DOI:
10.1039/C5BM00235D
(Paper)
Biomater. Sci., 2016,
4, 104-114
Dual acid-responsive supramolecular nanoparticles as new anticancer drug delivery systems†
Received
8th July 2015
, Accepted 7th September 2015
First published on 6th October 2015
Abstract
Considering the specific pH gradients of tumour microenvironments, a dual acid-responsive drug delivery system, which can respond to the tumor extracellular and intercellular pH stimuli, has been fabricated via simple host–guest recognition. Firstly, we synthesise 2,4,6-trimethoxybenzaldehyde modified dextran (Dex-TMBA) and mPEG-imine-β-cyclodextrin (PIC), respectively. And then, through the host–guest recognition between the cyclodextrin (CD) of PIC and the benzene ring of Dex-TMBA, a kind of dual acid-responsive supramolecular drug delivery system can be fabricated. Under neutral pH conditions, anticancer drugs can be loaded by forming supramolecular nanoparticles via the host–guest recognition. While, at tumor extracellular pH (∼6.8), the acid-labile benzoic–imine of PIC cleaves and the nanoparticles are amino positively charged to facilitate cell internalization. Subsequently, due to the hydrolysis of acetal bonds in Dex-TMBA under significantly increased acidity in subcellular compartments such as the endosomes (∼5.3), the loaded doxorubicin releases from the endocytosed drug delivery. This dual acid-responsive nanoparticles can efficiently load and release drugs, acting as drug delivery systems for enhancing anticancer efficiency.
1 Introduction
In the clinical diagnosis and treatment of tumors, it is desirable to design nanocarriers which can selectively target diseased sites to improve the therapeutic efficiency and eliminate the adverse effects on the organisms.1–4 Recently, the persistent development of stimuli-responsive polymeric carriers for intracellular drug delivery makes them promising in clinical therapies. Stimuli-responsive delivery systems, which can undergo chemical or physical transformations once exposed to tumor microenvironments or internal stimuli such as pH,5 redox,6 temperature,7 enzymes, etc.8 have emerged as a smart and powerful strategy for targeting delivery and controlled release.
Among the various stimuli, variation in pH is the most frequently used, as pH values in different tissues and cellular compartments vary tremendously. For example, the tumor extracellular environment is more acidic (pHe 6.5) than blood and normal tissues (pH 7.4), and the pH values of endosomes/lysosomes are even lower at 5.0–5.5.9 According to the tumor extracellular and intracellular pH gradients, dual pH-responsive drug delivery systems which can respond to not only pHe but also endosome/lysosome pH have been developed and exhibit great superiority in controlled release and targeted delivery of drugs, which are expected to simultaneously promote drug accumulation at the tumor site via the enhanced permeability and retention (EPR) effect and facilitate the cell internalization and intracellular drug release, greatly enhancing the drug delivery efficacy.10–14 The Wang group has designed a tailor-made dual pH-sensitive polymer drug conjugate nanoparticulate system, which is capable of reversing its surface charge from negative to positive at tumor extracellular pH (∼6.8) to facilitate cell internalization.10 Subsequently, the significantly increased acidity in subcellular compartments such as the endosome (∼5.0) further promotes doxorubicin release from the endocytosed drug carriers. This dual pH-sensitive nanoparticle has shown enhanced cytotoxicity in drug resistant cancer stem cells, indicating its great potential for cancer therapy. Zhang et al. have designed a dual-pH sensitive nanocarrier based on negatively charged PLLeu–PLL(DMA)–Tat(SA) (PPDTS). The amides would be hydrolyzed once the nanoparticles reach the mildly acidic tumor extracellular environment (pH ≈ 6.5) and the micelles would switch to positive charge again and be quickly internalized by tumor cells. By simply modifying the lysine amino residues of polylysine and Tat with two different amidating reagents, respectively, this nanocarrier will facilitate the tumor targeting and nuclear delivery simultaneously.11
