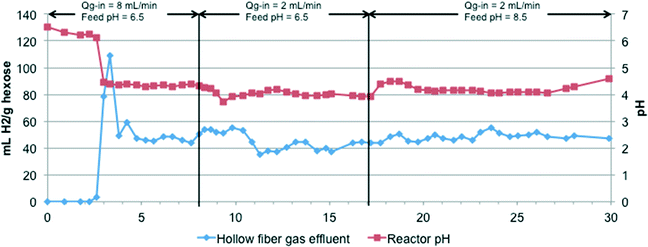 |
| Fig. 3 H2 yield and reactor pH during experiment with module M1. Changes in N2 influent flow (Qg-in) and influent feed pH are indicated at the top. | |
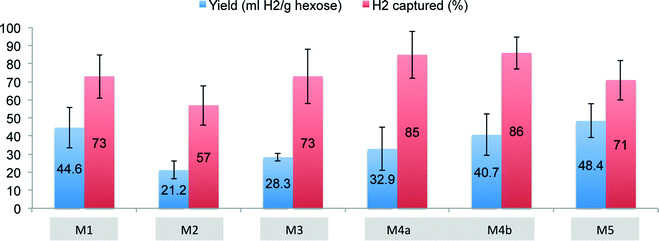 |
| Fig. 4 Summary of H2 yield and H2 capture efficiencies of the different membrane modules tested in this study. The bottom boxes indicate the different construction methods and encapsulating medium used. M1 = poly(vinyl) alcohol (PVA) + hollow fibers (HF), M2 = e-spun + HF, M3 = e-spun + HF + silica coat, M4a = HF + 1×(polydopamine (PDA) + cell coat) + silica gel seal, M4b = HF + 2×(PDA + cell coat) + silica gel seal, and M5 = HF + 2×(PDA + cell coat) + PVA seal. | |
Module M2, with the electrospun fibers, was operated for more than 30 days with Qg-in at 8–10 mL min−1. After an acclimation period of 1 week, H2 generation/capture was observed. During stable operation (DAYS 10–30) the average yield was 21.2 ± 4.8 mL H2 g−1 hexose (0.16 ± 0.04 mol H2 mol−1 hexose) with an average of 132.6 ± 29.3 mL H2 d−1 captured. This was less than that observed in module M1; nevertheless, module M2 contained only 0.3 mg of cells, 6% of the cell mass contained in module M1, suggesting that cell density is an important variable in this system. Interestingly, module M2 produced 442.1 mL H2 d−1 mg−1 biomass. This was seven times more H2 per mg biomass than observed in module M1, indicating that, despite the lower overall quantity of H2 generated over time, the electrospun system allowed for more H2 production per mg biomass, perhaps as a result of better viability post-encapsulation or better diffusion of substrate to the cells. Finally, the capture efficiency of module M2 was only 57 ± 11%, resulting in a H2 concentration of 1.0 ± 0.2% (v/v) in the module off-gas and a large fraction of the produced H2 lost to the liquid reactor effluent. Compared to module M1, the electrospun fibers in module M2 detached from the hollow fiber mat, resulting in poor contact between the encapsulated acetogens and the hollow fibers.
Experiments with modules M1 and M2 showed that H2 was lost from the system via two mechanisms: diffusion of H2 out of the module and into the reactor liquid and production of H2 outside of the bioactive composite membrane as a result of the presence of H2-producing bacteria in the bulk solution. Negative control experiments with PVA and electrospun layers did not show H2 production or capture (see Fig. S2†), clearly demonstrating the advantage of adding specific H2-producing organisms to the system. Nevertheless, these organisms were able to leak from the bioactive layer and seed the reactor, where the H2 generated would not necessarily be captured in a system treating actual waste and containing methanogens. Based on the diffusion calculation described above, modules M1 and M2 were stripping H2 from the reactor mixed liquor to some extent. Indeed, using eqn (6), about 12% and 26%, of the H2 flux coming into the lumen of modules M1 and M2 respectively, consisted of H2 stripped from the reactor bulk liquid via diffusion into the hollow fibers (Table 1). As stated above, this assumes the bulk liquid was saturated with H2, an assumption supported by observations of bubble formation in the reactor and GC measurements indicating that these bubbles were comprised primarily of H2. While not necessarily a problem with the laboratory-scale system, if scaled up and operated in this manner with unsterilized industrial waste, the H2 produced would be quickly consumed in the bulk reactor liquid reducing overall H2 production and capture.
