Fe3O4 and Au nanoparticles dispersed on the graphene support as a highly active catalyst toward the reduction of 4-nitrophenol†
Received
7th September 2015
, Accepted 13th November 2015
First published on 16th November 2015
Abstract
We report a highly efficient and reusable bifunctional nanostructured composite catalyst synthesized by a scalable facile hydrothermal method which enables the precise control of size and morphology of nanoparticles, wherein Au nanoparticles (NPs) and Fe3O4 particles exhibit excellent dispersing ability on the rGO (reduced graphene oxide) sheet surface (designated as rGO/Fe3O4/Au) to avoid adverse agglomeration between the nano particles and overlapping of the rGO sheets concurrently. The resultant bifunctional composite shows a high performance in the catalytic reduction of 4-nitrophenol (4-NP) with a conversion of 97% in 5 min and presents good reusability through highly efficient recovery by using external magnetic fields. In particular, there was no significant loss in catalytic activity of the reused catalysts even after being recycled for 8 cycles, displaying attractive potential in industrial applications where separation and recycling are imperative. The rational design provides an approach to synthesize a graphene-based composite with an interesting structure and multi-functional properties as well.
1. Introduction
In the past few decades, significant progress has been made with regard to the design, synthesis and utilization of nanostructured materials for efficient energy utilization and environmental protection.1 Among various systems, noble-metal nanostructures have received great interest due to their unique optical, catalytic and electrochemical properties which led to a number of fascinating potential applications in electronic, chemical, biological, hydrogen storage and catalytic fields.2 Au nanoparticles (NPs) have been proven to be highly efficient catalysts in several catalytic processes including low-temperature CO oxidation, the reductive catalysis of nitrogen oxides, acetylene hydrochlorination, the reduction of nitrophenol and organic synthesis. The catalytic performance of Au NPs is directly related to their size, shape and the dispersion.3 Unfortunately, unprotected Au NPs are active and susceptible to irreversible aggregation in solution during preparation and the catalytic process due to van der Waals forces and high surface energy. Certainly, the irreversible aggregation of Au NPs results in the substantial reduction of catalytic activity.
In order to overcome this drawback and improve the stability of Au NPs, an effective strategy to avoid the aggregation is introducing solid supports (such as silica, polymers, metal oxides, carbon materials, etc.) and then immobilizing the Au NPs on the supports to form composite catalysts.4 Carbon materials, for example carbon nanotubes (CNTs) and graphene, have been considered as impactful support materials owing to their high surface area, excellent loading capacity, appropriate pore sizes and abundant accommodation for NPs.5 Among them, support materials based on two dimensional (2D) reduced graphene oxide (rGO) have especially fascinated the scientific community due to their high electrical conductivity, large specific surface area, flexible structures and mechanical robustness.6 RGO presents outstanding capability to accommodate volume changes during the reaction and displays advantage in loading of the metal or metal oxides for potential applications in various fields.7 Moreover, this metal/metal oxide loading process can not only prevent graphene sheets from restacking but also improve the catalytic performance beneficial from the strong synergistic interaction between the two components.8 Furthermore, the strong covalent interfacing of the metal/metal oxides with graphene could also significantly reduce catalyst poisoning. Consequently, composite catalysts based on graphene loaded with metal/metal oxides exhibit high catalytic properties as well as good mechanical stability. There has been much effort made toward synthesizing highly dispersed Au NPs and assembling them on graphene to form different nanostructures. For instance, Li et al. synthesized Au/graphene composites under hydrothermal conditions through the self-assembly process, exhibiting an excellent catalytic performance towards the reduction of 4-NP to 4-Aminophenol (4-AP), which arises from the synergistic effect of graphene with Au NPs.9 Yao and co-workers recently developed a method to achieve the self-assembly of Au NPs on graphene sheets through chemical functionalization. These graphene/Au composites display good stability against agglomeration and show excellent catalytic activity in the reduction of 4-NP into 4-AP.10 Moreover, graphene-based composite catalysts such as graphene/Co3O4, graphene/MnCo2O4 and graphene/MoS2 have been studied as composite catalysts for environmental purification.11 In these cases, graphene serves as a support for the metal or metal oxide NPs. The graphene/NP composites can not only alleviate the aggregation problem of the NPs and the restacking of graphene, but also facilitate the electron transfer between the NPs and graphene during the catalytic process and improve the chemical stability as well. Although progress has been made, significant challenges remain in how graphene-based composite catalysts can be separated from the reaction solution and recycled further. Solving this problem is thus critical for their application in environmentally friendly process. Therefore, it is significant to develop well-defined nanostructure catalyst materials with a high surface area and unique separable features to enable convenient recycling. In this regard, integrating magnetic particles into the graphene-based catalyst composite as a magnetically recoverable catalyst support could be a promising solution.
In this work, we report a multifunctional catalyst composite with both magnetic and catalytic activities, which is synthesized by a hydrothermal method for the first time. GO sheets were modified by silica and they acted as the support for nanoparticles. The silica-modified GO sheets promoted the dispersing of Fe3O4 particles on graphene sheets during the simple in situ growth hydrothermal reaction using ferric acetylacetonate (Fe(acac)3) as a precursor. And then the Au NPs were evenly assembled to the as-prepared rGO/Fe3O4 by a hydrothermal method. The resulting product is designated as rGO/Fe3O4/Au. The synthesis offers good controllability of the size and dispersion of Fe3O4 and Au NPs with high-yield. The designed composite catalyst shows excellent dual functions: one is high catalytic efficiency towards the reduction of 4-NP (with a conversion of 97% in 5 min) in the presence of NaBH4; another is easy recyclability by using an external magnetic field without a significant decrease in the catalytic performance after 8 reuses.
2. Experimental section
All materials including natural graphite, cetyltrimethyl ammonium bromide (CTAB), ethanol, sodium hydroxide (NaOH), tetraethoxysilane (TEOS), concentrated ammonia solution (25 wt%), HAuCl4, sodium citrate, tannic acid, NaBH4 and 4-NP are of analytical grade and were purchased from Sinopharm Chemical Reagent Co., Ltd (China) and used as received without further purification.
2.1. Synthesis of silica modified GO
Graphite oxide (GO) was prepared from natural graphite by a modification of the Hummers method.12 The GO solution was prepared by dispersing GO in deionized water under sonication.