Usually, some acid-labile chemical bonds, such as maleic acid amide,1 acetal,15 hydrazone,16cis-acony,17 orthoester18 and benzoic imine,19 are employed to construct the above-mentioned pH-responsive drug delivery systems. Compared with conventional covalent bonds, the non-covalent supramolecular interactions such as multiple hydrogen bonding,20 host–guest recognition,21 hydrophobic interaction,22 π–π stacking23 and metal–ligand coordination,24 show more convenient synthesis procedure. Hence, supramolecular nanoparticles formed by non-covalent interactions have attracted more extensive attention to fabricate intelligent nanocarriers for anticancer drug delivery.23,25–27 Among the various non-covalent physical interactions, host–guest interaction is a prominent approach widely used to fabricate nanoparticles for drug delivery. Generally, cyclodextrin,28 cucurbituril29 and calixarene30 are adopted as receptor molecules (host) and small molecules,28,29 polymer chains,31 and cationic or anionic guests32 as ligand container molecules (guest) because of their insignificant toxicity and bioavailability.33 The Li group has designed a new type of acid-sensitive polypseudorotaxanes (PPRs) based on ortho ester-modified cyclodextrin (EMD-CD) and pluronic F-127. The water-insoluble EMD-CD (host) is capable of forming PPRs with F127 (guest) in ethanol or methanol. These PPR assemblies were stable at pH 8.4 but quickly dissociated in biocompatible products under neutral or acidic conditions due to the hydrolysis of the ortho ester group. The great pH-sensitive character, good biocompatibility, and ease of fabrication make the PPRs promising candidates for drug delivery.25
Herein, taking advantage of the specific pH gradients of tumour microenvironments, we designed a kind of dual pH-responsive nanocarrier for anticancer drugs which could greatly enhance the drug uptake efficiency by tumor cells. We chose dextran as the backbone. It is known that dextran is a kind of water-soluble natural polysaccharide with unique properties, such as excellent biocompatibility, wide availability and nonfouling property. Moreover, due to the plentiful hydroxyl groups on the branching chain, dextran can easily be chemically modified.34 To fabricate dual pH-sensitive nanocarriers, 2,4,6-trimethoxybenzaldehyde modified dextran (Dex-TMBA) and mPEG-imine-β-cyclodextrin (PIC) are synthesized, respectively. Under the physical neutral pH conditions, the cyclodextrin (CD) of PIC associate with the benzene ring of Dex-TMBA by host–guest recognition to load anticancer drugs, while disassemble in the tumor extracellular environment and subcellular compartments step by step (Scheme 1). First of all, due to the cleavage of the acid-labile benzoic–imine of PIC at tumor extracellular pH (∼6.8), the nanoparticles will be amino positively charged to facilitate cell internalization, accordingly regulate the quantity and percent of nanocarriers entering tumor cells and subsequently, the hydrolysis of acetal bonds in Dex-TMBA under significantly increased acidity in subcellular compartments such as the endosome (∼5.3) will result in the release of the loaded doxorubicin from the endocytosed drug delivery system. The dual pH-responsive supramolecular nanocarriers for anticancer drugs could overcome the shortcomings of single pH-responsive, disulfide linkage etc. and other sensitive drug delivery systems with higher anticancer efficacy, and were fabricated by flexible and easy supramolecular interactions. These dual acid-responsive nanoparticles can efficiently load and release drugs, acting as drug delivery systems for enhancing anticancer efficiency.