Table 1 Estimated contribution of collected H2 from the bioactive layer versus from diffusion/stripping of the bulk liquid. The bulk liquid was assumed to be at saturation conditions (CH2aq = 0.8 mM @ 22 °C), and the calculated flow of H2 by diffusion into the membrane is 34.4 mL per day (eqn (6))
Membrane module |
Measured flow of total captured H2 (mL per day) |
% H2 flow from bioactive layer |
M1 |
PVA + HF |
290.12 |
88.1% |
M2 |
e-spun + HF |
132.62 |
74.1% |
M3 |
e-spun + HF + silica coat |
173.79 |
80.2% |
M4a |
HF + (PDA + cell coat) + silica gel seal |
198.78 |
82.7% |
M4b |
HF + 2×(PDA + cell coat) + silica gel seal |
251.57 |
86.3% |
M5 |
HF + 2×(PDA + cell coat) + PVA seal |
272.14 |
87.4% |
M5 – HRT: 18 hours |
HF + 10×(PDA + cell coat) + PVA seal |
275.81 |
87.5% |
M5 – HRT: 48 hours |
HF + 10×(PDA + cell coat) + PVA seal |
143.45 |
76.0% |
M5 – sugar beet waste |
HF + 10×(PDA + cell coat) + PVA seal |
120.91 |
100% |
M5 – dairy waste |
HF + 10×(PDA + cell coat) + PVA seal |
272.46 |
87.4% |
3.2. Optimization of the composite membrane module
To address the problems of contact, leakage of bacteria from the membrane, and production of H2 in/loss of H2 to the bulk liquid, the composite membranes were modified (module M3) by adding a silica gel sealant layer on top of the electrospun-encapsulated cells. Silica gel was used for this purpose because this material can be easily modified, offering flexibility with respect to porosity, permeability, and surface functionality, thereby maximizing the activity of encapsulated bacteria and enhancing transport of substrates into the gel.26,30 During 15 days of operation with synthetic high strength waste, the membrane modules produced 173.8 ± 10.2 mL H2 d−1 with a yield of 28.3 ± 2.3 ml H2 per hexose (0.21 ± 0.02 mol H2 mol−1 hexose). The average H2 concentration in the out-gas was still low, 1.2 ± 0.1% H2 (v/v), but the capture efficiency increased to 73 ± 15% (Fig. 4), demonstrating that the sealant layer did improve performance. Nevertheless, it appeared to be difficult to control the quantity of cells isolated in each microfiber during fabrication.
To further optimize performance, a fourth set of membranes (M4a and M4b) was created to control and increase cell density within the membranes. In these modules, cells were directly deposited onto the bare hollow fiber membranes and were encapsulated/sealed from the bulk wastewater via a layer of silica gel, as in module M3. An immediate improvement in H2 production rate, yield, and capture efficiency was observed with these modules (Fig. 4). With 0.2 mg of biomass immobilized in module M4a, 198.8 ± 71.8 ml H2 per day was produced after 3 days. The yield was also higher, at 32.9 ± 11.9 ml H2 g−1 of hexose (0.24 ± 0.09 mol H2 mol−1 hexose), and the capture efficiency increased to 85 ± 13% (Fig. 3). With double the cell density (0.4 mg), M4b produced 251.6 ± 71.4 ml H2 per day with a yield of 40.7 ± 11.4 ml H2 g−1 of hexose (0.30 ± 0.08 mol H2 mol−1 hexose), and a capture efficiency of 86 ± 9%. H2 diffusion calculations showed that the contribution of H2 from the bioactive layer increased from 83% in M4a to 86% in M4b (Table 1). Both membranes were operated for 15 days.