In a typical procedure, the silica modified graphite oxide sheets were prepared by hydrolysis of TEOS in an alcohol medium in the presence of water and ammonia by a modification of the Stöber method. 20 mg NaOH and 0.5 g CTAB were dispersed in 20 mL GO solution (1 mg mL−1) by ultra-sonication for 2 h at room temperature. The mixed solution was maintained at 40 °C in a water bath for 2 h and 1.2 mL TEOS was added drop-wise into the above solution with vigorous stirring. After another 12 h stirring, the precipitates (silica modified GO) were filtered and washed several times with deionized water and dried at 80 °C under vacuum for 6 h.
2.2. Synthesis of an rGO/Fe3O4 composite
20 mL deionized water and 0.08 g Fe(acac)3 were added into the above collected silica modified GO in turn and the mixed solution was stirred for 30 min, the pH value of the mixed solution was adjusted to 11 by ammonia solution (25%). After additional agitation for 5 min, the resultant solution was transferred into a 50 mL autoclave, sealed and heated at 220 °C for 12 h, followed by washing with deionized water and drying at 60 °C in air overnight. Finally the rGO/Fe3O4 composite was prepared.
2.3. Synthesis of an rGO/Fe3O4/Au composite
The Au NPs on the GO surface with a diameter of about 10 nm were prepared by the citrate reduction method in the presence of tannic acid. 0.4 mL of 1 g L−1 HAuCl4 was mixed with 30 mL deionized water containing 0.04 g sodium citrate and 0.02 g tannic acid and kept in a water bath at 60 °C for 4 h. The as-prepared rGO/Fe3O4 composite was dispersed in the above solution and stirred for 30 min. Finally, the resulting rGO/Fe3O4/Au composite was separated by centrifugation, rinsed with ethanol and dried at room temperature.
The rGO/Fe3O4/Au composite using GO only to anchor Fe3O4 and Au was prepared by a similar process without the modification of silica on the GO surface.
2.4. Catalytic reaction
The reduction of 4-NP compound by the rGO/Fe3O4/Au composite in the presence of NaBH4 was carried out to examine the catalytic activity and recyclability of the rGO/Fe3O4/Au composite catalyst. For comparison, the yellow aqueous 4-NP solution (0.08 M) was prepared and measured prior to monitoring the changes of absorption. A total of 50 μL 4-NP solution (5 mM) was mixed with 1.0 mL of fresh NaBH4 aqueous solution (0.2 M). Subsequently, 2.0 mL ultrapure water was swiftly added to the solution and it turned from light yellow to bright yellow immediately. Then a 4 mg rGO/Fe3O4/Au composite catalyst was added to the solution. The above solution was quickly subjected to UV-vis measurements, therefore, the obtained data can be designated as the value for reaction time t = 0. Changes in the concentration of 4-NP were monitored by UV-vis spectroscopy at room temperature. In fact, as the reaction proceeds, the color of the mixture gradually vanished, indicating the reduction of the 4-NP. To study the reusability of the rGO/Fe3O4/Au composite catalyst, the used samples were separated from the solution with a magnet after the monitoring of the whole reduction process was completed. The recycled magnetic microspheres were washed with ethanol 6 times and with deionized water 3 times for the next cycle. Similar to the above reduction process, the obtained rGO/Fe3O4/Au composite was redispersed with 2.0 mL of deionized water and mixed with 0.05 mL of aqueous 4-NP solution (0.005 M) and 1.0 mL of fresh NaBH4 (0.2 M) solution. With a stiff limit of 5 min kept for completion of the reaction, the solution was measured using UV-vis spectroscopy. The procedure was repeated 8 times and no significant decrease in the 4-NP conversion was detected.
2.5. Characterization
X-ray diffraction (XRD) patterns were collected on a Rigaku D/max-TTR-III diffractometer using Cu Kα radiation (λ = 0.15405 nm). Raman spectra were measured on a Renishaw RM2000 Raman spectrometer with 457.9 nm wavelength incident laser light. The measurements of X-ray photoelectron spectra (XPS) were performed by using a VG ESCALAB MK II electron energy spectrometer using Mg Kα (1253.6 eV) as the X-ray excitation source. The morphologies of the as-prepared nanocomposites were inspected on a scanning electron microscope (SEM, JSM-6480A, Japan Electronics), an energy dispersive X-ray (EDX) and a transmission electron microscope (TEM). The high-resolution transmission electron microscopy (HRTEM) micrographs were acquired on a FEI Tecnai G2 S-Twin transmission electron microscope with a field emission gun operating at 200 kV. N2 adsorption/desorption isotherms were measured at a liquid nitrogen temperature (−196 °C) using a Micromeritics ASAP 2010 instrument. The specific surface area was calculated by the Brunauer–Emmett–Teller (BET) method. FT-IR spectra were measured on a Perkin-Elmer 580BIR spectrophotometer using the KBr technique. The absorbance of 4-NP was obtained on a TU-1901 UV/vis spectrophotometer. The samples were placed in a 1 cm × 1 cm × 3 cm quartz cuvette and the spectra were recorded at room temperature. Magnetization measurements were performed on a MPM5-XL-5 superconducting quantum interference device (SQUID) magnetometer at 300 K.
3. Results and discussion
3.1. Morphology, structure, phase and textural properties
The crystal structures of the pure GO, rGO/Fe3O4 and rGO/Fe3O4/Au composites were characterized by XRD and shown in Fig. 1. The XRD pattern of the GO consists of one major peak at around 10°, which is in good agreement with the reported results and confirms that GO was successfully synthesized from graphite by the modified Hummers method.13 Four resolved peaks at 2θ = 19.09°, 31.25°, 36.8° and 59.3° in the XRD pattern of the rGO/Fe3O4/Au composite can be indexed into the fcc Fe3O4 structure. While the peaks at 2θ = 38.4°, 44.6°, 65° and 77.3° are commonly observed in the ace-centered cubic structured Au NP XRD patterns. The sharp and strong peaks confirm that the Fe3O4 and Au are highly crystallized. The XRD pattern of the rGO/Fe3O4/Au composite matches well with the corresponding JCPDS standards of Fe3O4 and Au (JCPDS 26-1136; JCPDS 04-0784), indicating that both the Au NPs and Fe3O4 were successfully located onto GO sheets. The diffraction peaks for Au are similar to Fe3O4. More evidence to prove the successful loading of Au NPs was shown in the discussion in Fig. 3. In the XRD pattern of the rGO/Fe3O4 composite, a broad XRD peak at around 25° which is indicated by the red circle could be ascribed to the (002) plane of the reduced graphene. Moreover, the disappearance of the GO peak at 2θ = 9.9° confirms the reduction of GO to rGO in the composite which indicates that GO was reduced to rGO by thermal reduction during the preparation of a rGO/Fe3O4 composite. The typical features of graphene are also observed in the TEM images described below.