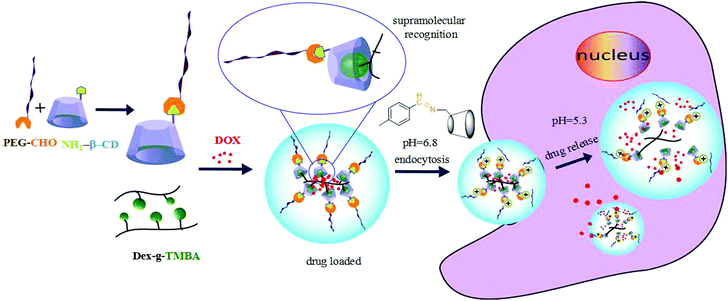 |
| Scheme 1 Schematic illustration of DOX loading and intracellular triggered release from DOX-loaded Dex-TMBA/PIC dual pH responsive supramolecular nanoparticles. | |
2. Materials and methods
2.1 Reagents and materials
Dextran (Dex, Mn = 40 kDa) and monomethoxy poly(ethylene glycol) (mPEG, Mn = 5000) were purchased from Sigma-Aldrich and used as received. 2,4,6-Trimethoxybenzaldehyde, succinic anhydride and 4-formylbenzoic acid were bought from Aladdin. Amino-β-cyclodextrin (β-CD-NH2) was bought from Shandong Binzhou Zhiyuan Biotechnology Co., Ltd). Benzoic acid, 4-dimethylaminopyridine (DMAP), N-(3-dimethylaminopropyl) N′-ethylcarbodiimide hydrochloride (EDC·HCl), N-hydroxysuccinimide (NHS) and p-toluenesulfonic acid (PTSA) were bought from Sigma-Aldrich and used directly. Doxorubicin hydrochloride (DOX·HCl) was purchased from Zhejieng Hisun Pharmaceutical Co., Ltd. All cells were purchased from Tong Pai Biotechnology Co., Ltd. (Shanghai China).
2.2 Characterization
1H NMR spectra were recorded on a Bruker AV 400 NMR spectrometer in dimethyl sulfoxide-d6 (DMSO-d6). Fourier Transform Infrared (FTIR) spectra were obtained using a Bio-Rad Win-IR instrument. The size and distribution of particles were tested by using a WyattQELS dynamic laser scattering (DLS) instrument with a vertically polarized He–Ne laser (DAWN EOS, Wyatt Technology). Transmission electron microscopy (TEM) images were taken by using a JEOL JEM-1011 transmission electron microscope with an accelerating voltage of 100 kV. Flow cytometry (Coulter Epice XL, Beckman Coulter, USA) was performed to quantitatively determine the percentage of cells. Confocal laser scanning microscopy (CLSM) was carried out by using an Olympus FluoView 1000. A Bio-Rad 680 microplate reader was used to conduct MTT.
2.3 Synthesis of 2,4,6-trimethoxybenzaldehyde modified dextran (Dex-TMBA)
2,4,6-Trimethoxybenzaldehyde (1.21 g, 6.17 mmol) and dextran (1.00 g, 0.025 mmol) were dissolved in 30 mL of DMSO in a glass ampoule with magnetic stirring. Then p-toluenesulfonic acid (0.202 g, 1.17 mmol) was added into the mixture at 80 °C for 72 h. After the reaction was complete, the solvent was precipitated in 10-fold acetone to obtain the crude product. Then, the crude product re-dissolved in deionized water, and dialysed against the deionized water for 3 d. The solution was then lyophilized and a light yellow solid product was finally obtained (yield: 74.6%).
2.4 Synthesis of benzoic acid modified dextran (Dex-BA)
Dex-BA was synthesized as a pH-insensitive control group. Dextran (1.00 g, 0.025 mmol), benzoic acid (0.52 g, 4.262 mmol), EDC·HCl (1.52 g, 7.958 mmol) and DMAP (0.07 g, 0.574 mmol) were dissolved in 30 mL of DMSO in a glass ampoule with a magnetic bar. The reaction was performed at 25 °C for 48 h. Then, the solvent and the unreacted substances were removed by dialysis against deionized water for 3 d. The solution was lyophilized, to finally give a white solid product (yield: 84.6%).
2.5 Synthesis of aldehyde mPEG (mPEG-CHO)
mPEG (1 g, 0.2 mmol), 4-formylbenzoic acid (0.03 g, 0.2 mmol), EDC (0.077 g, 0.4 mmol) and DMAP (0.004 g, 0.029 mmol) were dissolved in methyl trichloride (20 mL) in a glass ampoule with a magnetic bar. The reaction mixture was stirred at room temperature for 48 h, after the reaction was complete, the solution and the unreacted substances were removed by dialysis for 3 days (MWCO 3.5 kDa), the product was collected by lyophilization (yield: 91%).
