Life cycle assessment of sodium-ion batteries†
Received
1st March 2016
, Accepted 21st March 2016
First published on 21st March 2016
Abstract
Sodium-ion batteries are emerging as potential alternatives to lithium-ion batteries. This study presents a prospective life cycle assessment for the production of a sodium-ion battery with a layered transition metal oxide as a positive electrode material and hard carbon as a negative electrode material on the battery component level. The complete and transparent inventory data are disclosed, which can easily be used as a basis for future environmental assessments. Na-ion batteries are found to be promising under environmental aspects, showing, per kWh of storage capacity, environmental impacts at the lower end of the range published for current Li-ion batteries. Still significant improvement potential is given, especially by reducing the environmental impacts associated with the hard carbon production for the anode and by reducing the nickel content in the cathode active material. For the hard carbons, the use of organic waste can be considered to be promising in this regard. Nevertheless, when looking at the energy storage capacity over lifetime, achieving a high cycle life and good charge–discharge efficiency is fundamental. This represents the main challenge especially when competing with LFP–LTO type Li-Ion batteries, which already show extraordinarily long lifetimes.
Broader context
Sodium-ion batteries (SIBs) are emerging as potential alternatives/complementaries to lithium-ion batteries (LIBs). However, no quantification of the potential environmental impacts for the production of SIBs exists. This work closes this gap presenting a prospective life cycle assessment for the production of a sodium-ion battery with a layered transition metal oxide as a positive electrode material and hard carbon as a negative electrode material on the battery component level. SIBs are found to be promising under environmental aspects, showing, per kW h of storage capacity, environmental impacts at the lower end of the range published for current LIBs. Still significant improvement potential is given, especially by reducing the environmental impacts associated with the hard carbon production and by reducing the nickel content in the cathode. For hard carbons, the use of organic waste can be considered to be promising in this regard. Regarding the energy storage capacity over lifetime, achieving a high cycle life is one of the most important parameters when aiming at providing alternatives to LIBs under environmental aspects. In the same way, the internal charge/discharge efficiency plays a key role, and achieving an efficiency only slightly above that of current LIBs can provide substantial advantages over lifetime.
|
Introduction
Na-ion batteries are emerging as potential alternatives to existing lithium based battery technologies. In theory, the maximum achievable specific energy densities of sodium-ion batteries (SIBs) are, due to the higher mass and larger ionic radius of Na+ compared to Li+, expected to be slightly lower than those of Li-ion batteries (LIB). Nevertheless, reported energy densities are already higher than those of existing lithium iron phosphate–lithium titanate (LFP–LTO) type LIBs and are expected to exceed also those of lithium iron phosphate-graphite (LFP–C) LIBs.1–3 Furthermore, SIBs make use of abundant and cheap materials (like sodium instead of lithium, aluminium instead of copper) and are expected to be associated with lower environmental impacts.4–7 This makes SIBs especially interesting for stationary energy storage systems where the weight and volume are less crucial.8,9 On the other hand, this technology is still in an early phase and no quantification of the potential environmental impacts of the production of such batteries exists. The present paper closes this gap by providing an exhaustive life cycle assessment (LCA) of a representative SIB and the comparison of its environmental performance with existing studies on LIBs.10–13 This provides support for battery developers about environmental hotspots and improvement potentials of future SIBs. Furthermore, it offers a basis for forthcoming comparisons with other post-LIB energy storage technologies like lithium–air, lithium–sulphur batteries, or even fuel cells.14–17
Methodology
Life cycle assessment (LCA)
LCA is a standardized methodology for quantifying the environmental impacts of goods, products, or activities. It takes into account the whole life cycle, from resource extraction over production, use phase until the end-of-life handling, and recycling/disposition of waste.18,19 This paper quantifies the environmental impacts associated with the production of an SIB consisting of layered oxide and hard carbon electrode materials by means of LCA in order to compare it with existing LIBs. Since the focus is on the battery production, a cradle-to-gate perspective is used, providing results independent of the latter application. The functional unit (FU), i.e., the provided service that is used as a basis for quantification/comparison, is 1 kW h of storage capacity. In order to be able to assess also the influence of the battery cycle life and internal efficiencies, where significant differences exist between battery technologies,20 a secondary FU is used, i.e., 1 kW h of lifetime energy storage capacity. The cut-off system model is used according to ecoinvent 3.2.21 Thus, the impacts associated with waste treatment or recycling processes are allocated fully to the primary process, leaving waste products available free of burden. This is consistent with previous ecoinvent versions and thus allows for comparing the results with existing LCA studies that are based on ecoinvent 2.2.22 Whenever multi-output processes are modelled, their environmental impacts are allocated to the different products according to physical relationships (mass).
