STM investigation of structural isomers: alkyl chain position induced self-assembly at the liquid/solid interface†
Received
28th September 2015
, Accepted 16th November 2015
First published on 19th November 2015
Abstract
Investigating and regulating the self-assembly structure is of great importance in 2D crystal engineering and it is also gaining significant interest in surface studies. In this work, we systematically explored the variation of self-assembled patterns induced by the changeable side chain position. Moreover, molecules with different alkyl chain lengths (n = 15, 16) were also synthesized and probed for the purpose of understanding how an odd/even number of carbon atoms in the peripheral chains can affect the molecular adlayers. Structural isomers of bis-substituted anthraquinone derivatives 1,8-A-2OCn, 2,6-A-2OCn, 1,4-A-2OCn and 1,5-A-2OCn (n = 15, 16) were used and investigated by STM. 1,8-A-2OC16 and 1,8-A-2OC15 molecules adopted Z-like I and Linear I structures, respectively. 2,6-A-2OC16 and 2,6-A-2OC15 molecules were severally arranged in Linear II and Linear III configurations. 1,4-A-2OCn (n = 15, 16) molecules were staggered in a Z-like II fashion and 1,5-A-2OCn (n = 15, 16) molecules displayed a Linear IV nanostructure. Therefore, we arrive at a conclusion that self-assembly structures of anthraquinone isomers are chain-position-dependent, and designing isomeric compounds can be taken into consideration in regulating assembled structures. Besides, 2D nanopatterns of 1,8-A-2OCn and 2,6-A-2OCn can be regulated by the odd/even property of the side chains, but this is not the case for 1,4-A-2OCn and 1,5-A-2OCn, ascribed to the difference in driving forces for them. It is believed that the results are of significance to the alkyl chain position induced assembly configurations and surface research studies of structural isomers.
1. Introduction
Molecular self-assembly is a ubiquitous phenomenon in nature and has been recognized as a powerful means of fabricating functional nanomaterials.1 Thus, designing and creating two-dimensional (2D) architectures on solid surfaces based on molecular self-assembly are attracting great attention at present. A π-conjugated system can be achieved by employing well-designed molecules as building blocks2,3 and it has promoted advances in the field of organic materials, for example, molecular devices, molecular-scale electronics and liquid crystal materials.4,5 Therefore, understanding the arrangements of molecules on the substrate are fundamental for the development of molecular nanostructures and nanodevices.2,6–15 Scanning tunneling microscopy (STM) is a preferred technique for the investigation of self-assembled monolayers under ultrahigh vacuum conditions, and the liquid/solid or air/solid interface.16,17 STM offers submolecular visualization and also manipulation of the adsorbates. The successful formation and the subsequent visualization of the adlayer require a balance between various forces.18 In general, with the purpose of successfully controlling 2D molecular arrangements, the following noncovalent interactions and experimental parameters need to be considered. (i) Optimization of intermolecular interactions, for instance, hydrogen bonds,19–21 metal–ligand coordination,22–24 dipolar coupling,25–28 and van der Waals interactions.29 (ii) Molecule–substrate interactions. Molecules adsorb onto the surface typically via van der Waals interaction, which can be modulated by functional groups and changing the substrate.30 (iii) Experimental conditions. Temperature,31–34 concentration of the solution,20,35–38 and the time of scanning39 are well known to play an important role in 2D network formation.
Chemical structure, among other crucial factors which determine self-assembled nanopatterns, is of ever increasing importance in 2D crystal engineering. Structural isomers are common in organic chemistry. They are a series of molecules, which have the same molecular formulas, but different constitutional formulas. Disaffinity in the chemical structure can result in completely different chemical and physical properties, such as color, appearance, melting behavior, steric configuration, photoelectric performance, and so on. With the development in preparing supramolecular assemblies, designing and synthesizing structural isomers have attracted a lot of attention.40,41 A large number of papers have reported the effect of odd/even number of carbon atoms in substituent alkyl chains on molecular structures.42–46 This is a usual phenomenon in many systems, ascribed to the demand of minimizing steric repulsion between the methyl groups, and it is a suggestion that the formation of 2D nanostructures is dominated by the interactions between alkyl chains.44
Different self-assembly structures from isomers of tetrathienoanthracene derivatives have been reported by Fu et al.41 They showed that a slight geometric difference between the two isomers (position of the sulfur atoms) may lead to a dramatic change in 2D molecular networks. Yoshihiro and coworkers reported various 2D structures of bipyridine derivatives.40 Structural isomers of p-substituted (1C16p) and m-substituted (1C16m) bipyridine with hexadecyl adopted completely different assembly structures. Namely, 1C16p showed an almost linear form of zigzag-type alignment of the π-conjugated units, whereas, 1C16m adopted Z-shaped morphology via alkyl chain interdigitation. Anthraquinone derivatives have been explored in the field of liquid crystals because of their low cost, high temperature stability, and stability under ambient conditions.47 Matthew and his group reported the self-assembly properties of 1,5-di(octyloxy)-anthraquinone (15DA) on highly ordered pyrolytic graphite (HOPG).48 15DA formed well-ordered monolayers through octyl chain interdigitation and planar adsorption of the anthracene ring. Yanhu et al. demonstrated dipolar controlled self-assembly of anthracene derivatives.27 1,5-Bis-substituted-anthracene with an odd number of carbon atoms in the alkyl chain formed linear structures with the anthracene groups in adjacent rows pointing to the same direction, whereas, that with an even number of carbon atoms adopted a reverse direction of the anthracene moieties. As such, subtleties of the self-assembly can be explored systematically with an emphasis on the role of the molecular structure and the odd–even effect.
Herein, we explored different 2D nanopatterns which were obtained because of structural changes with STM at the liquid/solid interface. There are four kinds of anthraquinone isomers, which consist of an anthraquinone unit and two side chains in the 1,8-position, 2,6-position, 1,4-position and 1,5-position: 1,8-bis(n-alkoxy)-9,10-anthracenedione (1,8-A), 2,6-bis(n-alkoxy)-9,10-anthracenedione (2,6-A), 1,4-bis(n-alkoxy)-9,10-anthracenedione (1,4-A) and 1,5-bis(n-alkoxy)-9,10-anthracenedione (1,5-A), as shown in Fig. 1. For the purpose of exploring how an odd/even number of carbon atoms in the side chains can influence the self-assembled networks, we synthesized tetradecyl and pentadecyl substituted anthraquinone derivatives, respectively. The relative position of the substituted side-chains leads to a number of molecular configurations and entirely different physical and chemical properties. In this paper, we primarily probed the structural isomerism induced 2D self-assembled features and mechanism, and incidentally studied their differential scanning calorimetry (DSC) performance and ultraviolet (UV) absorption spectra.