2.6 Synthesis of carboxylated mPEG (mPEG-COOH)
mPEG (2 g, 0.4 mmol), succinic anhydride (0.12 g, 1.2 mmol) and DMAP (0.049 g, 0.4 mmol) were dissolved in 50 mL of methyl trichloride at 50 °C, then methanol (0.08 g, 2.4 mmol) was added to the solvent. After reacting for 48 h, the mixture was washed with 6 mL of saturated NaCl three times, after drying with anhydrous MgSO4, the solution precipitated in diethyl ether and was dried under vacuum (yield: 92.6%).
2.7 Synthesis of mPEG-imine-β-cyclodextrin (PIC)
mPEG-CHO (1 g, 0.195 mmol) and amino-β-cyclodextrin (β-CD-NH2, 0.224 g, 0.195 mmol) were dissolved in 15 mL of DMF in a glass ampoule with a magnetic bar. The reaction was performed at 30 °C for 48 h. Then, the solvent and the unreacted substances were removed by dialysis against deionized water for 3 days (MWCO 3.5 kDa). The solution was lyophilized, to finally give a light yellow solid (yield: 90%).
2.8 Synthesis of mPEG-β-cyclodextrin (PC)
mPEG-COOH (1 g, 0.195 mmol), amino-β-cyclodextrin (β-CD-NH2) (0.225 g, 0.195 mmol), EDC·HCl (0.075 g, 0.39 mmol), and NHS (0.045 g, 0.39 mmol), were dissolved in 25 mL of DMF in a glass ampoule with a magnetic bar. The reaction was performed at 30 °C for 48 h. Then, the solvent and the unreacted substances were removed by dialysis against deionized water for 3 d (MWCO 3.5 kDa). The solution was lyophilized, to finally give a light yellow solid (yield: 94.5%).
2.9 Preparation of supramolecular nanoparticles (Dex-TMBA/PIC, Dex-BA/PIC and Dex-BA/PC)
Dex-TMBA (0.1 g, 0.002 mmol) and PIC 0.05 g, 0.0079 mmol) were dissolved in 5.0 mL of DMSO and dropped into 50 mL PBS at pH 7.4. Then the solution was dialyzed against deionized water for 12 h to remove DMSO (MWCO 3.5 kDa). The final product was obtained by lyophilization. Dex-BA/PIC and Dex-BA/PC were synthesized in the similar way as Dex-TMBA/PIC.
2.10
In vitro drug loading and release
Doxorubicin (DOX) was chosen as a kind of model anticancer drug for in vitro drug loading and release. DOX-loaded nanoparticles were prepared by a simple dialysis technique.35 Typically, Dex-TMBA (20.0 mg), DOX·HCl (5.0 mg), and PIC (12 mg) were mixed in 2.0 mL of DMSO. The mixture was stirred at room temperature for 24 h, and then was dropwise added into 20.0 mL of PBS at pH 7.4. The DMSO and free DOX was removed by dialyzing against deionized water for 12 h at room temperature (MWCO = 3500 Da). The dialysis media was refreshed four times and the whole procedure was performed in the dark. Finally, the mixture was filtered and freeze-dried to obtain DOX-loaded Dex-TMBA/PIC. Similarly, DOX-loaded Dex-BA/PC and DOX-loaded Dex-BA/PIC were obtained following the above procedure. To determine the drug loading content (DLC) and the drug loading efficiency (DLE), DOX-loaded nanoparticles were dissolved in DMSO and analyzed by fluorescence measurements (Perkin-Elmer LS50B luminescence spectrometer). A standard curve (λex = 480 nm) was obtained from different DOX concentrations.33 The DLC and DLE were calculated according to the following equations: |  | (1) |
|  | (2) |
In vitro DOX release behaviour from DOX-loaded nanoparticles was investigated in PBS at three different pH gradients of 5.5, 6.8 and 7.4, respectively. The pre-weighed lyophilized DOX-loaded nanoparticles were suspended in 3 mL of release media, and then transferred into a dialysis tube (MWCO = 3500 Da). The release experiment was initiated by placing an end-sealed dialysis bag into 50 mL of PBS at 37 °C with continuous shaking at around 100 rpm. At definite time intervals, 2.0 mL of dialysate was taken out and an equal volume of fresh buffer was added.36 The amount of released DOX was measured by fluorescence measurement (λex = 480 nm). The release experiments were conducted in triplicate.