For quantifying the environmental impacts, the ReCiPe midpoint method is used, applying the hierarchist perspective.23 The following impact categories are considered: fossil depletion potential (FDP), global warming potential (GWP), terrestrial acidification potential (TAP), human toxicity potential (HTP), and freshwater and marine eutrophication (FEP and MEP). The metal depletion potential (MEP), initially considered, is excluded due to a high overestimation of manganese for this category, which does not allow for drawing sound conclusions for manganese containing batteries. Nevertheless, the corresponding MEP results are disclosed in the ESI,† where also more details of the used impact categories can be found. OpenLCA software is used for implementation and impact assessment.24
Battery modelling
The Na-ion battery subject to assessment is based on a layered oxide cathode in combination with a hard carbon anode, the most extensively studied and currently most promising material combination for such batteries.3,25,26 As a composite cathode, a layered oxide in combination with an organic binder (polyvinylidene fluoride; PVdF) is used.27 The composite anode is based on a hard carbon active material produced from a carbohydrate precursor (sugar), and a water-based binder, styrene-butadiene rubber (SBR) in combination with sodium carboxymethylcellulose (CMC).28,29 For both electrodes, aluminium is used as a current collector foil for both electrodes since it does not alloy with sodium at low potentials. Sodium hexafluorophosphate (NaPF6) salt in an organic solvent is used as an electrolyte, while the separator is a conventional polyethylene/polypropylene porous sheet identical to those used in Li-ion batteries.30,31 The layout and mass balance of a battery cell are based on existing patents and technical datasheets27,30,32 (details are given in the ESI†). Numerous promising material combinations for the production of layered oxide cathodes exist,3,9,33 which is why the performance values stated for a generic layered oxide battery are used for the assessment. The SIB, which is exemplarily investigated in this work, shows a cell specific energy density of 128 W h kg−1, comparable or even slightly above that of existing LFP cells.30 Information about the achievable lifetime of SIBs is scarce due to the low technical maturity of the technology. Datasheets for pre-commercial layered oxide type batteries state at least 300 charge–discharge cycles with 80% initial capacity retention, while 2000 cycles have been proven feasible by research institutions.30,34 However, due to a similar cell setup and working principles, SIBs are in principle expected to show a comparable lifetime like of LIBs once produced with state-of-the-art, industrial equipment. The battery cells are assembled in 18
650 type cell casings, which are then packed together with the battery management system (BMS) in a steel casing to form a battery pack comparable to LIB packs. Although the materials used for the SIB might show different properties than those of LIBs, the battery cell and the pack production process are assumed to be identical, with the final cell assembly and electrolyte filling taking place under dry room conditions. Housing and BMS make up 20% of the total mass of the SIB pack,11,13,35 giving a specific energy density of 102 W h kg−1 for the final battery pack.
The manufacturing of the hard carbon anode from a carbohydrate precursor and the manufacturing of the layered oxide cathode are modelled in detail based on data disclosed in technical datasheets and patents.30,32 Cell casing, separator, battery pack housing and the BMS are assumed not to differ significantly from those of existing LIBs, thus inventory data from existing studies on the latter are used. This brings the additional advantage of better comparability with LIBs, since differences that stem from different modelling approaches of these common components are minimised. Fig. 1 depicts the production process for a complete SIB, from the material precursors until the final battery pack as modelled as a basis for the environmental assessment. The detailed life cycle inventory data (LCI) for each step of the manufacturing process and the corresponding assumptions can be found in the ESI.† Since no large-scale SIB industry is yet established, the production is assumed to be situated in Europe, using the corresponding electricity mixes.