 |
| Fig. 1 Chemical structures, molecular models, and the calculated HOMOs (alkyl chains are replaced by methyl substituents for ease of calculation) of 1,8-A, 2,6-A, 1,5-A, and 1,4-A derivatives. | |
2. Experimental section
Structural isomers of 1,8-A, 2,6-A, 1,4-A and 1,5-A were synthesized as described in the ESI† (Scheme S1), and then recrystallized repeatedly in order to ensure the purity. The solutions of the products dissolving in CH2Cl2 were prepared for observation of their colors (Fig. S1, ESI†). 1-Octanoic acid was purchased from Tokyo Chemical Industry and used without further purification. The molecules were dissolved in 1-octanoic acid. The solutions used in our STM work had 50% saturation and the values are given in the corresponding sections. At first, 100% saturated solutions were prepared, and then they were diluted to 50% saturation according to accurate proportions. The samples were prepared by depositing a droplet (about 1 μL) of solution on a freshly cleaved atomically flat surface of HOPG (quality ZYB, Bruker, USA). STM experiments were performed on a Nanoscope IIIa Multimode SPM (Bruker, USA) under ambient conditions (temperature: 15–20 °C, humidity: 50%). The tips were mechanically cut from Pt/In wires (80/20). All images were recorded with constant current mode and shown without further processing. Imaging conditions are given in the corresponding figure captions. Material studio 4.4 was used to build the molecular models of the assembled structures and calculate the HOMOs. The DSC experiments were conducted with a scan rate of 10 °C min−1 for heating and cooling traces (instrument information: NETZSCH DSC 200F3). The UV absorption spectra were tested by dissolving the compounds in CH2Cl2 (the concentrations are given in the corresponding sections) using an ultraviolet spectrometer (equipment: Agilent Cary 60).
3. Results
Self-assembly patterns were immediately observed by STM measurement after depositing a drop of solution onto the HOPG surface. 1-Octanoic acid was selected as the solvent, and no solvent coadsorption was observed. Therefore, we conclude that the structural isomers of anthraquinone derivatives adopted configurations which are as denser as possible, with the solvent exerting its role only as a dispersant.
3.1 Self-assembly of 1,8-A-2OCn (n = 15, 16)
Initially, we investigated the self-assembled structures that were obtained by dropping 1,8-A-2OC16 solution at the 1-octanoic acid/HOPG interface. A large-scale STM image of 1,8-A-2OC16 self-assembled adlayer is shown in Fig. 2a. It can be clearly seen that bright stripes and dark troughs form the ordered pattern without any defects. The bright stripes are ascribed to the π-conjugated units of 1,8-A-2OC16, while the dark troughs are the peripheral alkyl chains. Fig. 2b shows a high-resolution STM image, which reveals the arrangement details of this Z-like structure, named Z-like I. The black solid arrows depicted in the left bottom of Fig. 2b displays the main symmetry axes of the graphite under the monolayer. Every two 1,8-A-2OC16 molecules adopt an arrangement in a head-to-head fashion and then all of the acetabuliform dimers aggregate with each other, forming Z-like stripes. Alkyl chains in adjacent stripes are interdigitated and extend along the direction of the basic graphite axis. Therefore, van der Waals interactions of molecule–molecule and molecule–substrate are maximumly enlarged, resulting in a stable and closely-packed 2D self-assembled monolayer. The angle between the side chain and the macroaxis of the anthraquinone unit is measured to be θ = 113 ± 2° (as shown in Fig. 2b). A structural model is tentatively proposed in Fig. 2c, which is in accordance with the high-resolution STM image. According to the periodicity of the molecular self-organization, a unit cell is superimposed in Fig. 2b. The lattice constants are determined to be a = 1.2 ± 0. 3 nm, b = 2.3 ± 0.3 nm and α = 75 ± 1°. Every unit cell consists of two molecules and the calculated area density is 1.33 nm2 per molecule.
 |
| Fig. 2 (a) Large-scale and (b) high-resolution STM images of 1,8-A-2OC16 self-assembled monolayer on the HOPG surface. Concentration: 3.92 × 10−3 M. (c) Molecular model of the Z-like structure (Z-like I). (e) Large-scale STM image of 1,8-A-2OC15 self-assembled monolayer on the HOPG surface (Linear I). The pink and green arrows represent different alkyl chain orientations of the tail-to-tail stripes. Concentration: 4.84 × 10−3 M. (f) High-resolution STM image of 1,8-A-2OC15. (g) Molecular model of the lamellar structure. A set of black arrows in (b) and (f) show the basic symmetry axis of the graphite substrate. The angles between the alkyl chain and the macroaxis of the anthraquinone unit in (b) and (f) are marked with the values of θ, θ1, and θ2. Weak hydrogen bonds C O⋯H–C forming 2D architectures are illustrated in (d) and (h). The long alkyl chains are replaced by methyl groups. Imaging conditions: It = 550 pA, Vbias = 700 mV for (a) and (b), It = 580 pA, Vbias = 790 mV for (e) and (f). | |
With the decrease of the alkyl chain length by one carbon atom, different assembled structures were discerned. As shown in Fig. 2e, the large-scale STM image reveals that 1,8-A-2OC15 adopted a linear configuration, denoted Linear I. The emergence of bright stripes and dark troughs is ascribed to the high and low tunneling current of the π-conjugated anthraquinone units and alkyl chains. Fig. 2f shows the high-resolution STM image, showing the detailed packing pattern of this linear configuration. It is obvious that the alkyl chains are arranged in a tail-to-tail fashion, instead of interdigitated ones as in the case of 1,8-A-2OC16. We have experimentally proved that this tail-to-tail fashion is not concentration-dependent. The pink and green arrows in Fig. 2e represent different orientations of the side-chains. Nevertheless, the arrangements of the orientation along which the chains extend are irregular and random, which means, the tail-to-tail alkyl chains of 1,8-A-2OC15 molecules in adjacent rows are arranged in either a parallel or V-like fashion. The black solid arrows in Fig. 2f demonstrate the 3-fold symmetry of the HOPG substrate. An STM image consists of 1,8-A-2OC15 molecules and the HOPG surface can be observed by varying the tunneling bias (see ESI,† Fig. S2), and this image is powerful evidence that alkyl chains extend along the main orientation of the graphite lattice. The angles β1 and β2 in Fig. 2f are measured to be 120 ± 1°, which allow the skeletons of the alkyl chains to match with the graphite lattice. This is proved to be one of the driving forces for the formation of a stable self-assembled adlayer in a 2D engineering system.46 The angles between the orientation of the alkyl chains and the anthraquinone moieties are measured to be θ1 = 101 ± 1° and θ2 = 123 ± 1°. On the basis of molecular arrangement, a structural model for the ordered linear monolayer is proposed in Fig. 2g. A unit cell is outlined in Fig. 2f and the parameters are determined to be a = 0.8 ± 0.2 nm, b = 8.8 ± 0.2 nm and α = 89 ± 2°. Every unit cell consists of four molecules and the calculated area density is 1.78 nm2 per molecule. The 1,8-A-2OCn (n = 15, 16) adlayers are formed via van der Waals interactions between the π-conjugated backbones, as also between the adsorbates and the substrate. In addition, hydrogen bonds make a great contribution to stabilizing the Z-like and linear structures, shown as enlarged pictures in Fig. 2d and h. In this work, hydrogen bonds are formed with the carbonyl groups acting as an acceptor and hydrogen atoms in its neighboring anthraquinone as a donor.