2.11 Intracellular drug release
The cellular uptake and intracellular release activities of DOX-loaded nanoparticles were observed by confocal laser scanning microscopy (CLSM) and flow cytometry analyses on HeLa cells.
Confocal laser scanning microscopy (CLSM).
For this study, HeLa cells were seeded in six-well plates at a density of 2 × 105 cells per well in 2.0 mL of complete DMEM (Dulbecco's modified Eagle's medium) containing 10% fetal bovine serum, supplemented with 50 IU mL−1 penicillin and 50 IU mL−1 streptomycin. After incubation for 24 h, the culture media were withdrawn and the cells were incubated with DOX-loaded nanoparticles at a final DOX concentration of 10.0 mg L−1 in complete DMEM for an additional 3 h at 37 °C and 4 °C, respectively. Then the culture medium was removed and the cells were washed with PBS three times. Thereafter, the cells were fixed with 4% paraformaldehyde for 30 min at room temperature, and the cell nuclei were stained with 4′,6-diamidino-2-phenylindole (DAPI, blue) for 20 min. The image of the cells was obtained through a confocal microscope (Olympus FluoView 1000).
For subcellular distribution observation, the cells were seeded according to the above mentioned description at 37 °C. After incubation with DOX-loaded Dex-TMBA/PIC, DOX-loaded Dex-BA/PIC and DOX-loaded Dex-BA/PC at a final DOX concentration of 10.0 mg L−1 in complete DMEM at pH 6.8 for 3 h, the medium was removed and the cells were washed with PBS and fixed with 4% paraformaldehyde for 30 min at room temperature. The cell nuclei were stained with DAPI. The CLSM observation was the same as above.
Flow cytometry analyses.
HeLa cells were seeded into 6-well plates at 2 × 105 cells per well in 2.0 mL of complete DMEM and cultured for 24 h. The cells were incubated at 37 °C for an additional 3 h with DOX-loaded nanoparticles at a final DOX concentration of 10.0 mg L−1. Thereafter, the culture medium was removed and the cells were washed with PBS thrice and treated with trypsin. Then, 2.0 mL of PBS was added to each culture well, and the solutions were centrifuged for 4 min at 3000 rpm. After the removal of supernatants, the cells were re-suspended in 0.3 mL of PBS. The flow cytometry analyses against HeLa cells at pH 6.8 culture medium were carried out in the same way as above. Data for 1 × 104 gated events were collected, and analysis was performed by using a flow cytometer (Beckman, California, USA).
Cell viability assays.
The relative cytotoxicity of DOX-free nanoparticles against HeLa and HepG2 cells was evaluated in vitro using a standard MTT assay. The cells were seeded in 96-well plates at 1 × 104 cells per well in 200.0 μL of complete DMEM and incubated at 37 °C under 5% CO2 atmosphere for 24 h. The culture medium was then removed and the nanoparticle solutions in complete DMEM with different concentrations (0–50 mg L−1) were added. The cells were subjected to the MTT assay after incubation for an additional 48 h. The absorbance of the solution was measured on a Bio-Rad 680 microplate reader at 490 nm. Cell viability (%) was calculated by the following equation: | Cell viability (%) = Asample/Acontrol × 100 | (3) |
where Asample and Acontrol represent the absorbance of the sample and control wells, respectively.