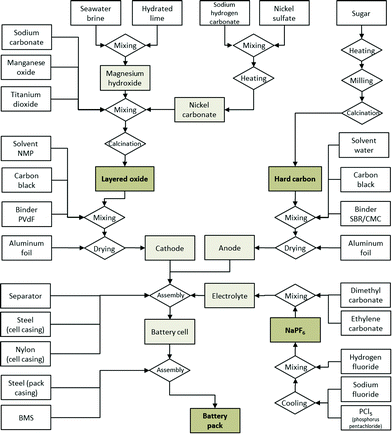 |
| Fig. 1 Flow diagram of the production process for the assessed Na-ion battery. | |
Fig. 2 shows the composition of the modelled SIB (battery cell). It is comparable to that of LIBs,36 with the anode active material making up a slightly higher share of the battery weight, which is compensated for by the use of lighter aluminium instead of copper for the current collector. The cell casing makes up a relatively high share, which is attributable to the use of 18
650 cell packaging; a pouch cell might reduce package weight substantially. The tabulated mass balance of a single 18
650 battery cell and further details of the modelling approach can be found in the ESI.†
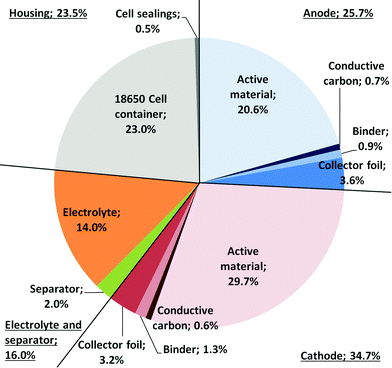 |
| Fig. 2 Composition (in wt%) of a single Na-ion battery cell. | |
Results and sensitivity analysis
Characterization results
The environmental impacts calculated for the production of the described SIB are given in Fig. 3 and discussed in the following sections.
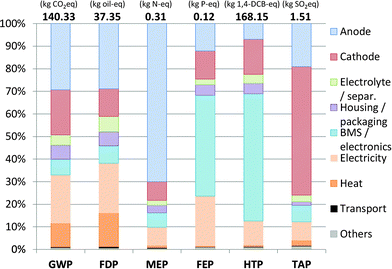 |
| Fig. 3 Characterization results for the production of 1 kW h of Na-ion battery storage capacity and contribution of the principal battery components to the overall impact per category. GWP = global warming potential, FDP = fossil depletion potential, MEP = marine eutrophication potential, FEP = freshwater eutrophication potential, HTP = human toxicity potential, TAP = terrestrial acidification potential. | |
GWP.
The anode, and here especially the production of the hard carbon active material, turns out to be an important driver for the global warming potential, the battery component being with the highest contribution to the total GWP (24%). Especially the production of the sugar from sugar beet, which is used as a precursor for the hard carbon preparation, is associated with significant GHG emissions (17% of the total GWP). The nitrogen required for maintaining an inert atmosphere in the hard carbon production process also contributes a small but notable share to the impacts (3% of the total). Apart from the hard carbon active material, the aluminium collector foil also shows relevant contributions (almost 6% of the total) from the anode. The cathode production is responsible for 20% of the total GWP, of that 9% only due to the PVdF binder, whose production is highly GHG intensive, and 3% due to the aluminium for the collector foil. Another 8% stem from the production of the layered oxide (active cathode material), and within this nickel carbonate is required as a precursor (4%). The electricity consumed during cell and battery pack manufacturing, assumed to be identical to that of existing LIBs, is also responsible for a major share (21%) of the total GWP impacts.
FDP.
The profile obtained for fossil depletion potential is similar to that for GWP. The main contributor is the production of the sugar required as a hard carbon precursor (16%) and thus the anode (29%). Production of the aluminium used for the anode and cathode collector foil is also important (7%) while the cathode production is less relevant (12%, including collector foil). Here, the nickel carbonate required for the active material is the most important driver. Other key drivers for impacts in this category are the energy demand during cell and pack manufacturing (heat: 15%, electricity: 22%), but also the BMS with 8% and the electrolyte with 6%.
HTP.
The human toxicity potential of the battery cells is comparably low: main drivers are the electronic components in the BMS, not the battery chemistry itself. BMS and wiring together make up 56% of the total impacts in this category, although the BMS is modelled based on the study by Notter et al.,11 which is already the BMS with the lowest impacts among the LCA studies on LIBs used for comparison. The remaining share comes to about equal amounts from cathode (16%; of that 10% from the nickel carbonate precursor) and anode production (7%; of that 5% from the hard carbon production, with the main driver again being the sugar precursor). The electricity required during battery pack and cell manufacturing contributes another 11%.
MEP.
For the marine eutrophication potential, the sugar as a hard carbon precursor is the major single contributor (63%). Therefore, 67% of the total impacts stem from the anode, and only 8% from the cathode, again with the nickel carbonate as the main driver for impacts (4%). Other important contributors are the electricity for battery pack and cell manufacturing (8%) and the production of the BMS (7%).
FEP.
For freshwater eutrophication, a similar picture as for HTP is obtained, with the electronic components of the BMS being the main driver for impacts in this category (45%). Here, especially the production of the gold required for integrated circuits is relevant (18% of the total). Anode and cathode production contribute about equal shares (12% each), with the main contributors being again the hard carbon precursor (10%) and the nickel carbonate production (9%). Electricity generation for manufacturing makes up another 22%.
TAP.