In spite of the silica gel's robustness in terms of material stability, when applied as a thin film, poor mechanical strength was observed.31 In wastewater treatment applications this would likely result in the loss of the acetogenic biomass over time. To overcome this problem, module M5 was constructed identically to module M4b, except that PVA was used as a sealant layer rather than silica gel. With 0.4 mg of encapsulated biomass, M5 produced 272.1 ± 37.4 mL H2 per day, with a yield of 48.4 ± 9.4 mL H2 g−1 hexose (0.36 ± 0.07 mol H2 mol−1 hexose), and a capture efficiency of 71 ± 11%. The contribution of H2 from the bioactive layer in this case was 87% (Table 1).
M5 demonstrated improved results in terms of H2 production and capture. Consequently, M5 was tested in dairy production wastewater and sugar beet wastewater to further demonstrate the applicability of the technology to actual waste streams. M5 modules used for these experiments contained biomass acclimated to dairy production wastewater and sugar beet wastewater at 2.0 mg biomass and 4.5 mg biomass, respectively. H2 production of 272.5 ± 92.0 mL H2 per day, a yield of 46.0 ± 15.5 ml H2 g−1 hexose (0.34 ± 0.12 mol H2 mol−1 hexose), and a capture efficiency of 79 ± 19% were achieved using M5 in dairy wastewater. M5 in sugar beet wastewater produced 120.9 ± 19.5 mL H2 per day, 19.1 ± 3.0 mL H2 g−1 hexose (0.14 ± 0.02 mol H2 mol−1 hexose), and captured 99.0 ± 0.2% of the total H2 produced. Both modules were operated for more than 15 days, and in the sugar beet and dairy wastewater 100% and 87% of the H2, respectively, was produced by the encapsulated bacteria based on the diffusion calculations. In the case of the sugar beet wastewater, the water in the reactor was not saturated with H2 (0.01 mM), leading to a negligible contribution from stripping.
Discussion
In addition to providing proof-of-concept data, this study reports a systematic approach to improving the physical characteristics and performance of an experimental technology, a composite bioactive membrane module. Each prototype membrane module proved effective in producing and capturing H2 from high strength wastewater. Module M5, however, demonstrated clear advantages in terms of yield, H2 capture efficiency, and the mechanical robustness of the module, resulting in a design potentially suitable for scale-up. To put this technology in context, the results of M5 were compared to other studies that used suspended cultures to produce H2 from different sources of pretreated waste (Table 2).
Table 2 Comparison of H2 yields at 22 °C. Results from similar studies adjust for temperature based on eqn (4). SS indicates sewage sludge, ADS indicates anaerobic digestion sludge, and gs indicates reactors using gas stripping
Carbohydrate substrate |
Seed type |
Reactor type |
Temp (°C) |
Yield (mL H2 g−1 hexose) |
Estimated yield at 22 °C |
Ref. |
Glucose |
SS |
CSTR |
36 |
260 |
72 |
32
|
Sucrose |
ADS |
CSTR |
35 |
148 |
45 |
33
|
Wheat starch |
ADS |
CSTR-gs |
35 |
254 |
77 |
34
|
Noodle mfg waste |
ADS |
CSTR |
35 |
200 |
61 |
35
|
Sugar beet wastewater |
ADS |
CSTR-gs |
32 |
231 |
92 |
36
|
High strength sewage surrogate |
ADS |
CSTR |
22 |
48.4 |
— |
This study – M5 |
Dairy production wastewater |
Acclimated ADS |
CSTR |
22 |
46.0 |
— |
This study – M5 |
Sugar beet wastewater |
Acclimated ADS |
CSTR |
22 |
19.1 |
— |
This study – M5 |
Estimated yields at 22 °C in similar studies from the literature, utilizing similar feedstocks, seed cultures, and reactor types, ranged from 45–92 mL H2 g−1 hexose (Table 2). Although the H2 yields in this study are within this range (48.43 ± 9.41 mL H2 g−1 hexose), the module-based technology proposed herein offers some critical advantages. First, the yield values reported in this study refer to the captured H2 that is readily available for on-site applications (e.g., cogeneration), and not to the total H2 produced in the reactors. While successfully demonstrating H2 production, previous studies have been hampered by technological limitations that result from the inability to easily capture and remove H2 from the system.34,37 Additionally, in these previous studies the need to prevent interspecies H2 transfer in non-sterile conditions requires operating with pretreated feedstock or at low pH or short retention times, lowering the net process energy balance or resulting in lower H2 yields.38 Lastly, many reactor designs for H2 production are difficult to scale-up from the laboratory to commercial and industrial scales. The proposed technology attempts to overcome these limitations by utilizing a modular system in which the energy required to supply sweep gas to the hollow fiber membrane system is significantly less than sparging39 and by utilizing encapsulated H2-producing bacteria to reduce interspecies H2 transfer and eliminate the need for feedstock pretreatment.