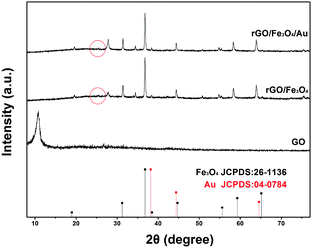 |
| Fig. 1 XRD patterns of the pristine GO, rGO/Fe3O4 and the rGO/Fe3O4/Au composite. | |
Raman spectroscopy was used to gain information on the structure of the graphene sheets in the rGO/Fe3O4/Au composite and GO. In Fig. 2, two intense peaks are clearly displayed at 1350 cm−1 (D band) and 1590 cm−1 (G band) in the Raman spectrum of the rGO/Fe3O4/Au composite and GO.14 It is well known that the peak intensity ratio of the D and G band (ID/IG) could be used to estimate the disorder degree and the average size of the sp2 domains of the graphite structure.15 In our experiment, ID/IG of the rGO/Fe3O4/Au composite increases compared with that of GO. The increased ID/IG ratio (1.11 for rGO/Fe3O4/Au and 1.04 for GO, respectively) is likely due to the decrease in the size of the sp2 graphitic domains during the preparation process and the occurrence of more defects in the graphite structure by the reduction process.
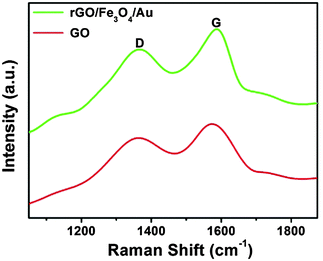 |
| Fig. 2 Raman spectra of the pristine GO sheets and the rGO/Fe3O4/Au composite. | |
X-ray photoelectron spectroscopy (XPS) was used to study the surface chemistry of the prepared reduced graphene oxide based composite. Fig. 3A shows XPS spectra of the rGO/Fe3O4/Au composite and GO. Compared with the pristine graphene sheets, the survey spectrum of rGO/Fe3O4/Au prominently shows carbon, oxygen, Au and iron species. In the survey region (0–1200 eV), the peak located at 285 eV is ascribed to the characteristic peak of C 1s, which can be divided into several peaks further. As seen from Fig. 3B, the deconvolution of the C 1s spectrum of rGO/Fe3O4/Au indicates the presence of nonoxygenated carbon at 284.8 eV, carbon in C–O (hydroxyl and epoxy groups) at 285.6 eV, C
O (carbonyl groups) at 287.1 eV and O–C
O (carboxyl groups) at 288.5 eV. XPS C 1s data for reduced graphite oxide show considerable restoration of the sp2 hybridized carbon atoms (C–C) after reduction by citrate, demonstrating that GO has been well deoxygenated to form graphene (Fig. S1, ESI†). In addition, the presence of the ferriferrous oxidation state is confirmed from the multiplet splitting of the Fe 2p peak, as shown in Fig. 3C. Two predominant band peaks at 711.2 eV and 723.8 eV can be assigned to Fe (2p3/2) and Fe (2p1/2), respectively, confirming the oxidation state of Fe3O4 in the composite. The Au 4f peaks at the binding energies of 84.1 and 87.8 eV, as shown in Fig. 3D, are attributed to Au 4f7/2 and Au 4f5/2, respectively. The two strong Au characteristic peaks suggest clearly the formation of the Au NPs in rGO/Fe3O4 with good crystallization. These results are also consistent with the XRD and Raman analysis as described above.
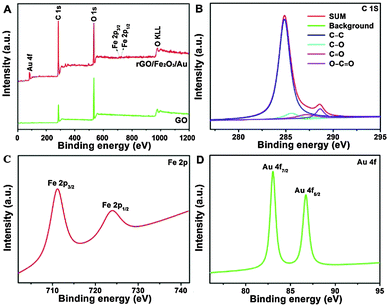 |
| Fig. 3 XPS spectra of the pure GO sheets and the as-prepared rGO/Fe3O4/Au composite. XPS survey spectra (A), the C 1s spectrum of rGO/Fe3O4/Au (B), the Fe 2p spectrum of the rGO/Fe3O4/Au composite (C) and the Au 4f spectrum of the rGO/Fe3O4/Au composite (D). | |
Fig. 4 shows the TEM images and morphology sketch of the GO modification process which plays an important role in the growth of Fe3O4 and the deposition of Au NPs. GO nanosheets shown in Fig. 4A illustrate the successful preparation of GO by the Hummers method. The GO sheets are measured to range from approximately 1 to 4 μm in lateral dimensions. The characteristics of 2D GO with a slightly curved and crinkled texture are observed. As shown in Fig. 4B, compared with GO sheets, the 2D surface of modified GO has a relatively rough surface after the silica modification reaction. Furthermore, the magnified TEM image of silica modified GO sheets makes clear that GO exhibits a curly morphology with a thicker and wrinkled structure which is very likely due to the modification of silica on the surface of GO (Fig. 4C). The modified GO sheets have more functional groups located on the GO sheets. These functional groups ensure mechanically steady bonding between GO and ions or inorganic particles, which certainly act as anchors for the adsorption of HAuCl4 and Fe(acac)3 in this work (Fig. 4D).