3.2 Self-assembly of 2,6-A-2OCn (n = 15, 16)
Fig. 3a shows a typical large-scale STM image of the 2,6-A-2OC16 adlayer physisorbed at the liquid/solid interface of HOPG. The structural details are resolved by a high-resolution STM image shown in Fig. 3b. The angle between the alkyl chain and the macroaxis of the anthraquinone is measured to be θ = 124 ± 2°. It can be clearly seen that the basic unit of the monolayer is a number of trimers, which are indicated by pink rectangles. Interestingly, we found that no matter how the domains change, molecules tend to gather consistently, every three molecules assemble together except for some accidental circumstance, which means missing a single molecule or dislocation (indicated by blue arrows in Fig. 3b), and is rare to be observed. Molecules in a trimer gather together via van der Waals interactions and C
O⋯H–C hydrogen bonds (Fig. 3d) between the π-conjugated anthraquinone units, and then all of the alkyl chains in adjacent rows interdigitate with each other, forming this stable 2D arrangement (named Linear II). However, molecules within a molecular row do not form perfectly straight lines. Periodic molecular dislocation along the direction of the alkyl chains can be explained on the basis of strong molecule–substrate interactions and the registry mechanism of the side chains with the underlying graphite.1 A careful observation suggests that the liquid/solid surface is covered by lots of small domains, instead of the highly ordered monolayer, and as a consequence, structural defects are a common phenomenon, as denoted by red circles in Fig. 3a. This may be proof that trimers in this monolayer show mobility along the direction of the main graphite axis. A unit cell is outlined in Fig. 3b and the parameters are determined to be a = 2.3 ± 0.5 nm, b = 2.6 ± 0.5 nm and α = 77 ± 2°. Every unit cell consists of three molecules and the calculated area density is 1.94 nm2 per molecule. Fig. 3c shows the temporary molecular model and it is in good agreement with the STM results.
 |
| Fig. 3 (a) Large-scale STM image of 2,6-A-2OC16 self-assembled monolayer on the HOPG surface. Structural defects are denoted by red circles. Concentration: 2.79 × 10−4 M. (b) High-resolution STM images of 2,6-A-2OC16 molecules. Six pink rectangles represent the trimers which make up the basic unit of the adlayer. Structural defects (single molecule dislocation or missing) are indicated by blue arrows. (c) Molecular model of the trimer linear structure (Linear II). (e) Large-scale and (f) high-resolution STM images of 2,6-A-2OC15 self-assembled monolayer on the HOPG surface. Concentration: 1.98 × 10−4 M. (g) Molecular model of the lamellar structure (Linear III). A set of black arrows in (b) and (f) show the basic symmetry axis of the graphite substrate. The angles between the alkyl chain and the macroaxis of the anthraquinone unit in (b) and (f) are marked by the value of θ. Weak hydrogen bonds C O⋯H–C forming 2D architectures are illustrated in (d) and (h). The long alkyl chains are replaced by methyl groups. Imaging conditions: It = 570 pA, Vbias = 820 mV for (a) and (b), It = 610 pA, Vbias = 870 mV for (e) and (f). | |
To gain further insight into exploring how the odd/even number of carbon atoms in the side chains can affect the self-assembly structures, another compound, 2,6-A-2OC15, was also synthesized and investigated by STM measurement. 2,6-A-2OC15 molecules adopt a linear structure (named Linear III), as shown in the large-scale STM image of Fig. 3e. The monolayer which covers the HOPG surface with orderly domains is constituted of stripes. The high-resolution STM image in Fig. 3f manifests more structural details. Anthraquinone units in adjacent rows are not parallel, but rather form a V-like fashion. This rotation of a whole row is needed for the matching of neighboring carbon atoms in adjacent stripes, then alkyl chains are fully interdigitated, and thus minimize the steric repulsion.43,45 This V-like fashion is an indication that one of the driving forces for this kind of assembled configuration is the interaction between the alkyl chains. The angle between the side chain and the macroaxis of the anthraquinone is measured to be θ = 143 ± 1°. A unit cell is superimposed in Fig. 3f. The unit cell parameters are determined to be a = 0.9 ± 0.3 nm, b = 4.7 ± 0.3 nm and α = 85 ± 1°. Every unit cell consists of two molecules and the calculated area density is 2.11 nm2 per molecule. On the basis of a large amount of high-resolution STM images, a structural model for this molecular structure is proposed in Fig. 3g (the rotated rows are depicted with blue carbon atom skeletons), which is in good agreement with the experimental results. Hydrogen bonding between the adjacent anthraquinone units, as shown in Fig. 3h, is another kind of main interaction to force molecules to arrange in this linear fashion.
Moreover, chirality is a common phenomenon which is observed during our STM performance. 2D chirality of molecular self-assembly on a solid surface has attracted lots of attention.49 In this study, chirality was observed as shown in Fig. 4. Several domains with the anthraquinone moieties orienting in different directions can be clearly recognized, as marked by blue (left-handed) and pink (right-handed) arrows. A small area is enlarged in Fig. 4b. The two enantiomeric domains are separated by red dotted lines, and their anthraquinone units form an angle of 72° (36° + 36°). The chiral domains have mirror symmetry and cannot be superimposed by the rotation of any angle. Interestingly, alkyl chains on two sides of the red dotted line are parallel to each other, assuming that they are extending in a comfort way, without inter-collisions and unstable domain boundaries. A proposed molecular model for this chiral structure is depicted in Fig. 4c. An achiral molecule of 2,6-A-2OC16 self-assembled into chiral structures on an achiral surface of HOPG. A similar chiral phenomenon has been explored and explained by the reduced freedom and constraint of the substrate lattice.50–53 Statistical analysis of more than ten images (scanning area: 100 × 100 nm2) collected at different locations from different samples reveals that the emergence probability of left-handed and right-handed structures is almost 1
:
1. The calculated average coverage ratios are 47.2% for the left-handed network and 52.8% for the right-handed pattern. We guess that the two enantiomorphous configurations, regardless of priorities and their coverage ratios, would further approach 50% if the collected large-scale images are as many as possible.