The cytotoxicity of DOX-loaded nanoparticles against HepG2 and HeLa cells was also evaluated in vitro by a standard MTT assay. Similarly, cells were seeded into 96-well plates at 1 × 104 cells per well in 200.0 μL of complete DMEM and further incubated at 37 °C for 24 h. After washing the cells with PBS, 180.0 μL of complete DMEM and 20.0 μL of DOX-loaded nanoparticles solutions in PBS were added to form culture media of different DOX concentrations (0–10.0 mg L−1 DOX). The cells were subjected to the MTT assay after incubation for 24, 48 and 72 h. And the relative cytotoxicity of DOX-loaded nanoparticles against HepG2 and HeLa cells at pH 6.8 culture medium was evaluated with the same procedure as above. The absorbance was measured on a Bio-Rad 680 microplate reader at 490 nm. Cell viability (%) was also calculated based on eqn (3).
3. Results and discussion
3.1 Synthesis of Dex-TMBA
The pH-sensitive acetalated dextran was synthesized as shown in Scheme 2, the ortho-hydroxyl of glucose molecules can react with the aldehyde of TMBA to give Dex-TMBA. The chemical structure of Dex-TMBA was confirmed by 1H-NMR (Fig. 1) and FT-IR (Fig. S1†). As shown in Fig. 1, the appearance of the characteristic peaks of cyclic benzylidene acetal at 5.6 ppm (a) and phenyl ring protons at 6.4 ppm (b) strongly indicated the successful synthesis of Dex-TMBA. The proton peaks of the dextran unit appearing at 3.0–5.0 ppm (c–j′) further confirmed the structure of Dex-TMBA. The appearance of the benzene ring at 1568 cm−1 further confirmed the successful synthesis of the desired Dex-TMBA (Fig. S1†). The modified molar ratio of TMBA and Dex was 0.54
:
1, which was calculated by measuring the absorbance at 290 nm of UV/vis spectroscopy.36
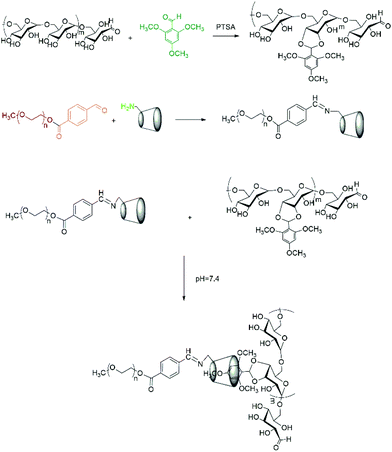 |
| Scheme 2 Synthetic route for Dex-TMBA/PIC. | |
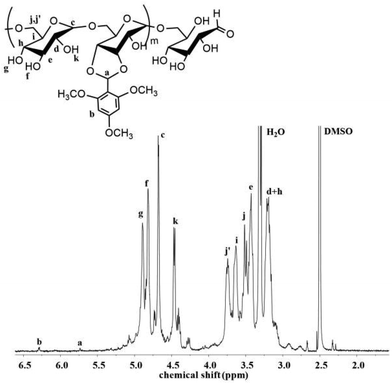 |
| Fig. 1
1H NMR spectrum of Dex-TMBA in DMSO-d6. | |
3.2 Synthesis of Dex-BA
Here, Dex-BA was synthesized as a pH-insensitive control group (Scheme S1†). As observed in the FTIR spectra (Fig. S1†), the characteristic peak appearing at 1720 cm−1, attributed to the C
O group, demonstrated the successful synthesis of Dex-BA. In addition, the peak at 7.5–8.2 ppm assigned to the aromatic protons, distinctly demonstrated that dextran was successfully modified by BA (Fig. S2†). Similarly, the modified molar ratio of BA and Dex was 0.71
:
1, which was calculated by measuring the absorbance at 290 nm of UV/vis spectroscopy.