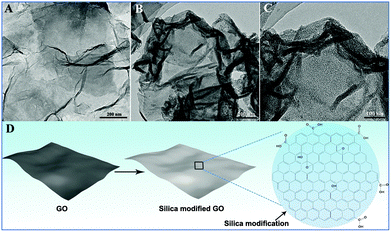 |
| Fig. 4 TEM images of GO (A), low- (B) and high-magnification (C) TEM images of silica modified GO. Schematic illustration of the silica modification process (D). | |
The morphologies of the rGO/Fe3O4 composite and the rGO/Fe3O4/Au composite were determined by SEM and TEM. Fig. 5A demonstrates obviously that Fe3O4 particles have been successfully located onto GO. A high magnification TEM image further reveals that the Fe3O4 particles are well dispersed on the GO sheets, as marked by the red arrow in the corresponding TEM image in Fig. 5B. For the rGO/Fe3O4 composite, GO exhibits a curly morphology with a thin, wrinkled structure. Notably, the decoration of the GO sheets by Fe3O4 particles increases the surface area and avoids the agglomeration of the Fe3O4 particles. Panels C and D in Fig. 5 show the TEM images of the rGO/Fe3O4/Au composite, exhibiting obviously the formation of Au NPs on the surface of rGO after the in situ reduction of HAuCl4. The reduction process by the citrate allows the in situ growth of Au NPs on the graphene sheets and then attachment onto the graphene sheet stably. A high magnification TEM image of the rGO/Fe3O4/Au composite (see in Fig. 5D) further reveals that the Au NPs are spherical in shape and have diameters ranging from 6 nm to 15 nm. Interestingly, the rGO sheets kept spreading well without agglomeration and with a reasonably large surface area which provides a better access for the liquid into the entire structure. In the HRTEM images (Fig. 5E and F), the measured lattice spacings of 0.24 nm and 0.24 nm match well with the d311 spacing of the Fe3O4 phase and d111 spacing of Au, respectively. This is also supported by the corresponding fast Fourier transform (FFT) pattern in the inset. In addition, the corresponding elemental mapping picture displays a clear elemental distribution of C, O, Fe and Au elements (Fig. 5G).
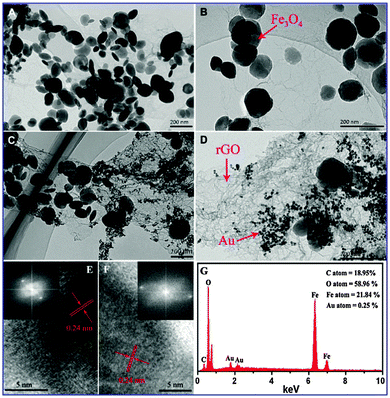 |
| Fig. 5 Low- (A) and high-magnification (B) TEM images of the rGO/Fe3O4 composite, low- (C) and high-magnification (D) TEM images of the rGO/Fe3O4/Au composite, the HRTEM image of the rGO/Fe3O4/Au composite (E and F) and EDS (G). The insets in (E) and (F) are corresponding FFT patterns. | |
Fig. 6 shows the N2 adsorption–desorption isotherms and pore size distribution of the rGO/Fe3O4/Au composite. According to the IUPAC classification, the sample exhibits typical IV type isotherms with an inconspicuous hysteresis loop observed in the range of 0.5–1.0 P/P0, indicating a mesoporous nature. As expected for the observed porous nature, our rGO/Fe3O4/Au composite has a large specific surface area of 102.6 m2 g−1, with a unimodal pore-size of 7 nm calculated by the Barrett–Joiner–Halenda (BJH) method.
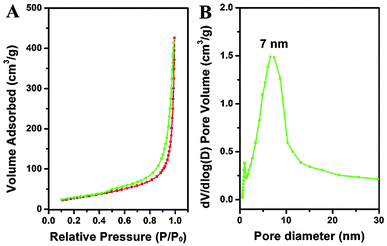 |
| Fig. 6 N2 adsorption/desorption isotherms (A) and particle size distribution (B) of the rGO/Fe3O4/Au composite. | |
The possible mechanism involved in the formation process of the rGO/Fe3O4/Au composite nanostructure is proposed and a representative schematic is illustrated in Scheme 1. Experimentally, the construction of the composite material began with the silica modification of GO. In GO water solution, epoxy and hydroxyl groups were covered on the GO surface, while carbonyl and carboxyl groups were located at the edges. These functional groups acted as anchor sites and enabled the subsequent modification of the GO sheets. With the addition of CTAB, the GO strongly interacted with CTAB molecules and formed the capped GO sheets which can be stabilized in deionized water with excellent dispersibility. Excellent dispersibility played a key role in the following GO modified homogeneously by the silica process which was accomplished by the hydrolysis of TEOS according to the Stöber method. The silica-coated GO sheets effectively avoided the aggregation of the GO sheets. Furthermore, this created negatively charged surface Si–OH groups on GO sheets. Certainly, negative charge groups could adsorb efficiently positive charge ions efficiently in the continued process. Moreover, the large surface area associated with the silica modification ensures the silica surface to immobilize a large number of ions during the following in situ growth process. After the silica modified GO and Fe(acac)3 were mixed with deionized water and reacted under heat treatment conditions, the Fe3O4 seeds were formed on the GO sheets due to the Fe(acac)3 hydrolysis under alkaline conditions and further in situ grown on the GO sheets to form a homogeneous rGO/Fe3O4 composite. Moreover, silica was removed under alkaline conditions after the reaction which is confirmed by the FT-IR spectra of GO, silica modified GO and rGO/Fe3O4/Au in Fig. S2 (ESI†). In Fig. S2 (ESI†), for GO, the strong band of OH (3430 cm−1) and H2O (1620 cm−1) indicates that there are a large number of OH groups and H2O present on the surface. After the silica was modified on GO sheets, several broad absorption bands assigned to OH (3430 cm−1), Si–O–Si (803 cm−1), Si–OH (950 cm−1), and Si–O (455 cm−1) groups are detected, which can be attributed to the amorphous mesoporous silica.16 The strong absorption bands at around 2920 cm−1 and 2850 cm−1 were assigned to the stretching of the C–H bonds of the CTAB molecule residues on the silica. After the hydrothermal reaction, the silica was removed. Notably, GO was reduced to rGO by thermal reduction and most of the oxygen-containing groups on GO sheets are removed. After that, rGO/Fe3O4 was reacted with citrate-capped AuCl4− solution for the reduction of AuCl4− to Au NPs to form the rGO/Fe3O4/Au composite nanostructure. The functional groups present on the surface of GO disappeared for the rGO/Fe3O4/Au sample. The process can be described as an in situ decoration of Au NPs on the surface of rGO/Fe3O4. The two important reactions improve greatly the electrochemical property of the rGO/Fe3O4/Au composite. In addition, citrate served as a reducing agent for the synthesis of Au NPs as well as a chelating ligand with strong coordinating ability to coordinate with Au and rGO/Fe3O4 composites to form stable complexes.