Laboratory studies have demonstrated that a range of industrial waste streams are feedstocks for fermentative H2 production, including noodle manufacturing waste,35 rice winery wastewater,40 filtered leachate of waste biosolids,41 sugar beet wastewater,36 palm oil mill effluent,42 and pig waste slurry.43 While using suspended growth, previous technologies were designed to build up enough biomass to provide H2 generation at low HRT. The proposed technology decouples the HRT from the SRT, providing flexibility. At an HRT of 18 hours, the optimized module M5 was able to generate 46.02 mL H2 g−1 hexose (0.34 ± 0.12 mol H2 mol−1 hexose) and 19.15 mL H2 g−1 hexose (0.14 ± 0.02 mol H2 mol−1 hexose) from dairy production and sugar beet wastewater respectively. Even though these values are less than 50% of those reported in other studies for similar substrates,36,44 they compare favorably to technologies that report the total H2 production of the bioreactor at higher concentrations of biomass.45 Indeed, based on the volume of sugar beet wastewater in Minnesota alone, this system, unoptimized, would yield approximately 2630 MW h year−1 additional electricity.
This technology is at a very early stage of development and at this time neither material cost nor module manufacture are sufficiently optimized for scale-up. Operational conditions of the reactors also play a key role in the membrane performance. For instance, CH4, CO2 and H2S are likely to be present if the buffering capacity and characteristics of the substrate provides a suitable environment of methanogenic growth. If the organic acids present in the bulk liquid are further degraded to CH4, the overall production of combustible gas will increase, resulting in a mixed CH4–H2 gas stream. Nevertheless, additional cleaning (e.g., wet scrubbing, packed columns) could be necessary to remove H2S or other impurities. In addition, the highest H2 concentration achieved in the membrane off-gas was 2.3% for M5. This would need to be improved, either via the use of vacuum gas collection, metal–organic frameworks for concentrating and storing H2,46,47 or improved H2 production in the bioactive layer. Nevertheless, the results presented with the optimized module M5 are promising and provide another technological opportunity to explore energy-neutral or energy-generating wastewater treatment.
Conclusions
Composite bioactive membrane modules were able to produce and capture H2 from high-strength synthetic and real wastewaters. This novel approach can potentially overcome many of the problems previously encountered in reactors employing fermentative H2 production. Indeed, by continuously removing H2 from the liquid phase, the H2 partial pressure was maintained below inhibitory values for the acetogenic community in this study. Furthermore, the hollow fiber membranes allowed the off gas to be easily collected, which would facilitate on-site energy generation (e.g., combined heat and power), use in fuel cells, or concentration for industrial use and storage. Although electrospun microfibers appeared to provide more surface area for the diffusion of nutrients and substrate to the encapsulated cells, the density of encapsulated cells was restricted, which in turn restricted the total quantity of H2 generated.
A multi-layer configuration (i.e., hollow fiber membranes/immobilized cells/sealant layer), together with alternative encapsulation methods (i.e., PDA-immobilized cells) showed promising results in terms of the yield and the H2 capture efficiency. Future research will focus on increasing the cell density in the bioactive layer, changing the H2 collection to improve the H2 concentration in the off-gas (i.e., via vacuum), and exploring alternative materials and manufacture protocols to improve scale-up.
Acknowledgements
This work was supported partially by the MRSEC Program of the National Science Foundation under Award Number DMR-0819885 and partially by the Environment and Natural Resources Trust Fund as recommended by the Legislative-Citizen Commission on Minnesota Resources. The authors would like to thank Goeun Heo and Julian Preciado in the Department of Mechanical Engineering at the University of Minnesota for providing the equipment and support for the preparation of the electrospun microfibers and silica coating. We would also like to thank Dr. Santiago Romero-Vargas Castrillon in the Department of Civil, Environmental, and Geo- Engineering at the University of Minnesota for his valuable insight on PDA and membrane chemistry. Competing financial interest: The authors have filed two patent applications related to this work.