 |
| Fig. 4 (a) STM images of a chiral monolayer of 2,6-A-2OC16, with a small area enlarged in (b). Concentration: 2.79 × 10−4 M. (c) A chiral structure model based on the molecular arrangement. Imaging conditions: It = 570 pA, Vbias = 820 mV. | |
3.3 Self-assembly of 1,4-A-2OCn (n = 15, 16)
The alkyl chain position provides an excellent approach for controlling 2D self-assembly ordering. 1,4-A-2OCn (n = 15, 16) were used here, as other compounds of anthraquinone isomers. Fig. 5a shows the large-scale STM image of 1,4-A-2OC16 physisorbed on the HOPG surface. A zigzag structure defined as Z-like II can be discerned. The high-resolution image, as shown in Fig. 5b, displays the arrangement details. Every two molecules gather together in a head-to-head fashion, forming an acetabuliform dimer. This is very familiar with the assembly patterns of 1,8-A-2OC16 (Fig. 2b). A careful observation suggests that alkyl chains in the 1-position and the 4-position spin to the same side, parallel to the macroaxis of the anthraquinone unit, as indicated in Fig. 6a. However, side chains of 1,8-A-2OC16 are on the same side of the π-conjugated anthraquinone moieties, and they can form a closely packed arrangement without the spin of the peripheral alkyl groups, as shown in Fig. 6b. This is powerful evidence that ether groups show great flexibility during the self-assembly process and as a consequence, alkyl chains adopt appropriate rotations in consideration of the closest packing and minimizing the adsorption energy. A unit cell consisting of two molecules is overlaid with the measured parameters of a = 1.4 ± 0.4 nm, b = 3.5 ± 0.4 nm and α = 86 ± 2°. The calculated area density is 2.44 nm2 per molecule. Fig. 5d shows the proposed structural model for the Z-like II arrangement and it is in good agreement with the STM results. C
O⋯H–C hydrogen bonds between the π-conjugated anthraquinone units are the main forces in the acetabuliform dimers, as shown in Fig. 5e. In addition, 1,4-A-2OC15 molecules were investigated and we found that there are no differences in structural patterns between 1,4-A-2OC16 and 1,4-A-2OC15 molecules, whether in large-scale STM images (Fig. S3a, ESI†) or high-resolution ones (Fig. 5c and Fig. S3b, ESI†). The structural model, unit cell parameters and hydrogen bonds for 1,4-A-2OC15 are shown in Fig. S3 (ESI†). Therefore, we conclude that the odd/even property of alkyl chains shows no impact on the structural diversity of 1,4-A-OCn (n = 15, 16) and the driving forces for the self-assembly process are molecule–molecule, molecule–substrate and hydrogen bonding interactions.
 |
| Fig. 5 (a) Large-scale and (b) high-resolution STM images of 1,4-A-2OC16 self-assembled monolayer on the HOPG surface. Concentration: 4.98 × 10−3 M. A set of black arrows in (b) show the basic symmetry axis of the graphite substrate. (c) Self-assembly pattern of 1,4-A-2OC15. Concentration: 5.34 × 10−3 M. (d) Molecular model of the zigzag structure (Z-like II) based on the STM image of 1,4-A-2OC16. (e) Illustration of C O⋯H–C hydrogen bonding interactions within the dimer aggregates. The long alkyl chains are replaced by methyl groups. Imaging conditions: It = 590 pA, Vbias = 860 mV for (a) and (b), It = 640 pA, Vbias = 830 mV for (c). | |
 |
| Fig. 6 Molecular models of 1,4-A-2OC16 (a) and 1,8-A-2OC16 (b). (a) Alkyl chains in the 1-position and the 4-position spinning to the same side, parallel to the macroaxis of the anthraquinone unit. (b) Side chains in the 1-position and the 8-position are on the same side of the π-conjugated anthraquinone moiety. | |
3.4 Self-assembly of 1,5-A-2OCn (n = 15, 16)
1,5-A-2OCn (n = 15, 16) molecules showed great central symmetry and they self-assembled on the graphite surface into identical structures no matter if the number of carbon atoms in the peripheral alkyl chain is odd or even. Fig. 7a shows the large-scale STM image of 1,5-A-2OC16. The HOPG substrate is covered by orderly arranged molecules with their side chains fully extended and interdigitated. This lamella structure is named Linear IV. Details of the assembled pattern are demonstrated in Fig. 7b. A set of black arrows are imposed in the left bottom, as an indication of the symmetry axis of the graphite lattice. The HOPG surface has a pronounced influence on the configuration of molecules, as the alkyl chains are running parallel to one of the main substrate axes. The angle between the alkyl chain and the macroaxis of the anthraquinone group is measured to be θ = 148 ± 2°. A unit cell is outlined in Fig. 7b and the parameters are determined to be a = 1.0 ± 0.1 nm, b = 3.1 ± 0.1 nm and α = 83 ± 1°. Every unit cell consists of one molecule and the calculated area density is 3.08 nm2 per molecule. Fig. 7d shows the temporary molecular model for 1,5-A-2OC16 and it is in good agreement with the STM results. This model can also be used to illustrate the molecular structure of 1,5-A-2OC15, which has been shown in the large-scale STM image of Fig. 7c. Further investigation provided detailed information on this linear structure which is shown in Fig. S4 (ESI†). Alkyl chains of 1,5-A-2OC15 form an angle of θ = 117 ± 2° with the macroaxis of the anthraquinones (Fig. S4b, ESI†). The area density is calculated to be 2.97 nm2 per molecule. In addition, a careful observation suggests that molecules in the monolayer are closely packed, without the π-conjugated anthraquinone spinning to form a V-like structure, as in the case of 2,6-A-2OC15. This can also be regarded as an indication that the driving forces for this lamella structure of 1,5-A-2OCn (n = 15, 16) are van der Waals interactions between molecule–molecule, molecule–substrate, and hydrogen bonds among anthraquinone moieties (Fig. 7e and Fig. S4d, ESI†).
 |
| Fig. 7 (a) Large-scale and (b) high-resolution STM images of 1,5-A-2OC16 self-assembled monolayer on the HOPG surface. Concentration: 2.87 × 10−3 M. A set of black arrows in (b) show the basic symmetry axis of the graphite substrate. (c) Self-assembly pattern of 1,5-A-2OC15. Concentration: 1.96 × 10−3 M. (d) Molecular model of the linear structure (Linear IV) based on the STM image of 1,5-A-2OC16. (e) Illustration of C O⋯H–C hydrogen bonding interactions within the linear structure. The long alkyl chains are replaced by methyl groups. Imaging conditions: It = 530 pA, Vbias = 750 mV for (a) and (b), It = 600 pA, Vbias = 820 mV for (c). | |
4. Discussion
To further compare the structural difference in anthraquinone isomers, we systematically summarized the geometric characteristics, unit cell parameters and packing densities of self-assembled patterns of 1,8-A, 2,6-A, 1,4-A and 1,5-A (n = 15, 16) molecules physisorbed at the 1-octanoic acid/HOPG interface, as shown in Table 1.
Table 1 Comparison of 1,8-A, 2,6-A, 1,4-A and 1,5-A (n = 15, 16) polymorphs by molecular modelling
Molecule |
Structural model |
Phase |
Basic unit |
a (nm) |
b (nm) |
α (°) |
θ (°) |
N
|
S
(nm²) |
N = number of molecules per unit cell.
