DOI:
10.1039/C5DT03928B
(Communication)
Dalton Trans., 2016,
45, 498-501
Low temperature isolation of a dinuclear silver complex of the cyclotetraphosphane [ClP(μ-PMes*)]2†
Received
7th October 2015
, Accepted 2nd December 2015
First published on 2nd December 2015
Abstract
The reaction of the cyclotetraphosphane [ClP(μ-PMes*)]2 (1, Mes* = 2,4,6-tri-tert-butylphenyl) with Ag[Al(ORF)4] (RF = CH(CF3)2) resulted in a labile, dinuclear silver complex of 1, which eliminates AgCl above −30 °C. Its properties were investigated by spectroscopic methods, single crystal X-ray diffraction and DFT calculations.
Introduction
Ring systems composed of Group 15 elements (pnictogens) are an intriguing aspect of main group chemistry.1 Especially, those comprising a binary N2E2 (E = P, As, Sb, Bi) scaffold represent versatile reagents in pnictogen chemistry.2 Metal complexes of such ring systems were investigated to quite some extent,3–5 including studies about their potential application in catalysis6 and even anti-tumour studies.7 Interestingly, despite the indisputable importance of phosphorus compounds as ligands in catalysis,8 the coordination chemistry of homologous P4 ring systems (cyclotetraphosphanes) has received much less attention. Examples include mainly P4R4 systems (A, Scheme 1; R = organic substituent) which coordinate to transition metal fragments such as metal carbonyls,9–13 metal halides14 or phosphane substituted metal complexes.15 The coordination chemistry of unsubstituted Pn scaffolds, on the other hand, is more versatile;16,17 in particular, there are numerous examples of coordination to P4, which can adopt either a tetrahedral (B1, B2),18–22 bicyclic (C1, C2)23–26 or rectangular/square planar structure (D1–D3)27–31 in the complex (Scheme 1). Likewise, the coordination chemistry of the cyclo-P5− moiety was well investigated; it found application in a variety of syntheses ranging from “simple” molecules such as Cp*FeP5 (Cp* = pentamethylcyclopentadiene)32,33 to chain-like poly-cations34,35 or spherical macromolecules.36–39
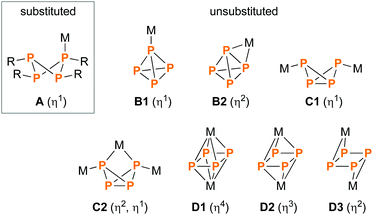 |
| Scheme 1 Common coordination patterns of various P4 scaffolds. | |
Results and discussion
Pursuing our interest in the chemistry of the recently reported cyclotetraphosphane [ClP(μ-PMes*)]2 (1),40 we explored its reactivity towards silver salts of the weakly coordinating anions [Al(ORF)4]− (RF = CH(CF3)2) and [B(C6F5)4]−.41,42 Taking advantage of the bulky, weakly coordinating anion and naked Ag+ ion – in terms of both stabilization and halide abstraction abilities – we hoped to find access to the dark red cyclotetraphosphenium ion [ClP(μ-PMes*)2P]+ (2+), which had previously been observed as an intermediate during the reaction of 1 and the Lewis acid GaCl3 (Scheme 2, top).43 However, when mixing Ag[Al(ORF)4] (4) and 1 in a 1
:
1 ratio in CH2Cl2 at low temperatures, no precipitation of AgCl was observed. Instead, crystallization at −80 °C afforded colourless crystals that were identified as CH2Cl2 solvate of a dimeric silver complex of 1 (5[Al(ORF)4]2, Scheme 2, bottom; yield of isolated substance: 28%).