References
-
US Environmental Protection Agency PA Office of Water, Wastewater Management Fact Sheet, Energy Conservation, EPA 832-F-06-024, U.S. Environmental Protection Agency, Washington DC, 2006, p. 7 Search PubMed.
-
T. P. Curtis, Low-energy wastewater treatment: strategies and technologies, In Environmental Microbiology, 2nd edn, Wiley-Blackwell, Hoboken, NJ, 2010 Search PubMed.
- H. Gao, Y. H. Scherson and G. Y. Wells, Towards energy neutral wastewater treatment: methodology and state of the art, Environ. Sci.: Processes Impacts, 2014, 16, 1223–1246 CAS.
- F. R. Hawkes, R. Dinsdale, D. L. Hawkes and I. Hussy, Sustainable fermentative hydrogen production: challenges for process optimization, Int. J. Hydrogen Energy, 2002, 27, 1339–1347 CrossRef CAS.
- C. H. Lay, J. H. Wu, C. L. Hsiao, J. J. Chang, C. C. Chen and C. Y. Lin, Biohydrogen production from soluble condensed molasses fermentation using anaerobic fermentation, Int. J. Hydrogen Energy, 2010, 35, 13445–13451 CrossRef CAS.
- K. S. Lee, P. J. Lin, J. H. Tay, K. Fangchiang and J. S. Chang, Continuous hydrogen production by anaerobic mixed microflora using a hollow-fiber microfiltration membrane bioreactor, Int. J. Hydrogen Energy, 2007, 32, 950–957 CrossRef CAS.
- Y. Sharma and B. Li, Optimizing energy harvest in wastewater treatment by combining anaerobic hydrogen producing biofermentor (HPB) and microbial fuel cell (MFC), Int. J. Hydrogen Energy, 2010, 35, 3789–3797 CrossRef CAS.
- I. Karube, T. Matsunaga, S. Tsuru and S. Suzuki, Continuous hydrogen production by immobilized whole cells of Clostridium butyricum, Biochim. Biophys. Acta, 1976, 444, 338–343 CrossRef CAS.
- I. Gill and A. Ballesteros, Bioencapsulation within synthetic polymers (Part 1): sol–gel encapsulated biologicals, Trends Biotechnol., 2000, 18(7), 282–296 CrossRef CAS PubMed.
- E. Reategui, E. Reynolds, L. Kasinkas, A. Aggarwal, M. J. Sadowsky, A. Aksan and L. P. Wackett, Silica gel-encapsulated AtzA biocatalyst for atrazine biodegradation, Appl. Microbiol. Biotechnol., 2012, 96, 231–240 CrossRef CAS PubMed.
- J. L. Gosse, M. S. Chinn, A. M. Grunden, O. I. Bernal, J. S. Jenkins, C. Yeager, S. Kosourov, M. Seibert and M. C. Flickinger, A versatile method for preparation of hydrated microbial-latex biocatalytic coatings for gas absorption and gas evolution, J. Ind. Microbiol. Biotechnol., 2012, 39, 1269–1278 CrossRef CAS PubMed.
- Y. Liu, M. H. Rafailovich, R. Malal, D. Cohn and D. Chidambaram, Engineering of bio-hybrid materials by electrospinning polymer-microbe fibers, Proc. Natl. Acad. Sci. U. S. A., 2009, 106(34), 14201–14206 CrossRef CAS PubMed.
- S. Klein, J. Kuhn, R. Avrahami, S. Tarre, M. Beliavski, M. Green and E. Zussman, Encapsulation of Bacterial Cells in Electrospun Microtubes, Biomacromolecules, 2009, 10, 1751–1756 CrossRef CAS PubMed.
- H. W. Tong, B. R. Mutlu, L. P. Wackett and A. Aksan, Manufacturing of bioreactive nanofibers for bioremediation, Biotechnol. Bioeng., 2014, 111(8), 1483–1493 CrossRef CAS PubMed.
- D. Das and T. N. Veziroǧlu, Hydrogen production by biological processes: a survey of literature, Int. J. Hydrogen Energy, 2001, 26(1), 13–28 CrossRef CAS.