3.3 Synthesis of PIC
mPEG-imine-β-cyclodextrin (PIC) was prepared as a pH-sensitive unit at pH 6.8 by a Schiff's reaction between the benzaldehyde of mPEG-CHO and the amine group of β-CD-NH2 as described in Scheme 2. According to FT-IR (Fig. S1†), the adsorption peaks at 1710 cm−1 assigned to the imine bond demonstrated the structure of PIC. At the same time, the characteristic signals of benzoic imine protons at 8.1 ppm (c) and the absence of the benzene group peak (a + b) clearly indicated the successful synthesis of PIC (Fig. 2).
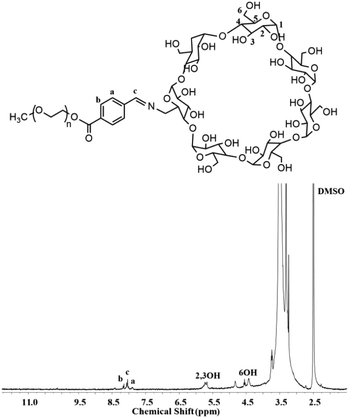 |
| Fig. 2
1H NMR spectrum of PIC in DMSO-d6. | |
3.4 Synthesis of PC
mPEG-β-cyclodextrin (PC) was synthesized as pH-insensitive control (Scheme S2†). Compared with the acid-labile benzoic imine linker, the amide bond conjugated between mPEG-COOH and β-CD-NH2 is stable at pH 6.8.
The structure of PC was also confirmed by FT-IR and 1H NMR (Fig. S1 and S3†). The peaks at 1654 cm−1 and 1737 cm−1 belonged to the C
O stretching vibration in the carboxyl group and the N–H bending vibration strongly suggested the successful synthesis of PC (Fig. S1†). In the 1H NMR spectrum of PC (Fig. S3†), the appearance of proton peaks at 7.7 ppm (b) assigned to the amide bond and the signals of cyclodextrin at 4.0–6.3 ppm also obviously suggested the successful synthesis of PC.
3.5 Characterization of supramolecular nanoparticles
The supramolecular nanoparticles were fabricated via the simple host–guest recognition between β-CD and benzene ring. To confirm the dual acid-responsive property of Dex-TMBA/PIC (at pH 6.8 and 5.3), the other supramolecular nanoparticles, pH-insensitive Dex-BA/PC and single pH-sensitive Dex-BA/PIC (at pH 6.8), were designed as compare groups.
In order to demonstrate the successful association between Dex-TMBA and PIC by host–guest interaction, the mixtures of Dex-TMBA and PIC with different ratios were dissolved in 2.5 mL of phosphate buffered saline (PBS). The fluorescence of the solution is demonstrated in Fig. 3. Obviously, Dex-TMBA showed dual fluorescence in PBS at 265 and 320 nm, the higher emission at 265 nm was assigned to the neutral form of TMBA. With the gradual addition of PIC into Dex-TMBA, the fluorescence intensity of Dex-TMBA increased significantly. These changes were induced by the host–guest interaction between the β-CD host and the TMBA guest. The restricted geometry of β-CD, especially its hydrophobic cavity offered a suitable environment for TMBA to enter, resulting in the increasing fluorescence intensity and protecting Dex-TMBA from the quenching effect. These results suggested the successful supramolecular association between Dex-TMBA and PIC.
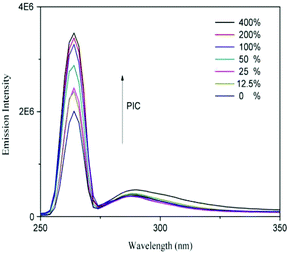 |
| Fig. 3 Fluorescence emission spectra of Dex-TMBA in PBS solution with different PIC concentrations. The concentration of Dex-TMBA was set at 1 mg mL−1. | |
The self-assembly between Dex-TMBA and PIC was also confirmed by fluorescence spectra using Nile Red as the fluorescent probe in phosphate buffer at pH 7.4, according to the literature method.37 The critical micelle concentration (CMC) are collected by tracking the fluorescence intensity of Nile Red as a function of the sample concentration. As shown in Table 1, Dex-TMBA/PIC supramolecular nanoparticles showed a higher CMC value of 37.4 mg L−1. While, the CMC values of Dex-BA/PC and Dex-BA/PIC were 31.1 mg L−1 and 25.2 mg L−1, respectively, a little lower than that of Dex-TMBA/PIC because benzoic acid was more hydrophobic than 2,4,6-trimethoxybenzaldehyde at pH 7.4 and more easily assembles in a neutral environment.