Acidification is clearly dominated by the production of the cathode (57%). Again, the nickel sulphate required as a precursor for the nickel carbonate production is the main single driver for the corresponding impacts, contributing alone around 53% of the total acidification potential of the whole battery pack. Other relevant contributors are the production of the sugar used as a precursor for hard carbon synthesis (13%), the BMS (7%), and the generation of the electricity for cell and battery pack manufacturing (8%; mainly due to the still high share of coal power plants in the European electricity mix).
Comparison with Li-ion technology
In order to obtain a better understanding of the potentials of the assessed SIB, the above results are compared with those of existing studies on the environmental impacts of state-of-the-art LIBs. Several studies exist on LIBs, but these use different life cycle impact assessment (LCIA) methodologies, different LCI databases and very different assumptions regarding the energy intensity of battery cell manufacturing.20,37 Therefore, the inventory data for the principal LCA studies on LIBs are recompiled and the manufacturing energy demand and electricity mix values used in different studies are homogenized, using the same average value in all cases (more details in the ESI†). This increases the comparability of the studies, putting them on a common base regarding these parameters and, above all, using the same LCIA methodology. Nevertheless, the different studies model also the other key components like the BMS or the binder used for the cathode and anode production in very different ways, and thus the direct comparability of the results is very limited. For example, Bauer10 uses simple proxies for many battery components (tetrafluoroethylene instead of PVdF, benzene instead of carbon black, generic organic chemicals for the electrolyte solvents, etc.), which increases uncertainties and might affect the impacts significantly. Still, a value range is obtained in this way for the potential impacts of LIB production that allows for benchmarking. Fig. 4 shows the comparison of the relative impacts obtained for the SIB with those of common LIBs on energy density basis (1 kW h of storage capacity).
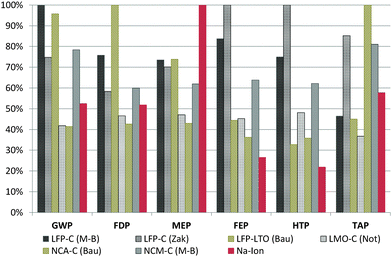 |
| Fig. 4 Relative contribution to environmental impacts per kW h of storage capacity in each assessed category. LFP = lithium iron phosphate, LTO = lithium titanate, LMO = lithium manganese oxide spinel, NCA = layered lithium nickel cobalt aluminium oxide, NCM = layered lithium nickel cobalt manganese oxide, C = graphite. M-B = inventory data from Majeau-Bettez et al.,13 Zak = inventory data from Zackrisson et al.,12 Bau = inventory data from Bauer,10 Not = inventory data from Notter et al.11 Impact categories are the same as in Fig. 3. | |
Based on the energy capacity (1 kW h of storage capacity), the assessed SIB shows promising results, taking into account that the battery chemistry is on a significantly lower technical development level than commercially available LIBs. Impacts obtained for GWP and FDP are at the lower end of the range set up by existing LIBs, while for FEP and HTP the SIB outscores all LIBs. The positive results obtained under toxicity and freshwater eutrophication aspects are mainly due to the use of aluminium instead of copper as the current collector in the anode, with copper being a very critical substance under these aspects.
Only for MEP, the SIB shows the highest impacts among all compared battery types. This is attributable to the use of sugar as an anode material precursor and might easily be improved by selecting a different type of precursor, as assessed later in the sensitivity analysis.
The LFP–C battery studied by Zackrisson et al.12 has to be mentioned explicitly in this context, since it shows extremely high values in some categories. Zackrisson et al. account for extraordinarily high amounts of electronic parts in their battery. This increases sharply the metal depletion, toxicity and eutrophication impacts, mainly due to the high amounts of gold, copper and other precious metals required for microelectronics. Bauer,10 on the other hand, uses simplified proxies for many materials and Notter et al.11 assume a water based binder for both the anode and the cathode, which leads to comparably favourable results in their assessments.