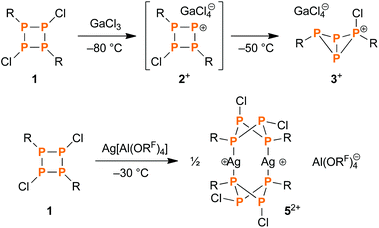 |
| Scheme 2 Top: the reaction of cyclophosphane 1 with GaCl3 led to the formation of the intermediate 2+. Bottom: the reaction of 1 and 4 at low temperatures yielded the dinuclear silver complex 52+ (R = Mes*). | |
Molecular structure
Most interestingly, both chlorine atoms remained at the P4 scaffold; nonetheless two rather long Ag⋯Cl contacts were observed, which are shorter than the sum of van der Waals radii (Ag1–Cl2A 3.496(1) Å, Ag1–Cl2A′ 3.641(2) Å; cf. ∑rvdW = 4.35 Å)44 as revealed by single crystal X-ray diffraction (Fig. 1). The P–Ag bond lengths (P1A–Ag1 2.394(2) Å, P3a–Ag1′ 2.391(2) Å) compare well to the sum of the covalent radii (2.39 Å),45 whereas the Ag–Ag distance (3.0511(7) Å) lies between the sum of the covalent (2.56 Å)45 and van der Waals radii (5.06 Å).44 The silver atoms are almost linearly coordinated (177.52(5)°) and lie in a perfect plane with the Mes* substituted P atoms. Strikingly, the configuration at P2 is inverted in comparison with the starting material, where both chlorine atoms are arranged in an equatorial position with respect to the P4 ring system. The dinuclear silver complex is nicely shielded by all four Mes* substituents: the Ag atoms and the equatorial Cl atoms (Cl2A, Cl2A′) are protected by the ortho-tBu groups, while the axial Cl atoms (Cl1A, Cl1A′) are sandwiched between the phenyl rings (Fig. S1, ESI†). A similar coordination pattern was previously observed in [(Cy4P4)2Sb2Cl2]2+ (Cy = cyclohexyl), where a planar Sb2Cl2 scaffold is coordinated by two Cy4P4 rings.46 To the best of our knowledge, it is the only other example of a dinuclear metal complex capped by two P4 ring systems.
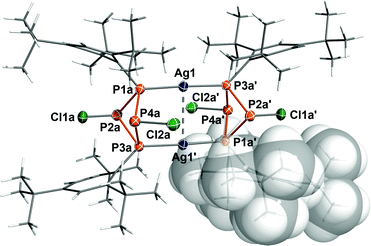 |
| Fig. 1 Molecular structure of 52+. Ellipsoids are set at 50% probability (173 K). The cation is situated on a crystallographic inversion centre and thus displays Ci symmetry. Selected bond lengths [Å] and angles [°]: P1a–Ag1 2.394(2), P1a–P2a 2.235(2), P1a–P4a 2.242(2), P2a–Cl1a 2.059(2), P2a–P3a 2.232(2), P3a–Ag1′ 2.391(2), P3a–P4a 2.252(2), P4a–Cl2a 2.069(2), Ag1–Ag1′ 3.0511(7), P3a′–Ag1–P1a 177.52(5), P1a–Ag1–Ag1′ 90.68(4), P3a′–Ag1–Ag1′ 87.24(4). | |
Spectroscopic characterization
The low temperature 31P NMR spectrum (−60 °C) of 5[Al(ORF)4]2 showed a complex AA′BB′MM′M′′M′′′XX′ spin system (Fig. 2). The A and B part were assigned to the inequivalent Cl substituted P atoms (axially substituted: 126.9 ppm, equatorially substituted: 122.1 ppm), the M part to the Mes* substituted P atoms (16.8 ppm) and the X part to the Ag nuclei. The 1J(31P–31P) coupling constants amount to −205 (1JAM) and −248 Hz (1JBM); the 1J(31P–107Ag) coupling is −468 Hz (1JMX), which compares to reported (unsigned) coupling constants of other two-coordinate complexes such as [(Ph3P)2Ag]+ (552 Hz) or [(p-Tol3P)2Ag]+ (496 Hz).47,48 Due to the complex multiplet structure and generally low solubility of 5[Al(ORF)4]2, the signal to noise ratio of the NMR spectrum was rather poor, which is why only the large 1J coupling constants could be determined unambiguously, while all smaller coupling constants have higher uncertainties. Nonetheless, the experimental data agree well with calculated NMR shifts and coupling constants (Table S3†).
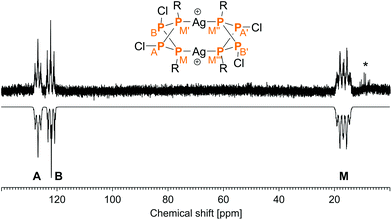 |
| Fig. 2 Experimental (up) and simulated (down) 31P NMR spectrum of 52+. Starred lines are due to slow elimination of AgCl even at low temperatures. | |
In the solid state Raman spectrum (at −60 °C), characteristic bands of the [P4Ag]2 fragment are observed at 464 cm−1 (P–P stretching within the P4 scaffold), 493 cm−1 (P–Cl stretching) and 514 cm−1 (P–C stretching). Further intense signals in the Raman spectrum can be attributed to the Mes* moieties (e.g. 563, 603 cm−1: tBu deformation; 739 cm−1: phenyl ring deformation; 1007, 1018 cm−1: Me deformation; 1130 cm−1: combination of ring and CH deformation; 1582 cm−1: ring stretching; 2900–3000 cm−1: CH stretching of Me groups) and the [Al(ORF)4]− anion (e.g. 563 cm−1: CF deformation; 746 cm−1: symmetrical AlO4 stretching; 818 cm−1: combination of deformation at O and CF3; 1364, 1386 cm−1: CH deformation; 2873 cm−1: CH stretching). The assignments of the signals were made on the basis of computed vibrational data.