S represents the area density.
|
1,8-A |
C16 |
|
Z-like I |
Dimer |
1.2 ± 0. 3 |
2.3 ± 0. 3 |
75 ± 1 |
113 ± 2 |
2 |
1.33 |
C15 |
|
Linear I |
Dimer |
0.8 ± 0.2 |
8.8 ± 0.2 |
89 ± 2 |
123 ± 1 |
4 |
1.78 |
|
|
|
101 ± 1 |
|
|
2,6-A |
C16 |
|
Linear II |
Trimer |
2.3 ± 0.5 |
2.6 ± 0.5 |
77 ± 2 |
124 ± 2 |
3 |
1.94 |
C15 |
|
Linear III |
Single molecule |
0.9 ± 0.3 |
4.7 ± 0.3 |
85 ± 1 |
143 ± 1 |
2 |
2.11 |
1,4-A |
C16 |
|
Z-like II |
Dimer |
1.4 ± 0.4 |
3.5 ± 0.4 |
86 ± 2 |
0 |
2 |
2.44 |
C15 |
1.5 ± 0.1 |
3.5 ± 0.1 |
74 ± 1 |
0 |
2 |
2.52 |
1,5-A |
C16 |
|
Linear IV |
Single molecule |
1.0 ± 0.1 |
3.1 ± 0.1 |
83 ± 1 |
148 ± 2 |
1 |
3.08 |
C15 |
1.1 ± 0.2 |
2.7 ± 0.2 |
88 ± 2 |
117 ± 1 |
1 |
2.97 |
4.1 Role of π-conjugated anthraquinone moieties and hydrogen bonds
π-Conjugated anthraquinone moieties play an important role in the process of forming orderly 2D self-assembled patterns because of their high electronic density. Besides, the orientation and the dipole direction of two carbonyl groups in the 9,10-position of the anthraquinone skeleton are reverse and thus lead to great structural symmetry. This symmetrical arrangement extinguishes the polarity of molecules, and as a result, anthraquinone derivatives show weak polarity. Therefore, the π-conjugated anthraquinone cores exert their role through the van der Waals interaction between the anthraquinone core and the substrate, π–π stacking and hydrogen bonding interactions. Moreover, due to their relatively strong, selective and directional nature, hydrogen bonds showed tremendous regulation in 2D crystal engineering. Tamaki et al. have reported the weak C
O⋯H–C hydrogen bonding formed between anthraquinone units, which was the dominant force in stabilizing the 2D self-assembled monolayers.54 In structural isomers of 1,8-A, 2,6-A, 1,4-A and 1,5-A (n = 15, 16), weak hydrogen bonds exist between anthraquinone moieties. This sort of hydrogen bond plays an important role in the process of molecular recognition and then can give rise to well-organized structures on surfaces.19 In our study, hydrogen bonding interactions are powerful forces to make single molecules in a row to be orderly arranged, as shown in Fig. 2d, h, 3d, h, 5e, and 7e, Fig. S3d and S4d (ESI†), illustrating the detailed information on C
O⋯H–C hydrogen bonds within different monolayers.
4.2 Alkyl chain position induced self-assembly diversity and property difference
Chemical structure is one of the dominant factors which exert a direct influence on self-assembled monolayers on the surface. Anthraquinone isomers are the target products obtained by changing the alkyl chain position, with the purpose of achieving diverse 2D nanostructures. In the case of cetyl substituted structural isomers, 1,8-A-2OC16, 2,6-A-2OC16, 1,4-A-2OC16 and 1,5-A-2OC16 adopted Z-like I (basic unit: dimer), Linear II (basic unit: trimer), Z-like II (basic unit: dimer) and Linear IV (basic unit: single molecule) structures, respectively. Their calculated area densities are 1.33, 1.94, 2.44 and 3.08 nm2 per molecule. It is obvious that the density order is 1,8-A-2OC16 > 2,6-A-2OC16 > 1,4-A-2OC16 > 1,5-A-2OC16. However, in the case of pentadecyl substituted anthraquinone isomers, 1,8-A-2OC15, 2,6-A-2OC15, 1,4-A-2OC15 and 1,5-A-2OC15 adopted Linear I (basic unit: dimer), Linear III (basic unit: single molecule), Z-like II (basic unit: dimer) and Linear IV (basic unit: single molecule) structures, respectively. Their calculated area densities are 1.78, 2.11, 2.52 and 2.97 nm2 per molecule and the density order is 1,8-A-2OC15 > 2,6-A-2OC15 > 1,4-A-2OC15 > 1,5-A-2OC15.
Anthraquinone isomers have the same molecular formulas but adopt completely different assembly structures, have the same single-molecule area but show hugely different packing densities which change in a large range. Therefore, we draw a conclusion that the alkyl chain position can strongly regulate the 2D self-assembled nanostructures.
As a step further, we conducted some performance testing. Different alkyl chain positions can lead to entirely different chemical and physical properties. The most obvious indication of distinction between the anthraquinone isomers is color discrepancy. The four kinds of compounds were dissolved in CH2Cl2, and the solution color was compared at the concentration of 10−2 M. As shown in Fig. S1 (ESI†), the immediate appearance of dark and light yellow color is characteristic of these structural isomers.
Fig. 8 shows the ultraviolet (UV) absorption spectra of 1,8-A, 2,6-A, 1,4-A and 1,5-A (n = 15, 16) compounds in CH2Cl2 solution. They peak at 385, 350, 380 and 420 nm, respectively, as shown in Table S1 (ESI†), which gives a summary of the maximum absorbance peaks of anthraquinone isomers. The concentration of these four kinds of isomers was 10−5 M (n = 16) and 10−6 M (n = 15). The characteristic peaks observed in Fig. 8 are assigned to π–π* electron transition,55 and they are not related to the length of alkyl chains. Besides, the height of the absorbance peak is influenced by the concentration of the solution. Then we adopted different concentrations in order to avoid spectral line overlaps of compounds with the same substituent position but different chain lengths. We inferred that the observed spectral shift is correlated with the alkyl chain position, that is to say, structural isomers show different UV absorption properties.56,57 For anthraquinone isomers, the absorption peaks display a gradual red-shift as the alkyl chain changes from the 2,6-position to the 1,4-position, 1,8-position, and then to the 1,5-position.
 |
| Fig. 8 UV absorption spectra of anthraquinone isomers in CH2Cl2 solution at room temperature. Concentration: 10−5 M (n = 16) and 10−6 M (n = 15). | |
In addition, we investigated the phase transition behavior of these anthraquinone isomers. Differential scanning calorimetry (DSC) traces of 1,8-A, 2,6-A, 1,4-A and 1,5-A (n = 15, 16) on heating are shown in Fig. 9. The property difference induced by the alkyl chain position is remarkable, as indicated by the temperature in the horizontal ordinate, which corresponded to the heat flow peak in the vertical coordinate. Clearing temperature (melting point) variation tendency measured by the DSC experiments is 2,6-A-2OC16 (117 °C) > 1,8-A-2OC16 (112 °C) > 1,5-A-2OC16 (102 °C) > 1,4-A-2OC16 (91 °C) and 2,6-A-2OC15 (111 °C) > 1,8-A-2OC15 (109 °C) > 1,5-A-2OC15 (104 °C) > 1,4-A-2OC15 (97 °C), as also summarized in Table S2 (ESI†). This discrepancy in the melting point is ascribed to different energies required to form a uniform phase from the solid state.58 Therefore, we conclude that these four kinds of anthraquinone isomers need quite different energies for self-assembling into ordered 2D formation, thus different kinds of structures are formed, which are stable both thermodynamically and kinetically.