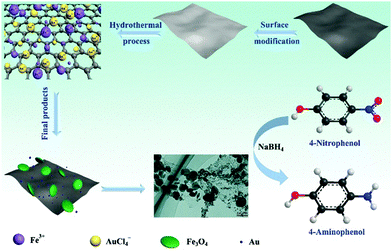 |
| Scheme 1 Schematic illustration of the formation process of the rGO/Fe3O4/Au composite. | |
The rGO/Fe3O4/Au composite obtained by our facile approach shows many unique advantages in comparison with conventional catalysts. Firstly, this advanced in situ growth and reduction method achieved a tight decoration of Fe3O4 and Au NPs on the rGO support; the tight decoration ensures rGO/Fe3O4/Au to keep quite good stability in the catalytic reaction, as well as in magnetic recycling. Secondly, well-crystallized Fe3O4 and Au NPs are evenly prepared and specially dispersed on the rGO supports with a narrow size distribution. This novel structure can effectively prevent nano particles and nano sheets from aggregation in close proximity even at high particle densities. Thirdly, it is feasible to grow two or more kinds of nano particles on the nano sheet surface simultaneously to play different roles, for example in this report, the Au NPs act as high performance catalysts and the decorated Fe3O4 nano particles made the composite convenient for recycling. Consequently, inorganic nanostructured catalysts with a high surface area, magnetic properties, facile regeneration and catalytic performance are reserved, which is important for the super catalytic activity.
3.2. Catalytic performance of the rGO/Fe3O4/Au composite
The reduction of 4-NP to 4-AP by the NaBH4 process is monitored by tracking the changes in the absorbance peak on the UV/vis spectra at different times. The addition of sodium borohydride in 4-NP resulted in a yellow aqueous solution and an absorption maximum located at 400 nm (Fig. 7A–C) due to the formation of 4-nitrophenolate ions. Fig. 7A illustrates a blank test conducted with a mixture of 4-NP and NaBH4, without the rGO/Fe3O4/Au composite. No change in the color of the reaction mixture or the absorption peak intensity was observed even after standing for 12 h, which states clearly that the reduction of 4-NP did not occur without the introduction of the catalyst. After the addition of a small amount (4.0 mg) of the rGO/Fe3O4/Au composite, the reduction commenced and the absorption peak at 400 nm significantly decreased as the reaction proceeds. Meanwhile, a new peak appearing at 295 nm, which corresponds to an increase in 4-AP, reveals surely the reduction of 4-NP to form 4-AP (Fig. 7B). After a 5 min reduction reaction, the pristine bright yellow solution faded to completely colorless and no characteristic absorption of nitro compound could be detected at 400 nm, which proved that the catalytic reduction of 4-NP had proceeded successfully (Fig. 7C). The mechanism of the catalytic reduction of 4-NP to 4-AP is explained in detail in Fig. 8. The catalytic reduction of 4-NP to 4-AP occurred via relaying of electrons from the BH4− donor to the acceptor 4-NP. When the rGO/Fe3O4/Au catalyst composite was added into the reaction mixture, the BH4− ions as well as the 4-NP were adsorbed on the surface of the catalyst and transfer a surface-hydrogen species to the surface of the Au NPs, leading to the rapid occurrence and completion of the reduction reactions. The rate of reduction reaction catalyzed by the rGO/Fe3O4/Au composite was assumed to be independent of the concentration of NaBH4 because the initial concentration of the NaBH4 solution is excess compared to 4-NP. Therefore, a pseudo first-order kinetic equation can be applied to evaluate the catalytic rate, denoted as | −dC/dt = dA/dt = kCt = kAt | (1) |
| ln(Ct/C0) = ln(At/A0) = −kt | (2) |
where Ct is the concentration of 4-NP at time t and k is the apparent rate constant. The ratio of Ct to C0 is measured from the relative intensity of absorbance (At/A0) at 400 nm. Fig. 7D shows the relation of ln(Ct/C0) versus time t. The clearly linear relationship between ln(Ct/C0) and t indicates that the reduction of 4-NP by the rGO/Fe3O4/Au composite matches well with the pseudo-first-order and the rate constant k is calculated to be 0.686 min−1, and the turnover frequency (TOF) of the rGO/Fe3O4/Au composite for the catalytic reaction of 4-NP was calculated to be 716 h−1.
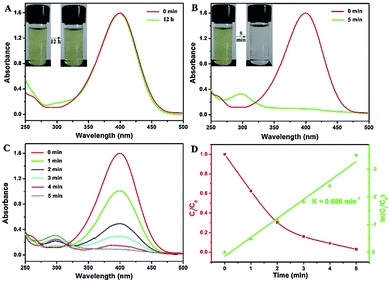 |
| Fig. 7 UV-vis spectra of the catalytic reduction of 4-NP to 4-AP developed at different reaction times when no catalyst was added (A), UV-vis spectra of the catalytic reduction of 4-NP to 4-AP developed at different reaction times using the 4 mg rGO/Fe3O4/Au composite (B); UV-vis spectra of the catalytic reduction of 4-NP to 4-AP developed at different reaction times (C); Ct/C0 and ln(Ct/C0) versus time for the reduction of 4-NP over the 4 mg rGO/Fe3O4/Au catalyst, The ratio of 4-NP concentration (Ct at time t) to its initial value C0 is directly represented by the relative intensity of the respective absorption peak at 400 nm (D). | |
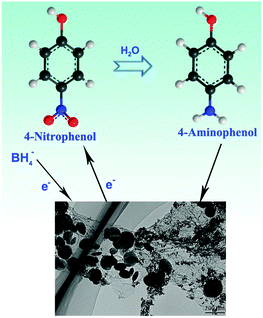 |
| Fig. 8 Mechanism of the catalytic reduction of 4-NP. | |
To investigate the reusability, the rGO/Fe3O4/Au composite was separated using a magnet from the catalytic reaction solution and the cyclic stability was recorded. Fig. 9A shows the magnetization curve of rGO/Fe3O4/Au at room temperature. The saturation magnetization value is 8.0 emu g−1. The inset photograph in Fig. 9A demonstrates the easy separation of rGO/Fe3O4/Au by an external magnetic field in 30 s and states clearly the reusability of the rGO/Fe3O4/Au catalyst. The reusability test result of the rGO/Fe3O4/Au composite, as shown in Fig. 9B, further confirms that the catalyst exhibits a similar catalytic performance without visible reduction (decreased less than 10%) even after running for 8 cycles, which suggested an excellent stability and a long life of the as-prepared rGO/Fe3O4/Au catalyst.