Computational study
To further investigate the bonding situation in 52+, density functional theory (DFT) calculations were performed.† According to NBO analysis,49 there is no significant bonding interaction between the two Ag atoms, as already indicated by the nearly linear coordination of the Ag centres. Furthermore, both Wiberg bond index (0.31) and natural bond index (0.56) indicate a low covalent character of the Ag–P bonds; accordingly, the natural Lewis representation comprises two distinguished Ag+ cations and two neutral cyclophosphane moieties. Second order perturbation analysis reveals two stabilizing interactions per Ag+ cation between the empty s-orbital and the lone pairs (LPs) of the flanking P atoms (346.2 kJ mol−1 each), which is consistent with a classical dative bond from P to Ag (Fig. 3). The natural partial charge of each Ag centre is +0.59e, while each of the four coordinating P atoms bears a charge of +0.17e. Hence, the formal charge transfer amounts to −0.41e per Ag+ ion.
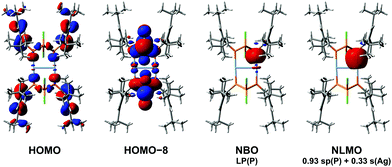 |
| Fig. 3 Left: molecular orbitals (MOs) of 52+. The HOMO shows contributions to the LPs at the coordinating P atoms, the HOMO−8 encompasses the LPs at Cl and the other P atoms as well as bonding within the P4 ring systems. Right: natural bond orbital (NBO) representation of a LP at phosphorus and the corresponding natural localized molecular orbital (NLMO), which consists mainly of a formal sp hybrid orbital at P (86%) and an s orbital at Ag (11%), thus illustrating the bonding between these two centres. | |
Intramolecular elimination of AgCl
When the reaction mixture of the cyclophosphane 1 and the silver salt 4 was allowed to warm to temperatures above −30 °C, precipitation of a white solid was observed, indicating elimination of AgCl. In situ31P NMR spectroscopy revealed that the intermediately formed silver complex 52+ decomposed above that temperature (Fig. S3†), yielding the bicyclic cation [Mes*P4(Cl)Mes*]+ (3+, Scheme 3), which had previously been obtained by reacting 1 with GaCl3 (Scheme 2, top).43 The same reaction outcome was observed when warming a solution of pure 5[Al(ORF)4]2. In this respect, 5[Al(ORF)4]2 can be viewed as an isolable intermediate, which demonstrates that the eventual AgCl elimination does not occur via direct attack of a silver ion, but rather via complexation and a subsequent intramolecular elimination reaction.
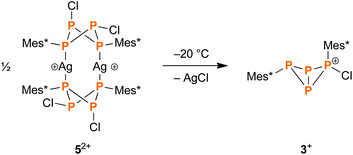 |
| Scheme 3 Above −30 °C, the silver complex 52+ eliminates AgCl, which leads to the formation of the bicyclic phosphino–phosphonium cation 3+. | |
Interestingly, when treating 1 with Ag[B(C6F5)4] (6) under the same reaction conditions, precipitation of AgCl was observed even at −80 °C, leading once more to the formation of 3+, as indicated by 31P NMR spectroscopy. However, an intermediate similar to the cyclotetraphosphenium cation 2+ could not be observed in any of these reactions.
Conclusions
In conclusion, we present a thermally labile dinuclear silver complex capped by two P4 ring systems, which eliminates AgCl at temperatures above −30 °C. Thus it can be considered an intermediate of the chloride abstraction from [ClP(μ-PMes*)]2, demonstrating that the reaction occurs via an intramolecular rather than an intermolecular process.
Acknowledgements
We would like to thank Dr Dirk Michalik for low temperature NMR measurements as well as the Fonds der Chemischen Industrie (scholarship for JB) and Deutsche Forschungsgemeinschaft (SCHU 1170/11-1) for funding.