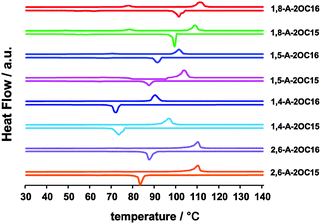 |
| Fig. 9 DSC thermograms of compounds 1,8-A, 2,6-A, 1,4-A and 1,5-A (n = 15, 16) for the trace of heating (the above line) and cooling (the below line). | |
4.3 Influence of odd/even number of carbon atoms in the alkyl chains on self-assembled nanostructures
The odd/even structural change in the alkyl chain has been identified as the possible origin of difference in the melting point and dynamics of monolayers,59 thus it is a common phenomenon that an odd/even number of carbon atoms in the peripheral alkyl chains can greatly influence self-assembly structures. A large amount of papers have reported the dependence of molecular configurations on the odd–even effect.42–46,60 In this present work, the monolayers of 1,8-A and 2,6-A compounds are intimately related to the odd/even property of the side chains.
1,8-A-2OC16 molecules gather together in a head-to-head fashion and then all of the acetabuliform dimers aggregate with each other, forming Z-like I stripes. Alkyl chains in adjacent stripes are interdigitated for close packing. But for 1,8-A-2OC15, the molecules self-assembled into Linear I structure without Z-like stripes. Anthraquinone moieties are staggered in a head-to-head fashion, but alkyl chains in adjacent stripes are arranged in a tail-to-tail mode. It is well known that the alkyl chains show great commensurability with the graphite surface and tend to extend along the direction of the main graphite axis so as to maximize the adsorbate–substrate interactions.42,61 We observed that alkyl chains of 1,8-A-2OC15 in adjacent rows oriented at 120° angles or parallel to each other, as illustrated in Fig. 2f and Fig. S22, (ESI†), indicating the effect of the substrate on the assembling process. Detailed information on these two structures is shown in Table 1. The non-interdigitated pentadecyl chains resulted in decreased area density for 1,8-A-2OC15, as the S value of 1.78 nm2 denoted, by comparing with 1.33 nm2 for 1,8-A-2OC16. A question arises why 1,8-A-2OC16 self-assembled into closed structures with the alkyl chains interdigitated with each other, but the side chains of 1,8-A-2OC15 were arranged in a tail-to-tail fashion. The same STM results obtained from experiments with solution concentration varying from 5% saturation (4.84 × 10−4 M) to 100% saturation (9.68 × 10−3 M) indicate that the phenomenon of non-interdigitated alkyl chains was caused by the odd number of carbon atoms in the side chains instead of the low concentration. Fig. 10 shows the stick model of 1,8-A-2OC16 and 1,8-A-2OC15. The long chains are tiled on the substrate, and carbon atoms are connected in an up-down-up-down (zigzag) fashion. The angle between the alkyl chain and the macroaxis of the anthraquinone corresponded to the STM results shown in Fig. 2b and f. For molecules of 1,8-A-2OC16, carbon atoms in neighboring chains are oriented in the same direction, as shown in Fig. 10a by pink arrows, and the steric repulsion is reduced. Alkyl chains of 1,8-A-2OC15 molecules are assumed to be staggered in an interdigitated fashion, as shown in Fig. 10b. However, in this hypothetical case, no matter how molecules moved along the direction of the chains, part of the carbon atoms in neighboring chains are oriented in a head-to-head fashion, as the head-to-head arrows indicated. As a result, steric repulsion is too strong to be able to stabilize the adsorbates and consequently, interdigitated alkyl chains of 1,8-A-2OC15 are nonexistent.
 |
| Fig. 10 Stick model of 1,8-A-2OC16 and 1,8-A-2OC15. (a) The actual model based on STM results. (b) The assumed model in which alkyl chains are interdigitated. The direction of carbon atoms is denoted by pink arrows. The repulsive and non-repulsive regions are marked by blue rectangles for ease of comparison. | |
2,6-A-2OC16 molecules adopted a Linear II structure. It can be clearly seen that the basic unit of the monolayer is a number of trimers, which are indicated by pink rectangles in Fig. 3b and c. Trimers in a row are discontinuous, showing orderly dislocation along the direction of the alkyl chains, and molecules in neighboring rows are parallel to each other. Nevertheless, anthraquinone units of 2,6-A-2OC15 molecules in neighboring rows are not parallel, forming a V-like fashion, named Linear III structures. Both of these two structures are closely packed, without any uncovered HOPG surface, though the calculated area density for 2,6-A-2OC16 (S = 1.94 nm2 per molecule) is slightly higher than that for 2,6-A-2OC15 (S = 2.11 nm2 per molecule). Fig. 11a and d show the space-filling sphere figures which display the matched end methyls with methylene groups within the interdigitated side chains. The adjacent alkyl chains tend to be arranged with their end methyls orienting in the direction of the methylenes which are connected with the ether groups. This is necessary for avoiding or decreasing steric repulsion. 2,6-A-2OC16 molecules are staggered with the anthraquinones parallel to each other, but 2,6-A-2OC15 molecules adopted a V-like fashion. A careful analysis indicates that the nonparallel rows of 2,6-A-2OC15 are needed for changing the direction of the end methyl and then obtain well-matched alkyl chains. For the purpose of exploring why anthraquinones in these two molecules are arranged in different ways, we put forward two hypothetical cases, as shown in Fig. 11b and c. The carbon atom skeletons of the rotated molecules are denoted in blue for the ease of distinguishing. The yellow arrows indicate the direction of the end methyls and methylenes which are connected with the ether groups. Arrows in the blue ovals (Fig. 11a and d) are oriented in the same direction, leading to matched alkyl chains. But in the pink ovals (Fig. 11b and c), neighboring arrows are in a head-to-head or tail-to-tail way.
 |
| Fig. 11 Space-filling spheres figure representation of the matched end methyls with methylene groups within the interdigitated side chains. The orange arrows indicate the direction of the end carbon atoms. Blue and pink ovals represent the matched and repulsive carbon atoms, respectively. (a and c) Models for 2,6-A-2OC16 and 2,6-A-2OC15, with the anthraquinones in neighboring rows arranged parallel to each other. (b and d) Models for 2,6-A-2OC16 and 2,6-A-2OC15, with the anthraquinones in neighboring rows arranged in a V-like fashion, which are the result of rotation from (a) and (c). | |
It is easy to judge that steric repulsion of Fig. 11a is smaller than that of Fig. 11b, and steric repulsion of Fig. 11d is smaller than that of Fig. 11c. Therefore, the assumed cases shown in Fig. 11b and c are unreasonable, and the molecular rotation for 2,6-A-2OC15 is needed.
However, 1,4-A and 1,5-A showed no structural difference when the alkyl chain length (n = 15, 16) is taken into consideration. Both 1,4-A-2OC16 and 1,4-A-2OC15 adopted a Z-like II structure. The detailed differences between them are unit cell parameters, as summarized in Table 1. The area density for the former is 2.44 nm2 per molecule and 2.52 nm2 per molecule for the latter. The 1,4-A-2OC16 molecule is larger by one methylene in the side chain than 1,4-A-2OC15, while the area for a single molecule for 1,4-A-2OC16 is smaller than that for 1,4-A-2OC15. Thus, we confirm that the Z-like II structure for 1,4-A-2OC16 is denser than that for 1,4-A-2OC15. 1,5-A-2OC16 and 1,5-A-2OC15 self-assembled into the same linear IV structure. The 1,5-A-2OC16 molecule is larger by one methylene in the side chain than 1,4-A-2OC15, and the area density of a single molecule for the former (S = 3.08 nm2 per molecule) is slightly larger than that for the latter (S = 2.97 nm2 per molecule). Then we infer that the degree of close packing for 1,5-A-2OC16 and 1,5-A-2OC15 is nearly the same. The non-emergence of the odd/even effect for 1,4-A and 1,5-A can be regarded as an indication that the driving force for them to self-assemble into ordered monolayers is the van der Waals interactions between molecule–molecule, molecule–substrate, and hydrogen bonds among anthraquinone moieties, and they are strong enough to stabilize the molecules, without taking well-matched alkyl chains into consideration.