Influence of the cycle life
Battery lifetime is a key factor for environmental impacts of batteries.20,38 Since SIBs are still in a very early development phase, it is difficult to predict the stability achievable on medium or even long term. Nevertheless, 2000 cycles have already been reported for a 18
650 type prototype cell and thus seem to be feasible even on short term.34 The influence of different cycle lives on the overall impact associated with the battery production over its lifetime is assessed in comparison with LIBs, where more reliable information is available in this regard.39 The cycle lives at 80% depth of discharge (DoD) for the Li-ion batteries are average values from technical specifications, and, if not available, from existing LCA studies: LFP–C: 2960 cycles; LFP–LTO: 13
850 cycles; LMO–C: 1070 cycles; NCA–C: 2200 cycles and NCM–C: 1650 cycles.20 The environmental impacts associated with the storage of 1 kW h of electricity over lifetime are shown in Fig. 5 (without considering the electricity generation, since the objective is battery comparison). The importance of cycle life is clearly visible, e.g. for the LMO–C type LIB: while achieving very good results per kW h of storage capacity (Fig. 4), its low cycle life converts it into the worst scoring LIB type when considering the lifetime storage capacity. Compared to the LIBs, the SIB scores slightly worse in the majority of the assessed categories when assuming a lifetime of only 1000 cycles, while with 2000 cycles it already gets into the range of existing LIBs. With 3000 cycles it clearly outperforms the assessed LIBs in all the assessed categories, except the LFP–LTO, which shows extraordinarily high lifetimes.10,20 Still, per kW h of storage capacity the impacts of the SIB are lower than those of the LFP–LTO (Fig. 5), and with a similar lifetime the SIB would offer better results also compared to this LIB type. As a consequence, achieving a high cycle life represents one of the main challenges for SIBs in order to excel in existing LIB technologies under environmental aspects.
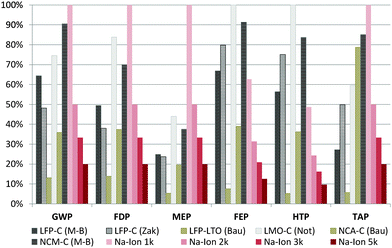 |
| Fig. 5 Influence of SIB cycle life on the environmental impacts per kW h of energy stored over lifetime. The number (1k/2k/3k/5k) indicates the assumed cycle life of the SIB: 1000/2000/3000/5000 cycles with 80% capacity retention. Remaining abbreviations and impact categories are the same as in Fig. 3. | |
Influence of the charge/discharge efficiency
The internal efficiency of batteries varies and has a relevant influence on their life cycle environmental impacts.12,20 When also considering the internal efficiency of the batteries, the use phase has to be included. This can be done in a simplified way by only accounting the additional energy required per amount of energy stored due to internal losses. A life expectancy (i.e., capacity retention higher than 80%) of 2000 charge/discharge cycles at 80% DoD and an internal efficiency of 90% are assumed for this purpose. Fig. 6 shows the influence of varying cell energy efficiency on the overall impact over lifetime (i.e., the share of environmental impacts over lifetime only due to charge–discharge losses), using the European electricity mix.21 The electricity consumed over battery lifetime due to cell energy efficiency is responsible for around 38% of the impacts caused for GWP, FDP and FEP. For HTP, it makes up roughly 23%, and for MEP and TAP around 19% of the lifetime environmental impacts. Thus, especially for the categories GWP, FDP and FEP a high sensitivity on the cell energy efficiency is given. The efficiency increase of 2%, for example from 90% to 92%, leads to a reduction of the overall impacts in these categories by about 7%. Naturally, this effect depends also on the used electricity mix and the lifetime of the battery and is more pronounced for long cycle lives. The results demonstrate the importance of the energy efficiency of batteries, which should be more explicitly considered as an important research target for battery development.
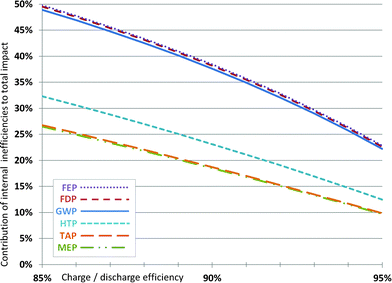 |
| Fig. 6 Contribution of charge–discharge efficiency to the environmental impact per kW h of electricity stored over battery lifetime for varying battery efficiencies (2000 cycles at 80% DoD). Impact categories are the same as in Fig. 3. | |
Influence of anode hard carbon precursors
Since hard carbon (HC) production, especially that of the sugar precursor, shows high environmental impacts in the majority of the assessed categories, different possible HC precursors are screened: starch, cellulose, organic waste and petroleum coke instead of sugar. Starch and cellulose are alternative carbohydrate precursors, organic waste is used as a generic representative for organic residues like nutshells or fruit peels/wastes,40,41 and petroleum coke as a fossil precursor.26 The characterisation results relative to the base case (HC from sugar) are given in Fig. 7. More details of the modelling and the corresponding LCI can be found in the ESI.†
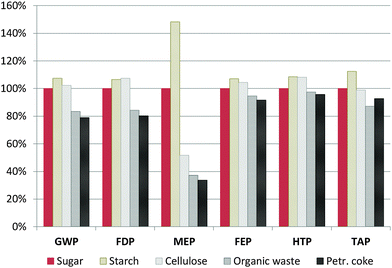 |
| Fig. 7 Influence of the hard carbon precursor on the total environmental impacts associated with SIB production, relative to the base case (sugar precursor). Impact categories are the same as in Fig. 3. | |