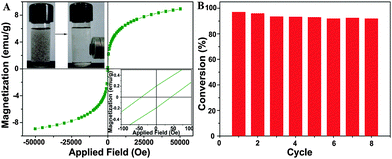 |
| Fig. 9 Magnetization curve of rGO/Fe3O4/Au at room temperature (A), the inset shows the photographs of the magnetic separation. The reusability of the rGO/Fe3O4/Au catalyst (B). | |
The good catalytic activity of the rGO/Fe3O4/Au composite could be explained from three aspects. First, highly dispersed Au NPs were decorated on the surface of rGO sheets to make sure Au NPs exhibit high catalytic activities. In addition, each nanoparticle isolated on rGO sheets has a relatively homogeneous environment around the particle surface thus leading to a high rate of reduction. Second, the good mechanical stability, excellent conductivity properties and large surface area derived from rGO, as well as its porous characteristic are expected to have favorable functions for the excellent catalytic properties of the rGO/Fe3O4/Au composite.
Third, the interaction between Au NPs and rGO supports is more effective than that of the bulk forms, which facilitates the electron transfer between the Au NPs, thus the rGO sheets can provide more active sites to adsorb and reduce 4-NP. Apparently, the synergetic effects between Au NPs and rGO improve catalytic properties of the rGO/Fe3O4/Au composite greatly. Importantly, the good dispersion of Fe3O4 and Au particles is ascribed to the silica-coated GO sheets which can effectively avoid the aggregation of the GO sheets. The abundant surface groups on silica modified GO ensure the surface to immobilize a large number of ions during the particle decorating process. In order to clarify the crucial role the silica modified graphene surface played in the particle decorating process, the rGO/Fe3O4/Au composite using GO only to anchor Fe3O4 and Au was prepared by a similar process without the modification of silica on the GO surface. And, the catalytic property of the composite is demonstrated in Fig. 10 which shows a low performance in the catalytic reduction of 4-NP with conversion of 88% in 15 min. The relatively low catalytic performance of the composite using GO only to anchor Fe3O4 and Au may be ascribed to the morphological properties of the composite. As shown in Fig. 10, in contrast to the good dispersion and a high loading amount of the Fe3O4 and Au particles in the rGO/Fe3O4/Au composite using silica modified GO to anchor particles, agglomeration properties of graphene and low loading of particles are observed for the composite using GO only to anchor Fe3O4 and Au, which may result in the inferior catalytic characteristics.
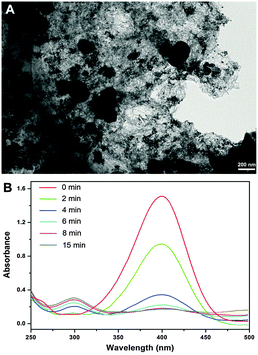 |
| Fig. 10 TEM image of the rGO/Fe3O4/Au composite using GO only to anchor Fe3O4 and Au (A). UV-vis spectra of the catalytic reduction of 4-NP to 4-AP developed at different reaction times for the sample (B). | |
4. Conclusions
In summary, an rGO/Fe3O4/Au composite with highly dispersed Au NPs and Fe3O4 particles has been synthesized with high yield using a facile hydrothermal method firstly. The as-prepared rGO/Fe3O4/Au composite possesses a high surface area, good dispersity of Au NPs and active interaction between Au NPs and rGO supports, thus exhibiting a high catalytic performance demonstrated strongly by the reduction of 4-NP to 4-AP by NaBH4. In addition, the rGO/Fe3O4/Au composite can be conveniently separated from the reaction solution using external magnetic fields and the recovered composite presents excellent reusability. Finally, we believe that the promising synthetic strategy can be extended to develop other graphene based different metal or metallic compound decorated composites for various applications in fields of catalysis, optical devices, sensors and alkaline secondary batteries.
Acknowledgements
Financial support from the National Natural Science Foundation of China (NSFC 61071027) and the Fundamental Research Funds for the Central Universities of China (ZYGX2015KYQD014) is greatly acknowledged.
References
-
(a) C. J. Liu, U. Burghaus, F. Besenbacher and Z. L. Wang, Preparation and characterization of nanomaterials for sustainable energy production, ACS Nano, 2010, 4, 5517–5526 CrossRef CAS PubMed;
(b) L. Jia, Y. L. Zhao and X. J. Liang, Fast evolving nanotechnology and relevant programs and entities in China, Nano Today, 2011, 6, 6–11 CrossRef;
(c) H. L. Wang and H. J. Dai, Strongly coupled inorganic-nano-carbon hybrid materials for energy storage, Chem. Soc. Rev., 2013, 42, 3088–3113 RSC;
(d) A. S. Arico, P. Bruce, B. Scrosati, J. M. Tarascon and W. Van Schalkwijk, Nanostructured materials for advanced energy conversion and storage devices, Nat. Mater., 2005, 4, 366–377 CrossRef CAS PubMed;
(e) V. Biju, Chemical modifications and bioconjugate reactions of nanomaterials for sensing, imaging, drug delivery and therapy, Chem. Soc. Rev., 2014, 43, 744–764 RSC;
(f) X. J. Zhou, J. L. Qiao, L. Yang and J. J. Zhang, A review of graphene-based nanostructural materials for both catalyst supports and metal-free catalysts in PEM fuel cell oxygen reduction reactions, Adv. Energy Mater., 2014, 4, 1301523 Search PubMed.
-
(a) S. J. Guo and E. K. Wang, Noble metal nanomaterials: controllable synthesis and application in fuel cells and analytical sensors, Nano Today, 2011, 6, 240–264 CrossRef CAS;
(b) P. K. Jain, X. H. Huang, I. H. El-Sayed and M. A. El-Sayed, Noble Metals on the Nanoscale: optical and photothermal properties and some applications in imaging, sensing, biology, and medicine, Acc. Chem. Res., 2008, 41, 1578–1586 CrossRef CAS PubMed;
(c) X. Zhang, X. J. Zan and Z. H. Su, Polyelectrolyte multilayer supported Pt nanoparticles as catalysts for methanol oxidation, J. Mater. Chem., 2011, 21, 17783–17789 RSC;
(d) M. Schrinner, M. Ballauff, Y. Talmon, Y. Kauffmann, J. Thun, M. Moller and J. Breu, Single nanocrystals of platinum prepared by partial dissolution of Au-Pt nanoalloys, Science, 2009, 323, 617–620 CrossRef CAS PubMed;
(e) L. Kuai, B. Y. Geng, X. T. Chen, Y. Y. Zhao and Y. C. Luo, Facile subsequently light-induced route to highly efficient and stable sunlight-driven Ag-AgBr plasmonic photocatalyst, Langmuir, 2010, 26, 18723–18727 CrossRef CAS PubMed.