5. Conclusion
Structural isomers of anthraquinone derivatives 1,8-A, 2,6-A, 1,4-A and 1,5-A (n = 15, 16) were synthesized and tested via STM for the sake of exploring how the alkyl chain position and the odd/even property can influence the self-assembled patterns on the liquid/solid surface of HOPG. Weak hydrogen bonds, alkyl chain interdigitation, π–π stacking and adsorbate–substrate interactions are the main forces which dominated the anthraquinone derivatives to stagger into ordered and close packing. 1,8-A, 2,6-A, 1,4-A and 1,5-A with side chains of n = 16 adopted Z-like I, Linear II, Z-like II and Linear IV phases, respectively. Structural differences were induced by the change in the substituent position. Compared with 1,8-A-2OC16 and 2,6-A-2OC16, 1,8-A-2OC15 and 2,6-A-2OC15 self-assembled into Linear I and Linear III arrangements, indicating that the odd/even number of carbon atoms in the alkyl chain can influence the process of self-assembly. However, 1,4-A and 1,5-A showed that the odd–even effect did not induce the structural difference, and then we arrive at a conclusion that the driving forces for 1,4-A and 1,5-A molecules to be orderly arranged on the HOPG surface were hydrogen bonding and molecule–substrate interactions. Moreover, chirality was observed for achiral molecules of 2,6-A-2OC16, and the left-handed and right-handed networks are regardless of priorities. Much is to be learned and explored on regulating the process of self-assembly for anthraquinone isomers.
Acknowledgements
Financial support from the National Program on Key Basic Research Project (2012CB932900), the National Natural Science Foundation of China (21573077, 51373055, and 21403072), the China Postdoctoral Science Foundation (2014M552189), and the Fundamental Research Funds for the Central Universities (SCUT) is gratefully acknowledged.
References
- K. S. Mali, K. Lava, K. Binnemans and S. De Feyter, Chem. – Eur. J., 2010, 16, 14447–14458 CrossRef CAS PubMed.
- J. M. Lehn, Proc. Natl. Acad. Sci. U. S. A., 2002, 99, 4763–4768 CrossRef CAS PubMed.
- G. M. Whitesides and M. Boncheva, Proc. Natl. Acad. Sci. U. S. A., 2002, 99, 4769–4774 CrossRef CAS PubMed.
- C. Joachim, J. K. Gimzewski and A. Aviram, Nature, 2000, 408, 541–548 CrossRef CAS PubMed.
- A. Nitzan and M. A. Ratner, Science, 2003, 300, 1384–1389 CrossRef CAS PubMed.
- J. V. Barth, J. Weckesser, C. Cai, P. Günter, L. Bürgi, O. Jeandupeux and K. Kern, Angew. Chem., 2000, 112, 1285–1288 CrossRef.
- J. V. Barth, J. Weckesser, C. Z. Cai, P. Gunter, L. Burgi, O. Jeandupeux and K. Kern, Angew. Chem., Int. Ed., 2000, 39, 1230–1234 CrossRef CAS.
- K. W. Hipps, L. Scudiero, D. E. Barlow and M. P. Cooke, J. Am. Chem. Soc., 2002, 124, 2126–2127 CrossRef CAS PubMed.
- S. De Feyter, M. M. S. A. Mottaleb, N. Schuurmans, B. J. V. Verkuijl, J. H. V. Esch, B. L. Feringa and F. C. De Schryver, Chem. – Eur. J., 2004, 10, 1124–1132 CrossRef CAS PubMed.
- D. G. Choi, S. Kim, E. Lee and S. M. Yang, J. Am. Chem. Soc., 2005, 127, 1636–1637 CrossRef CAS PubMed.
- M. Lackinger, S. Griessl, W. M. Heckl, M. Hietschold and G. W. Flynn, Langmuir, 2005, 21, 4984–4988 CrossRef CAS PubMed.
- L. Xu, X. R. Miao, L. H. Cui, P. Liu, K. Miao, X. F. Chen and W. L. Deng, J. Phys. Chem. C, 2015, 119, 17920–17929 CAS.
- M. Ruben, Angew. Chem., 2005, 117, 1620–1623 CrossRef.
- J. A. A. W. Elemans and S. De Feyter, Soft Matter, 2009, 5, 721–735 RSC.
- A. G. Phillips, L. M. A. Perdigao, P. H. Beton and N. R. Champness, Chem. Commun., 2010, 46, 2775–2777 RSC.
- S. Chiang, Chem. Rev., 1997, 97, 1083–1096 CrossRef CAS PubMed.
- S. De Feyter and F. C. De Schryver, J. Phys. Chem. B, 2005, 109, 4290–4302 CrossRef CAS PubMed.
- K. Tahara, K. Inukai, N. Hara, C. A. Johnson II, M. M. Haley and Y. Tobe, Chem. – Eur. J., 2010, 16, 8319–8328 CrossRef CAS PubMed.
- L. Kampschulte, M. Lackinger, A. K. Maier, R. S. K. Kishore, S. Griessl, M. Schmittel and W. M. Heckl, J. Phys. Chem. B, 2006, 110, 10829–10836 CrossRef CAS PubMed.
- L. Kampschulte, T. L. Werblowsky, R. S. K. Kishore, M. Schmittel, W. M. Heckl and M. Lackinger, J. Am. Chem. Soc., 2008, 130, 8502–8507 CrossRef CAS PubMed.
- Z. Mu, L. Shu, H. Fuchs, M. Mayor and L. Chi, J. Am. Chem. Soc., 2008, 130, 10840–10841 CrossRef CAS PubMed.
- M. A. Lingenfelder, H. Spillmann, A. Dmitriev, S. Stepanow, N. Lin, J. V. Barth and K. Kern, Chem. – Eur. J., 2004, 10, 1913–1919 CrossRef CAS PubMed.
- S. Stepanow, N. Lin, D. Payer, U. Schlickum, F. Klappenberger, G. Zoppellaro, M. Ruben, H. Brune, J. V. Barth and K. Kern, Angew. Chem., 2007, 119, 724–727 CrossRef.
- M. Surin, P. Samorì, A. Jouaiti, N. Kyritsakas and M. W. Hosseini, Angew. Chem., 2007, 119, 249–253 CrossRef.
- T. Yokoyama, S. Yokoyama, T. Kamikado, Y. Okuno and S. Mashiko, Nature, 2001, 413, 619–621 CrossRef CAS PubMed.