The use of starch or cellulose instead of sugar as the HC precursor slightly increases environmental impacts in the majority of the assessed categories, except MEP, where cellulose gives significantly better results. On the other hand, processing organic waste materials shows noteworthy improvement potential, reducing the overall impacts caused by battery production in all categories (for example, by 16% for GWP, 15% for FDP and up to 62% for MEP). High reduction of impacts is achieved especially for MEP, the only category where the SIB showed significantly worse results than the LIBs, basically due to the impacts associated with the sugar beet cultivation. Thus, the use of residues or by-products like banana peels, shaddock peels or apple wastes40,41 could be a promising option for reducing the impacts associated with the HC anode and with that the whole battery. Finally, the use of a fossil precursor, petroleum coke, also shows very favourable results, basically because of the much lower amounts of raw material required (a demand of 1.14 kg of coke is estimated per kg of HC compared to 20 kg of carbohydrate; for more details see the ESI†). Nevertheless, while this analysis can show up the tendencies, its limitations have to be taken into account: (i) the HC production process (and the yields), but also the upstream processes could vary significantly depending on the type of residue used; (ii) the precursor might have significant influence on the final electrochemical properties of the HC, and (iii) for by-products like petroleum coke or residues, the methodology used for allocating the environmental burdens of the main process and the upstream processes can affect the results considerably. Especially for the petroleum coke, the latter can be expected to increase in future, e.g. for growing shares of unconventional oils from tar sands. Thus, a separate exhaustive study of the environmental impacts of HC production would be highly recommendable.
Future improvement potentials
SIBs show some important environmental advantages compared to existing LIB technologies. The possibility to use aluminium both for the anode and the cathode avoids the need for copper, with the latter being one of the main drivers for the impacts caused by the production of LIBs. On the other hand, hard carbon is needed as an anode material, whose production from sugar is associated with relatively high impacts, partially neutralizing the benefit of the avoided copper. Thus, a hard carbon with low impacts should be favoured, for example from organic waste materials.
The production of the cathode material is also relevant for the overall environmental performance. Especially the need for nickel shows high associated impacts in several impact categories, which stem from nickel mining and nickel sulphate production as precursors for nickel carbonate. Reducing or eliminating the nickel content in the cathode active material should therefore be another objective for reducing impacts. Nevertheless, changing the cathode material composition requires a more in-detail study, since it would change the electrochemical performance of the battery as well.
Energy (electricity and heat) demand for SIB manufacturing, assumed to be identical to LIBs, is a third important driver for environmental impacts. Improving the energy efficiency of the manufacturing process, strongly driven by the dry room needed for cell assembly,3 could decrease the environmental impacts for both SIBs and LIBs.
Another critical factor, especially under GWP aspects, is the binder used for electrode production. PVdF is used as a binder for the cathode, which is also the dominating one in LIBs.35,42 Its production is associated with very high emissions of GHG gases and thus an important contributor to the GWP. In consequence, the use of alternative, water based binders also for the cathode could further reduce GHG emissions,11 both for SIBs and LIBs (more details in the ESI†).
Finally, the minimization of the required amount of electronic components (cables, BMS) could improve the environmental performance of SIBs, these also being important contributors to the overall impacts. Again, this applies in the same way to LIBs.
Conclusion
Based on the energy capacity (1 kW h of storage capacity), and with an assumed cycle life of 2000 cycles, the assessed SIB shows promising results already at the lower end of those of existing LIBs. It can be assumed that optimization potential is still given and thus a better performance can be achieved, especially by carefully selecting the cathode composition taking into account the environmental aspects and by reducing the impacts associated with the hard carbon. Nevertheless, the cycle life is the key for a good performance in this regard, and thus increasing the cycle life is one of the first parameters that should be focused on when aiming at providing alternatives to Li-ion batteries under environmental aspects. With lifetimes of around 3000 cycles, the assessed SIB would already outperform existing LIBs under environmental aspects, except for LFP–LTO type LIBs. In the same way, the internal charge/discharge efficiency plays a key role, and achieving an efficiency only slightly above that of current LIBs can provide substantial advantages over lifetime.
When taking into account the low technical maturity of SIBs, the improvements that can be made in comparison to existing LIBs are promising. Still, they are not fundamental, and thus economic advantages are also required for their future success. The cost of LIBs is partially driven by the high cost of raw materials, which could probably limit their application on a large-scale in energy storage. The use of more abundant raw materials in SIBs will bring advantages in this regard. A full economic assessment will be required to comprehensively demonstrate the possible advantages of Na-ion batteries under this perspective.