-
(a) R. R. Si, J. F. Liu, K. Yang, X. Chen, W. X. Dai and X. Z. Fu, Temperature-programed surface reaction study of CO oxidation over Au/TiO2 at low temperature: an insight into nature of the reaction process, J. Catal., 2014, 311, 71–79 CrossRef CAS;
(b) L. Q. Nguyen, C. Salim and H. Hinode, Performance of nano-sized Au/TiO2 for selective catalytic reduction of NOx by propene, Appl. Catal., A, 2008, 347, 94–99 CrossRef CAS;
(c) M. Conte, C. J. Davies, D. J. Morgan, T. E. Davies, D. J. Elias, A. F. Carley, P. Johnston and G. J. Hutchings, Aqua regia activated Au/C catalysts for the hydrochlorination of acetylene, J. Catal., 2013, 297, 128–136 CrossRef CAS;
(d) A. Corma and H. Garcia, Supported gold nanoparticles as catalysts for organic reactions, Chem. Soc. Rev., 2008, 37, 2096–2126 RSC;
(e) P. X. Zhao, X. W. Feng, D. S. Huang, G. Y. Yang and D. Astruc, Basic concepts and recent advances in nitrophenol reduction by gold- and other transition metal nanoparticles, Coord. Chem. Rev., 2015, 287, 114–136 CrossRef CAS;
(f) M. D. Hughes, Y. J. Xu, P. Jenkins, P. McMorn, P. Landon, D. I. Enache, A. F. Carley, G. A. Attard, G. J. Hutchings, F. King, E. H. Stitt, P. Johnston, K. Griffin and C. J. Kiely, Tunable gold catalysts for selective hydrocarbon oxidation under mild conditions, Nature, 2005, 437, 1132–1135 CrossRef CAS PubMed;
(g) A. S. K. Hashmi and G. J. Hutchings, Gold catalysis, Angew. Chem., Int. Ed., 2006, 45, 7896–7936 CrossRef PubMed;
(h) L. Kuai, S. Z. Wang and B. Y. Geng, Gold-platinum yolk–shell structure: a facile galvanic displacement synthesis and highly active electrocatalytic properties for methanol oxidation with super CO-tolerance, Chem. Commun., 2011, 47, 6093–6095 RSC.
-
(a) P. Xiao, Y. X. Zhao, T. Wang, Y. Y. Zhan, H. H. Wang, J. L. Li, A. Thomas and J. J. Zhu, Polymeric carbon nitride/mesoporous silica composites as catalyst support for Au and Pt nanoparticles, Chem. – Eur. J., 2014, 20, 2872–2878 CrossRef CAS PubMed;
(b) A. Buonerba, A. Noschese and A. Grassi, Highly efficient direct aerobic oxidative esterification of cinnamyl alcohol with alkyl alcohols catalysed by gold nanoparticles incarcerated in a nanoporous polymer matrix: a tool for investigating the role of the polymer host, Chem. – Eur. J., 2014, 20, 5478–5486 CrossRef CAS PubMed;
(c) M. J. Li, Z. L. Wu and S. H. Overbury, CO oxidation on phosphate-supported Au catalysts: effect of support reducibility on surface reactions, J. Catal., 2011, 278, 133–142 CrossRef CAS;
(d) Y. Mei, Y. Lu, F. Polzer, M. Ballauff and M. Drechsler, Catalytic activity of palladium nanoparticles encapsulated in spherical polyelectrolyte brushes and core–shell microgels, Chem. Mater., 2007, 19, 1062–1069 CrossRef CAS;
(e) Y. Lu, Y. Mei, M. Drechsler and M. Ballauff, Thermosensitive core–shell particles as carriers for Ag nanoparticles: modulating the catalytic activity by a phase transition in networks, Angew. Chem., Int. Ed., 2006, 45, 813–816 CrossRef CAS PubMed;
(f) M. S. Chen and D. W. Goodman, The structure of catalytically active gold on titania, Science, 2004, 306, 252–255 CrossRef CAS PubMed;
(g) E. Lam and J. H. T. Luong, Carbon materials as catalyst supports and catalysts in the transformation of biomass to fuels and chemicals, ACS Catal., 2014, 4, 3393–3410 CrossRef CAS.
-
(a) S. Nardecchia, D. Carriazo, M. L. Ferrer, M. C. Gutierrez and F. del Monte, Three dimensional macroporous architectures and aerogels built of carbon nanotubes and/or graphene: synthesis and applications, Chem. Soc. Rev., 2013, 42, 794–830 RSC;
(b) D. M. Sun, C. Liu, W. C. Ren and H. M. Cheng, A review of carbon nanotube- and graphene-based flexible thin-film transistors, Small, 2013, 9, 1188–1205 CrossRef CAS PubMed.
-
(a) C. C. Huang, C. Li and G. Q. Shi, Graphene based catalysts, Energy Environ. Sci., 2012, 5, 8848–8868 RSC;
(b) S. Stankovich, D. A. Dikin, G. H. B. Dommett, K. M. Kohlhaas, E. J. Zimney, E. A. Stach, R. D. Piner, S. T. Nguyen and R. S. Ruoff, Graphene-based composite materials, Nature, 2006, 442, 282–286 CrossRef CAS PubMed;
(c) X. Z. Zhou, X. Huang, X. Y. Qi, S. X. Wu, C. Xue, F. Y. C. Boey, Q. Y. Yan, P. Chen and H. Zhang,
In situ synthesis of metal nanoparticles on single-layer graphene oxide and reduced graphene oxide surfaces, J. Phys. Chem. C, 2009, 113, 10842–10846 CrossRef CAS;
(d) Z. Hu, Y. D. Huang, S. F. Sun, W. C. Guan, Y. H. Yao, P. Y. Tang and C. Y. Li, Visible light driven photodynamic anticancer activity of graphene oxide/TiO2 hybrid, Carbon, 2012, 50, 994–1004 CrossRef CAS;
(e) Z. Hu, J. Li, C. Y. Li, S. J. Zhao, N. Li, Y. F. Wang, F. Wei, L. Chen and Y. D. Huang, Folic acid-conjugated graphene-ZnO nanohybrid for targeting photodynamic therapy under visible light irradiation, J. Mater. Chem. B, 2013, 1, 5003–5013 RSC.