- M. de Wild, S. Berner, H. Suzuki, H. Yanagi, D. Schlettwein, S. Ivan, A. Baratoff, H. J. Guentherodt and T. A. Jung, ChemPhysChem, 2002, 3, 881–885 CrossRef CAS PubMed.
- Y. Wei, W. Tong, C. Wise, X. Wei, K. Armbrust and M. Zimmt, J. Am. Chem. Soc., 2006, 128, 13362–13363 CrossRef CAS PubMed.
- Y. Wei, W. Tong and M. B. Zimmt, J. Am. Chem. Soc., 2008, 130, 3399–3405 CrossRef CAS PubMed.
- X. Qiu, C. Wang, Q. Zeng, B. Xu, S. Yin, H. Wang, S. Xu and C. Bai, J. Am. Chem. Soc., 2000, 122, 5550–5556 CrossRef CAS.
- Z. Y. Yang, H. M. Zhang, C. J. Yan, S. S. Li, H. J. Yan, W. G. Song and L. J. Wan, Proc. Natl. Acad. Sci. U. S. A., 2007, 104, 3707–3712 CrossRef CAS PubMed.
- R. Gutzler, T. Sirtl, J. F. Dienstmaier, K. Mahata, W. M. Heckl, M. Schmittel and M. Lackinger, J. Am. Chem. Soc., 2010, 132, 5084–5090 CrossRef CAS PubMed.
- C. Marie, F. Silly, L. Tortech, K. Müllen and D. Fichou, ACS Nano, 2010, 4, 1288–1292 CrossRef CAS PubMed.
- D. Rohde, C. J. Yan, H. J. Yan and L. J. Wan, Angew. Chem., Int. Ed., 2006, 45, 3996–4000 CrossRef CAS PubMed.
- C. J. Li, Q. D. Zeng, Y. H. Liu, L. J. Wan, C. Wang, C. R. Wang and C. L. Bai, ChemPhysChem, 2003, 4, 857–859 CrossRef CAS PubMed.
- S. Lei, K. Tahara, F. C. De Schryver, M. Van der Auweraer, Y. Tobe and S. De Feyter, Angew. Chem., 2008, 120, 3006–3010 CrossRef.
- C. A. Palma, M. Bonini, A. Llanes-Pallas, T. Breiner, M. Prato, D. Bonifazi and P. Samori, Chem. Commun., 2008, 5289–5291 RSC.
- C. A. Palma, J. Bjork, M. Bonini, M. S. Dyer, A. L. Pallas, D. Bonifazi, M. Persson and P. Samorì, J. Am. Chem. Soc., 2009, 131, 13062–13071 CrossRef CAS PubMed.
- K. Tahara, S. Okuhata, J. Adisoejoso, S. Lei, T. Fujita, S. Der Feyter and Y. Tobe, J. Am. Chem. Soc., 2009, 131, 17583–17590 CrossRef CAS PubMed.
- L. Piot, A. Marchenko, J. Wu, K. Müllen and D. Fichou, J. Am. Chem. Soc., 2005, 127, 16245–16250 CrossRef CAS PubMed.
- Y. Kikkawa, E. Koyama, S. Tsuzuki, K. Fujiwara and M. Kanesato, Langmuir, 2010, 26, 3376–3381 CrossRef CAS PubMed.
- C. Fu, F. Rosei and D. F. Perepichka, ACS Nano, 2012, 6, 7973–7980 CrossRef CAS PubMed.
- I. De Cat, C. Gobbo, B. Van Averbeke, R. Lazzaroni, S. De Feyter and J. V. Esch, J. Am. Chem. Soc., 2011, 133, 20942–20950 CrossRef CAS PubMed.
- H. Fang, L. C. Giancarlo and G. W. Flynn, J. Phys. Chem. B, 1998, 102, 7421–7424 CrossRef CAS.
- Y. Kikkawa, E. Koyama, S. Tsuzuki, K. Fujiwara, K. Miyake, H. Tokuhisa and M. Kanesato, Chem. Commun., 2007, 1343–1345 RSC.
- F. Tao and S. L. Bernasek, Chem. Rev., 2007, 107, 1408–1453 CrossRef CAS PubMed.
- F. Tao, J. Goswami and S. L. Bernasek, J. Phys. Chem. B, 2006, 110, 4199–4206 CrossRef CAS PubMed.
- A. E. Murschell, W. H. Kan, V. Thangadurai and T. C. Sutherland, Phys. Chem. Chem. Phys., 2012, 14, 4626–4634 RSC.
- M. Pokrifchak, T. Turner, I. Pilgrim, M. R. Johnston and K. W. Hipps, J. Phys. Chem. C, 2007, 111, 7735–7740 CAS.
- L. Xu, X. R. Miao, L. H. Cui, P. Liu, X. F. Chen and W. L. Deng, Nanoscale, 2015, 7, 11734–11745 RSC.
- T. Chen, W. H. Yang, D. Wang and L. J. Wan, Nat. Commun., 2013, 4, 1389–1397 CrossRef PubMed.
- F. Masini, N. Kalashnyk, M. M. Knudsen, J. R. Cramer, E. Lægsgaard, F. Besenbacher, K. V. Gothelf and T. R. Linderoth, J. Am. Chem. Soc., 2011, 133, 13910–13913 CrossRef CAS PubMed.
- K. Tahara, H. Yamaga, E. Ghijsens, K. Inukai, J. Adisoejoso, M. O. Blunt, S. De Feyter and Y. Tobe, Nat. Chem., 2011, 3, 714–719 CrossRef CAS PubMed.
- M. Parschau, S. Romer and K. H. Ernst, J. Am. Chem. Soc., 2004, 126, 15398–15399 CrossRef CAS PubMed.
- T. Yoshinori, M. Kosuke and M. Kazuo, Bull. Chem. Soc. Jpn., 2013, 86, 354–362 CrossRef.
- L. H. Cui, X. R. Miao, L. Xu, Y. Hu and W. L. Deng, Phys. Chem. Chem. Phys., 2015, 17, 3627–3636 RSC.
- H. Fan, J. Smuts, L. Bai, P. Walsh, D. W. Armstrong and K. A. Schug, Food Chem., 2016, 194, 265–271 CrossRef CAS PubMed.
- L. Jin and P. Zhang, Chem. Eng. J., 2015, 280, 241–247 CrossRef CAS.
- J. Y. Wang, J. Yan, L. Ding, Y. Ma and J. Pei, Adv. Funct. Mater., 2009, 19, 1746–1752 CrossRef CAS.
- G. M. Florio, T. L. Werblowsky, B. Ilan, T. Müller, B. J. Berne and G. W. Flynn, J. Phys. Chem. C, 2008, 112, 18067–18075 CAS.
- L. Xu, X. R. Miao, B. Zha, K. Miao and W. L. Deng, J. Phys. Chem. C, 2013, 117, 12707–12714 CAS.
- J. P. Rabe and S. Buchholz, Science, 1991, 253, 424–427 CAS.
Footnote |
† Electronic supplementary information (ESI) available: Detailed description of the Experimental section and additional STM images. See DOI: 10.1039/c5cp05795g |
|
This journal is © the Owner Societies 2016 |