- J. W. van Groenestijn, J. H. O. Hazewinkel, M. Nienoord and P. J. T. Bussmann, Energy aspects of biological hydrogen production in high rate bioreactors operated in the thermophilic temperature range, Int. J. Hydrogen Energy, 2012, 27, 1141–1147 CrossRef.
- A. Gabelman and S. T. Hwang, Hollow fiber membrane contactors, J. Membr. Sci., 1999, 159(1–2), 61–106 CrossRef CAS.
- Y. Fang, R. M. Hozalski, L. W. Clapp, P. J. Novak and M. J. Semmens, Passive dissolution of hydrogen gas into groundwater using hollow-fiber membranes, Water Res., 2002, 36, 3533–3542 CrossRef CAS PubMed.
- L. W. Clapp, M. J. Semmens, P. J. Novak and R. M. Hozalski, Model for in situ perchloroethene dechlorination via membrane-delivered hydrogen, J. Environ. Eng., 2004, 130, 1367–1381 CrossRef CAS.
- B. Lauterbock, M. Ortner, R. Haider and W. Fuchs, Counteracting ammonia inhibition in anaerobic digestion by removal with a hollow fiber membrane contactor, Water Res., 2012, 4861–4869 CrossRef CAS PubMed.
- F. R. Hawkes, I. Hussy, G. Kyazze, R. Dinsdale and D. L. Hawkes, Continuous dark fermentative hydrogen production by mesophilic microflora: Principles and progress, Int. J. Hydrogen Energy, 2007, 32, 172–184 CrossRef CAS.
- T. M. Liang, S. S. Cheng and K. L. Wu, Behavioral study on hydrogen fermentation reactor installed with silicone rubber membrane, Int. J. Hydrogen Energy, 2002, 27, 1157–1165 CrossRef CAS.
- V. V. Teplyakov, L. G. Gassanova, E. G. Sostina, E. V. Slepova, M. Modigell and A. I. Netrusov, Lab-scale bioreactor integrated with active membrane system for hydrogen production: experience and prospects, Int. J. Hydrogen Energy, 2002, 27, 1149–1155 CrossRef CAS.
- K. Bélafi-Bakó, D. Búcsú, Z. Pientka, B. Bálint, Z. Herbel, K. L. Kovács and M. Wessling, Integration of biohydrogen fermentation and gas separation processes to recover and enrich hydrogen, Int. J. Hydrogen Energy, 2006, 31, 1490–1495 CrossRef.
- C. G. Klatt and T. M. LaPara, Aerobic Biological Treatment of Synthetic Municipal Wastewater in Membrane-Coupled Bioreactors, Biotechnol. Bioeng., 2003, 82(3), 313–320 CrossRef CAS PubMed.
- B. R. Mutlu, S. Yeom, H. W. Tong, L. P. Wackett and A. Aksan, Silicon alkoxide cross-linked silica nanoparticle gels for encapsulation of bacterial biocatalysts, J. Mater. Chem. A, 2013, 1, 11051–11060 CAS.
-
APHA, AWWA and WEF, Standard methods for the examination of water and wastewater, 21st edn, 2005 Search PubMed.
- T. W. Hegarty, Temperature Coefficient (Q10), Seed Germination and Other Biological Processes, Nature, 1973, 243, 305–306 CrossRef.
-
Sandia National Laboratories, Technical reference on hydrogen compatibility on materials, SAND2012-7321, Sandia National Laboratories, Livermore CA, 2012, section 8100 Search PubMed.
- B. R. Mutlu, S. Yeom, L. R. Wackett and A. Aksan, Modeling and Optimization of a Bioremediation System Utilizing Silica Gel Encapsulated Whole-Cell Biocatalyst, Chem. Eng. J., 2015, 574–580 CrossRef CAS.
- G. Wu, J. Wang, J. Shen, T. Yang, Q. Zhang, B. Zhou, Z. Deng, F. Bin, D. Zhou and F. Zhang, Properties of sol–gel derived scratch-resistant nano-porous silica films by a mixed atmosphere treatment, J. Non-Cryst. Solids, 2000, 275(3), 169–174 CrossRef CAS.
- H. H. P. Fang, H. Liu and T. Zhang, Bio-hydrogen production from wastewater, Water Sci. Technol., 2004, 4(1), 77–85 CAS.