-
(a) H. P. Cong, J. F. Chen and S. H. Yu, Graphene-based macroscopic assemblies and architectures: an emerging material system, Chem. Soc. Rev., 2014, 43, 7295–7325 RSC;
(b) Q. J. Xiang, J. G. Yu and M. Jaroniec, Enhanced photocatalytic H-2-production activity of graphene-modified titania nanosheets, Nanoscale, 2011, 3, 3670–3678 RSC;
(c) Y. J. Hu, J. A. Jin, P. Wu, H. Zhang and C. X. Cai, Graphene-gold nanostructure composites fabricated by electrodeposition and their electrocatalytic activity toward the oxygen reduction and glucose oxidation, Electrochim. Acta, 2010, 56, 491–500 CrossRef CAS;
(d) C. Nethravathi, M. Rajamathi, N. Ravishankar, L. Basit and C. Felser, Synthesis of graphene oxide-intercalated alpha-hydroxides by metathesis and their decomposition to graphene/metal oxide composites, Carbon, 2010, 48, 4343–4350 CrossRef CAS;
(e) X. Huang, X. Y. Qi, F. Boey and H. Zhang, Graphene-based composites, Chem. Soc. Rev., 2012, 41, 666–686 RSC;
(f) X. Huang, Z. Y. Yin, S. X. Wu, X. Y. Qi, Q. Y. He, Q. C. Zhang, Q. Y. Yan, F. Boey and H. Zhang, Graphene-based materials: synthesis, characterization, properties, and applications, Small, 2011, 7, 1876–1902 CrossRef CAS PubMed;
(g) Y. J. Hu, H. Zhang, P. Wu, H. Zhang, B. Zhou and C. X. Cai, Bimetallic Pt-Au nanocatalysts electrochemically deposited on graphene and their electrocatalytic characteristics towards oxygen reduction and methanol oxidation, Phys. Chem. Chem. Phys., 2011, 13, 4083–4094 RSC;
(h) H. B. Wang, T. Maiyalagan and X. Wang, Review on recent progress in nitrogen-doped graphene: synthesis, characterization, and its potential applications, ACS Catal., 2012, 2, 781–794 CrossRef CAS.
-
(a) C. Z. Zhu and S. J. Dong, Recent progress in graphene-based nanomaterials as advanced electrocatalysts towards oxygen reduction reaction, Nanoscale, 2013, 5, 1753–1767 RSC;
(b) G. P. Singh, K. M. Shrestha, A. Nepal, K. J. Klabunde and C. M. Sorensen, Graphene supported plasmonic photocatalyst for hydrogen evolution in photocatalytic water splitting, Nanotechnology, 2014, 25, 11 Search PubMed;
(c) H. Zhang, S. Chen, X. Quan, H. T. Yu and H. M. Zhao,
In situ controllable growth of noble metal nanodot on graphene sheet, J. Mater. Chem., 2011, 21, 12986–12990 RSC;
(d) E. O. Pentsak, E. G. Gordeev and V. P. Ananikov, Noninnocent nature of carbon support in metal/carbon catalysts: etching/pitting vs nanotube growth under microwave irradiation, ACS Catal., 2014, 4, 3806–3814 CrossRef CAS.
- J. Li, C. Y. Liu and Y. Liu, Au/graphene hydrogel: synthesis, characterization and its use for catalytic reduction of 4-nitrophenol, J. Mater. Chem., 2012, 22, 8426–8430 RSC.
- H. Q. Yao, T. C. Huang and H. J. Sue, Self-assembly of Au nanoparticles on graphene, RSC Adv., 2014, 4, 61823–61830 RSC.
-
(a) Y. Y. Liang, H. L. Wang, J. G. Zhou, Y. G. Li, J. Wang, T. Regier and H. J. Dai, Covalent hybrid of spinel manganese-cobalt oxide and graphene as advanced oxygen reduction electrocatalysts, J. Am. Chem. Soc., 2012, 134, 3517–3523 CrossRef CAS PubMed;
(b) Y. G. Li, H. L. Wang, L. M. Xie, Y. Y. Liang, G. S. Hong and H. J. Dai, MoS2 Nanoparticles grown on graphene: an advanced catalyst for the hydrogen evolution reaction, J. Am. Chem. Soc., 2011, 133, 7296–7299 CrossRef CAS PubMed;
(c) Y. Y. Liang, Y. G. Li, H. L. Wang, J. G. Zhou, J. Wang, T. Regier and H. J. Dai, Co3O4 nanocrystals on graphene as a synergistic catalyst for oxygen reduction reaction, Nat. Mater., 2011, 10, 780–786 CrossRef CAS PubMed.
- H. L. Poh, F. Sanek, A. Ambrosi, G. J. Zhao, Z. Sofer and M. Pumera, Graphenes prepared by staudenmaier, hofmann and hummers methods with consequent thermal exfoliation exhibit very different electrochemical properties, Nanoscale, 2012, 4, 3515–3522 RSC.
- D. C. Marcano, D. V. Kosynkin, J. M. Berlin, A. Sinitskii, Z. Z. Sun, A. Slesarev, L. B. Alemany, W. Lu and J. M. Tour, Improved synthesis of graphene oxide, ACS Nano, 2010, 4, 4806–4814 CrossRef CAS PubMed.
- J. W. Lee, A. S. Hall, J. D. Kim and T. E. Mallouk, A facile and template-free hydrothermal synthesis of Mn3O4 nanorods on graphene sheets for supercapacitor electrodes with long cycle stability, Chem. Mater., 2012, 24, 1158–1164 CrossRef CAS.
- M. Razaul. Karim, H. Shinoda, M. Nakai, K. Hatakeyama, H. Kamihata, T. Matsui, T. Taniguchi, M. Koinuma, K. Kuroiwa, M. Kurmoo, Y. Matsumoto and S. Hayami, Electrical conductivity and ferromagnetism in a reduced graphene–metal oxide hybrid, Adv. Funct. Mater., 2012, 23, 323–332 CrossRef.
- X. J. Kang, Z. Y. Cheng, D. M. Yang, P. A. Ma, M. M. Shang, C. Peng, Y. L. Dai and J. Lin, Design and synthesis of multifunctional drug carriers based on luminescent rattle-type mesoporous silica microspheres with a thermosensitive hydrogel as a controlled switch, Adv. Funct. Mater., 2012, 22, 1470–1481 CrossRef CAS.
Footnote |
† Electronic supplementary information (ESI) available. See DOI: 10.1039/c5cp05336f |
|
This journal is © the Owner Societies 2016 |