Notes and references
- L. Stahl, Coord. Chem. Rev., 2000, 210, 203–250 CrossRef CAS.
- G. He, O. Shynkaruk, M. W. Lui and E. Rivard, Chem. Rev., 2014, 114, 7815–7880 CrossRef CAS PubMed.
- M. S. Balakrishna, V. S. Reddy, S. S. Krishnamurthy, J. F. Nixon and J. C. T. R. B. St. Laurent, Coord. Chem. Rev., 1994, 129, 1–90 CrossRef CAS.
- G. G. Briand, T. Chivers and M. Krahn, Coord. Chem. Rev., 2002, 233–234, 237–254 CrossRef CAS.
- M. S. Balakrishna, D. J. Eisler and T. Chivers, Chem. Soc. Rev., 2007, 36, 650–664 RSC.
- D. W. Stephan, Angew. Chem., Int. Ed., 2000, 39, 314–329 CrossRef.
- D. Suresh, M. S. Balakrishna, K. Rathinasamy, D. Panda and S. M. Mobin, Dalton Trans., 2008, 2812–2814 RSC.
-
Phosphorus(III) Ligands in Homogeneous Catalysis: Design and Synthesis, ed. P. C. J. Kamer and P. W. N. M. Van Leeuwen, John Wiley & Sons, 2012 Search PubMed.
- H. G. Ang, J. S. Shannon and B. O. West, Chem. Commun., 1965, 10–11 RSC.
- G. W. A. Fowles and D. K. Jenkins, Chem. Commun., 1965, 61–62 RSC.
- M. A. Bush, V. R. Cook and P. Woodward, Chem. Commun., 1967, 630–631 RSC.
- N. H. Tran Huy, Y. Inubushi, L. Ricard and F. Mathey, Organometallics, 1997, 16, 2506–2508 CrossRef.
- N. H. Tran Huy, Y. Lu and F. Mathey, Organometallics, 2011, 30, 1734–1737 CrossRef.
- C. S. Cundy, M. Green, F. G. A. Stone and A. Taunton-Rigby, J. Chem. Soc. A, 1968, 1776–1778 RSC.
- I. G. Phillips, R. G. Ball and R. G. Cavell, Inorg. Chem., 1992, 31, 1633–1641 CrossRef CAS.
- M. Scheer, G. Balázs and A. Seitz, Chem. Rev., 2010, 110, 4236–4256 CrossRef CAS PubMed.
- B. M. Cossairt, N. A. Piro and C. C. Cummins, Chem. Rev., 2010, 110, 4164–4177 CrossRef CAS PubMed.
- O. J. Scherer, Comments Inorg. Chem., 1987, 6, 1–22 CrossRef CAS.
- I. Krossing, J. Am. Chem. Soc., 2001, 123, 4603–4604 CrossRef CAS PubMed.
- V. Mirabello, M. Caporali, V. Gallo, L. Gonsalvi, D. Gudat, W. Frey, A. Ienco, M. Latronico, P. Mastrorilli and M. Peruzzini, Chem. – Eur. J., 2012, 18, 11238–11250 CrossRef CAS PubMed.
- S. Heinl, E. V. Peresypkina, A. Y. Timoshkin, P. Mastrorilli, V. Gallo and M. Scheer, Angew. Chem., Int. Ed., 2013, 52, 10887–10891 CrossRef CAS PubMed.
- F. Spitzer, M. Sierka, M. Latronico, P. Mastrorilli, A. V. Virovets and M. Scheer, Angew. Chem., Int. Ed., 2015, 54, 4392–4396 CrossRef CAS PubMed.
- S. Heinl and M. Scheer, Chem. Sci., 2014, 5, 3221–3225 RSC.
- C. Schwarzmaier, A. Y. Timoshkin, G. Balázs and M. Scheer, Angew. Chem., Int. Ed., 2014, 53, 9077–9081 CrossRef CAS PubMed.
- S. Pelties, D. Herrmann, B. de Bruin, F. Hartl and R. Wolf, Chem. Commun., 2014, 7014–7016 RSC.
- C. Schwarzmaier, S. Heinl, G. Balázs and M. Scheer, Angew. Chem., Int. Ed., 2015, 54, 13116–13121 CrossRef CAS PubMed.
- W. W. Seidel, O. T. Summerscales, B. O. Patrick and M. D. Fryzuk, Angew. Chem., Int. Ed., 2009, 48, 115–117 CrossRef CAS PubMed.
- A. S. P. Frey, F. G. N. Cloke, P. B. Hitchcock and J. C. Green, New J. Chem., 2011, 35, 2022 RSC.