Furthermore, the used impact assessment methodology fails to quantify the (metal) resource depletion in a satisfactory way, considered to be one of the strengths of SIBs. A dedicated study of these aspects would provide further insights into the future potentials of SIBs.
Abbreviations
Battery chemistries
C | Carbon (graphite for battery electrodes/anodes) |
HC | Hard carbon |
LFP | Lithium iron phosphate |
LMO | Lithium manganese oxide |
NCA | Lithium nickel cobalt aluminium oxide |
NCM | Lithium nickel cobalt manganese oxide |
NMMT | Sodium nickel manganese magnesium titanium oxide |
LTO | Lithium titanate |
Environmental impact categories
CED | Cumulative energy demand |
CEDnr | Cumulative non-renewable energy demand |
FDP | Fossil depletion potential |
FEP | Freshwater eutrophication potential |
FETP | Freshwater eco-toxicity potential |
GWP | Global warming potential |
HTP | Human toxicity potential |
MDP | Metal depletion potential |
MEP | Marine eutrophication potential |
METP | Marine eco-toxicity potential |
ODP | Ozone depletion potential |
PMF | Particulate matter formation |
POF | Photochemical ozone formation |
TAP | Terrestrial acidification potentialv |
TETP | Terrestrial eco-toxicity potential |
Others
CMC | Carboxymethylcellulose |
DMC | Dimethyl carbonate |
DoD | Depth of discharge |
EC | Ethylene carbonate |
GHG | Greenhouse gas |
LCA | Life cycle assessment |
LCI | Life cycle inventory |
LIBs | Lithium-ion batteries |
NMP |
N-Methyl-2-pyrrolidone |
PVdF | Polyvinylidene fluoride |
SBR | Styrene-butadiene rubber |
SIBs | Sodium-ion batteries |
TOC | Toxic organic compounds |
Acknowledgements
All authors would like to acknowledge the basic funding of the Helmholtz Society.
References
- J. Tang, A. D. Dysart and V. G. Pol, Curr. Opin. Chem. Eng., 2015, 9, 34–41 CrossRef
.
- M. H. Han, E. Gonzalo, G. Singh and T. Rojo, Energy Environ. Sci., 2014, 8, 81–102 Search PubMed
.
- N. Yabuuchi, K. Kubota, M. Dahbi and S. Komaba, Chem. Rev., 2014, 114, 11636–11682 CrossRef CAS PubMed
.
-
L. Zyga, Physorg Sci. X Netw., 2015.
- V. Palomares, P. Serras, I. Villaluenga, K. B. Hueso, J. Carretero-González and T. Rojo, Energy Environ. Sci., 2012, 5, 5884–5901 CAS
.
- D. Larcher and J.-M. Tarascon, Nat. Chem., 2015, 7, 19–29 CrossRef CAS PubMed
.
- J.-M. Tarascon, Philos. Trans. R. Soc., A, 2010, 368, 3227–3241 CrossRef PubMed
.
- B. L. Ellis and L. F. Nazar, Curr. Opin. Solid State Mater. Sci., 2012, 16, 168–177 CrossRef CAS
.
- H. Pan, Y.-S. Hu and L. Chen, Energy Environ. Sci., 2013, 6, 2338–2360 CAS
.
-
C. Bauer, Paul Scherrer Inst. LEA Villigen Switz., 2010.
- D. A. Notter, M. Gauch, R. Widmer, P. Wäger, A. Stamp, R. Zah and H.-J. Althaus, Environ. Sci. Technol., 2010, 44, 6550–6556 CrossRef CAS PubMed
.
- M. Zackrisson, L. Avellán and J. Orlenius, J. Cleaner Prod., 2010, 18, 1519–1529 CrossRef CAS
.
- G. Majeau-Bettez, T. R. Hawkins and A. H. Strømman, Environ. Sci. Technol., 2011, 45, 4548–4554 CrossRef CAS PubMed
.
- L. Grande, E. Paillard, J. Hassoun, J.-B. Park, Y.-J. Lee, Y.-K. Sun, S. Passerini and B. Scrosati, Adv. Mater., 2015, 27, 784–800 CrossRef CAS PubMed
.
- P. G. Bruce, S. A. Freunberger, L. J. Hardwick and J.-M. Tarascon, Nat. Mater., 2012, 11, 19–29 CrossRef CAS PubMed
.