-
H. S. Shin, S. H. Kim and S. K. Han, Effect of substrate concentration on continuous biohydrogen production, Proc. 2nd International Workshop on Innovative Anaerobic Anaerobic Technology, Sendai, Japan, 2004, pp. 1–4 Search PubMed.
- I. Hussy, F. R. Hawkes, R. Dinsdale and D. L. Hawkes, Continuous fermentative hydrogen production from a wheat starch co-product by mixed microflora, Biotechnol. Bioeng., 2003, 84(6), 619–626 CrossRef CAS PubMed.
-
T. Noike, Biological hydrogen production of organic wastes—Development of the two-phase hydrogen production process, International Symposium on Hydrogen and Methane Fermentation of Organic Waste, Tokyo, 2002, pp. 31–39 Search PubMed.
- I. Hussy, F. R. Hawkes, R. Dinsdale and D. L. Hawkes, Continuous fermentative hydrogen production from sucrose and sugarbeet, Int. J. Hydrogen Energy, 2005, 30(5), 471–483 CrossRef CAS.
- J. J. Lay, Modeling and optimization of anaerobic digested sludge converting starch to hydrogen, Biotechnol. Bioeng., 2000, 68(3), 269–278 CrossRef CAS PubMed.
- D. Das, N. Khanna and T. N. Veziroglu, Recent developments in biological hydrogen production processes, Chem. Ind. Chem. Eng. Q., 2008, 14(2), 57–67 CrossRef CAS.
- M. Pankhania, T. Stephenson and M. J. Semmens, Hollow fibre bioreactor for wastewater treatment using bubbleless membrane aeration, Water Res., 1994, 28(10), 2233–2236 CrossRef CAS.
- H. Q. Yu, Z. H. Zhu, W. R. Hu and H. S. Zhang, Hydrogen production from rice winery wastewater in an upflow anaerobic reactor by using mixed anaerobic cultures, Int. J. Hydrogen Energy, 2002, 27(11/12), 1359–1365 CrossRef CAS.
- C. C. Wang, C. W. Chang, C. P. Chu, D. J. Lee, B. V. Chang, C. S. Liao and J. H. Tay, Using filtrate of waste biosolids to effectively produce bio-hydrogen by anaerobic fermentation, Water Res., 2003, 37(11), 2789–2793 CrossRef CAS PubMed.
- S. O-Thong, P. Prasertsan, N. Intrasungkha, S. Dhamwichukorn and N. K. Birkeland, Optimization of simultaneous thermophilic fermentative hydrogen production and COD reduction from palm oil mill effluent by Thermoanaerobacterium-rich sludge, Int. J. Hydrogen Energy, 2008, 33, 1221–1231 CrossRef CAS.
- T. A. Kotsopoulos, Biohydrogen production from pig slurry in a CSTR reactor system with mixed cultures under hyperthermophilic temperature (70 C), Biomass Bioenergy, 2009, 33(9), 1168–1174 CrossRef CAS.
- D. Karadag, O. E. Köroğlu, B. Ozkaya, M. Cakmakci, S. Heaven and C. Banks, A review on fermentative hydrogen production from dairy industry wastewater, J. Chem. Technol. Biotechnol., 2014, 89, 1627–1636 CrossRef CAS.
- C. Li and H. H. P. Fang, Fermentative hydrogen production from wastewater and solid wastes by mixed cultures, Crit. Rev. Environ. Sci. Technol., 2007, 37(1), 1–39 CrossRef CAS.
- R. Q. Snurr, J. T. Hupp and S. T. Nguyen, Prospects for Nanoporous Metal-Organic Materials in Advanced Separations Processes, AIChE J., 2004, 50, 1090–1095 CrossRef CAS.
- H. Frost, T. Düren and R. Q. Snurr, Effectsr of Surface Area, Free Volume, and Heat of Adsorption on Hydrogen Uptake in Metal-Organic Frameworks, J. Phys. Chem. B, 2006, 110, 9565–9570 CrossRef CAS PubMed.
Footnote |
† Electronic supplementary information (ESI) available. See DOI: 10.1039/c6ew00101g |
|
This journal is © The Royal Society of Chemistry 2016 |