- A. Velian and C. C. Cummins, Chem. Sci., 2012, 3, 1003 RSC.
- C. Camp, L. Maron, R. G. Bergman and J. Arnold, J. Am. Chem. Soc., 2014, 136, 17652–17661 CrossRef CAS PubMed.
- S. Yao, N. Lindenmaier, Y. Xiong, S. Inoue, T. Szilvási, M. Adelhardt, J. Sutter, K. Meyer and M. Driess, Angew. Chem., Int. Ed., 2015, 54, 1250–1254 CrossRef CAS PubMed.
- O. J. Scherer and T. Brück, Angew. Chem., Int. Ed. Engl., 1987, 26, 59–59 Search PubMed.
- M. Baudler, S. Akpapoglou, D. Ouzounis, F. Wasgestian, B. Meinigke, H. Budzikiewicz and H. Münster, Angew. Chem., Int. Ed. Engl., 1988, 27, 280–281 CrossRef.
- S. Welsch, L. J. Gregoriades, M. Sierka, M. Zabel, A. V. Virovets and M. Scheer, Angew. Chem., Int. Ed., 2007, 46, 9323–9326 CrossRef CAS PubMed.
- C. Heindl, S. Heinl, D. Lüdeker, G. Brunklaus, W. Kremer and M. Scheer, Inorg. Chim. Acta, 2014, 422, 218–223 CrossRef CAS.
- M. Scheer, J. Bai, B. P. Johnson, R. Merkle, A. V. Virovets and C. E. Anson, Eur. J. Inorg. Chem., 2005, 5, 4023–4026 CrossRef.
- M. Scheer, A. Schindler, R. Merkle, B. P. Johnson, M. Linseis, R. F. Winter, C. E. Anson and A. V. Virovets, J. Am. Chem. Soc., 2007, 129, 13386–13387 CrossRef CAS PubMed.
- C. Schwarzmaier, A. Schindler, C. Heindl, S. Scheuermayer, E. V. Peresypkina, A. V. Virovets, M. Neumeier, R. Gschwind and M. Scheer, Angew. Chem., Int. Ed., 2013, 52, 10896–10899 CrossRef CAS PubMed.
- F. Dielmann, C. Heindl, F. Hastreiter, E. V. Peresypkina, A. V. Virovets, R. M. Gschwind and M. Scheer, Angew. Chem., Int. Ed., 2014, 53, 13605–13608 CrossRef CAS PubMed.
- J. Bresien, C. Hering, A. Schulz and A. Villinger, Chem. – Eur. J., 2014, 20, 12607–12615 CrossRef CAS PubMed.
- I. Krossing and A. Reisinger, Coord. Chem. Rev., 2006, 250, 2721–2744 CrossRef CAS.
- I. Krossing and I. Raabe, Angew. Chem., Int. Ed., 2004, 43, 2066–2090 CrossRef CAS PubMed.
- J. Bresien, K. Faust, A. Schulz and A. Villinger, Angew. Chem., Int. Ed., 2015, 54, 6926–6930 CrossRef CAS PubMed.
- S. Alvarez, Dalton Trans., 2013, 42, 8617–8636 RSC.
- P. Pyykkö and M. Atsumi, Chem. – Eur. J., 2009, 15, 12770–12779 CrossRef PubMed.
- E. Conrad, N. Burford, U. Werner-Zwanziger, R. McDonald and M. J. Ferguson, Chem. Commun., 2010, 46, 2465–2467 RSC.
- R. E. Bachman and D. F. Andretta, Inorg. Chem., 1998, 37, 5657–5663 CrossRef CAS PubMed.
- E. L. Muetterties and C. W. Alegranti, J. Am. Chem. Soc., 1972, 94, 6386–6391 CrossRef CAS.
-
E. D. Glendening, J. K. Badenhoop, A. E. Reed, J. E. Carpenter, J. A. Bohmann, C. M. Morales, C. R. Landis and F. Weinhold, NBO 6.0, Theoretical Chemistry Institute, University of Wisconsin, Madison, 2013 Search PubMed.
Footnote |
† Electronic supplementary information (ESI) available: Experimental and computational details, crystallographic and spectroscopic data. CCDC 1417701. For ESI and crystallographic data in CIF or other electronic format see DOI: 10.1039/c5dt03928b |
|
This journal is © The Royal Society of Chemistry 2016 |
Click here to see how this site uses Cookies. View our privacy policy here.