- U. Eberle, B. Müller and R. von Helmolt, Energy Environ. Sci., 2012, 5, 8780–8798 Search PubMed
.
- O. Z. Sharaf and M. F. Orhan, Renewable Sustainable Energy Rev., 2014, 32, 810–853 CrossRef CAS
.
- ISO, ISO 14040, Geneva, Switz., 2006.
- ISO, ISO 14044, Geneva, Switz., 2006.
- A. Nordelöf, M. Messagie, A.-M. Tillman, M. Ljunggren Söderman and J. Van Mierlo, Int. J. Life Cycle Assess., 2014, 19, 1866–1890 CrossRef
.
-
E. Moreno Ruiz, T. Lévová, G. Bourgault and G. Wernet, Ecoinvent Centre, Zürich, Switz., 2015 Search PubMed
.
-
H.-J. Althaus, G. Doka, T. Heck, S. Hellweg, R. Hischier, T. Nemecek, G. Rebitzer, M. Spielmann and G. Wernet, ecoinvent report No. 1, Dübendorf, Switz., 2007.
-
M. Goedkop, R. Heijungs, M. Huijbregts, A. De Schryver, J. Struijs and R. Van Zelm, ReCiPe 2008. First edition Report I, 2012.
-
S. Winter, Y. Emara, A. Ciroth, C. Su and M. Srocka, openLCA 1.4 - Comprehensive User Manual, GreenDelta GmbH, Berlin, Germany, 2015 Search PubMed.
- D. Kundu, E. Talaie, V. Duffort and L. F. Nazar, Angew. Chem., Int. Ed., 2015, 54, 3431–3448 CrossRef CAS PubMed
.
- K. Yanagida, A. Yanai, Y. Kida, T. Nohma and I. Yonezu, J. Electrochem. Soc., 2002, 149, A804–A807 CrossRef CAS
.
-
J. Barker and R. Heap, WO2014/009710 A1, 2014
.
- J.-H. Lee, S. Lee, U. Paik and Y.-M. Choi, J. Power Sources, 2005, 147, 249–255 CrossRef CAS
.
- S. F. Lux, F. Schappacher, A. Balducci, S. Passerini and M. Winter, J. Electrochem. Soc., 2010, 157, A320–A325 CrossRef CAS
.
-
J. Barker, R. Heap, N. Roche, C. Tan, R. Sayers and Y. Liu, Low Cost Na-ion Battery Technology, Faradion Ltd, San Francisco, US, 2014 Search PubMed
.
-
S. Ohmori and T. Yamamoto, US2012/0021273 A1, 2012
.
-
J. Barker and R. Heap, WO2014/009722 A1, 2014
.
- M. D. Slater, D. Kim, E. Lee and C. S. Johnson, Adv. Funct. Mater., 2013, 23, 947–958 CrossRef CAS
.
-
J.-M. Tarascon, C. Masquelier, L. Croguennec and S. Patoux, CNRS Press Releases, 2015.
-
J.B. Dunn, L.G. Gaines, M. Barnes, J. Sullivan and M. Wang, Report ANL/ESD/12-3, Argonne, US, 2012.
- A. W. Golubkov, D. Fuchs, J. Wagner, H. Wiltsche, C. Stangl, G. Fauler, G. Voitic, A. Thaler and V. Hacker, RSC Adv., 2014, 4, 3633–3642 RSC
.
- J. B. Dunn, L. Gaines, J. Sullivan and M. Q. Wang, Environ. Sci. Technol., 2012, 46, 12704–12710 CrossRef CAS PubMed
.
- B. M. Zimmermann, H. Dura and M. Weil, Metall. Res. Technol., 2014, 111, 169–178 CrossRef
.
-
P. Stenzel, M. Baumann, J. Fleer, B. Zimmermann and M. Weil, IEE Energycon, 2014, pp. 1334–1342 Search PubMed
.
- N. Sun, H. Liu and B. Xu, J. Mater. Chem. A, 2015, 3, 20560–20566 CAS
.
- L. Wu, D. Buchholz, C. Vaalma, G. A. Giffin and S. Passerini, ChemElectroChem, 2015, 292–298 Search PubMed
.
- L. A.-W. Ellingsen, G. Majeau-Bettez, B. Singh, A. K. Srivastava, L. O. Valøen and A. H. Strømman, J. Ind. Ecol., 2014, 18, 113–124 CrossRef CAS
.
Footnote |
† Electronic supplementary information (ESI) available: Detailed life cycle inventory data, input–output tables, information about the impact assessment methodology and the tabulated LCA result. See DOI: 10.1039/c6ee00640j |
|
This journal is © The Royal Society of Chemistry 2016 |