Table 1 Characterization of supramolecular nanoparticles
Sample |
Feed ratio (mg : mg) |
CMCa (mg L−1) |
DLSa (nm) |
DLC (wt%) |
DLE (wt%) |
Determined in PBS at pH 7.4 at room temperature.
|
Dex-TMBA/PIC |
Dex-TMBA : PIC = 100 : 50 |
37.4 |
97 ± 9 |
4.73 |
26.01 |
Dex-BA/PIC |
Dex-BA : PIC = 100 : 56 |
25.2 |
93 ± 5 |
6.28 |
44.47 |
Dex-BA/PC |
Dex-BA : PC = 100 : 56 |
31.1 |
95 ± 6 |
6.06 |
43.25 |
To demonstrate the pH-sensitivity of Dex-TMBA/PIC, the hydrodynamic radii (Rh) were measured by DLS measurements at different pH values. As shown in Fig. 4, the average size of Dex-TMBA/PIC was around 97 nm at pH 7.4 (A). However, the particle size significantly increased to around 247 nm at pH 5.3 (B) due to the acid-sensitive property of the cyclic benzylidene acetal bond. In an acidic environment, the cyclic benzylidene acetal bond would cleave and the supramolecular nanoparticles would expand or even completely dissemble. The same results were also confirmed by transmission electron microscopy (TEM); the supramolecular nanoparticles were spherical with an average diameter of 90 nm at pH 7.4 (C). On the contrary, the average diameter increased to 245 nm at pH 5.3 (D). Compared with the results of DLS, the obtained smaller size might be due to the dehydration of nanoparticles when preparing the sample. Both DLS and TEM results confirmed that Dex-TMBA/PIC could not only self-assemble into supramolecular nanoparticles but also exhibit acid-responsive property. Similarly, the particle sizes of Dex-BA/PC and Dex-BA/PIC were also detected by DLS at pH 5.3 and pH 7.4, respectively (Fig. S4 and S5†). Notably, the particle size of Dex-BA/PIC and Dex-BA/PC showed little change with the pH value decreasing from 7.4 to 5.3 because Dex-BA had no acid responsive property at pH 5.3. Though the PIC segment of Dex-BA/PIC could respond to a mildly acidic environment (pH 6.8), the change of pH had nearly no influence on the self-assembly behavior of Dex-BA and PIC at pH 5.3.
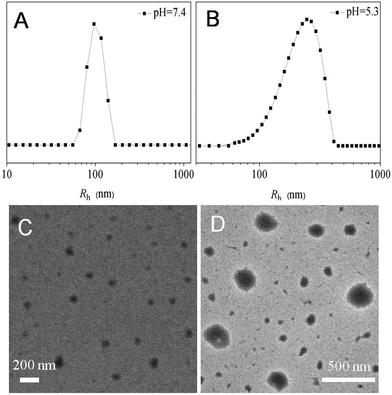 |
| Fig. 4 The hydrodynamic radii (Rh) of Dex-TMBA/PIC at pH 7.4 (A) and pH 5.3 (B) and TEM micrographs of Dex-TMBA/PIC at pH 7.4 (C) and pH 5.3 (D). | |
The stability of the Dex-TMBA/PIC supramolecular nanoparticles in the physiological environment (pH 7.4) was also detected by DLS. As shown in Fig. S6,† the size of the nanoparticles at pH 7.4 in PBS showed no change within 24 h; even after 48 h, the size showed little change, revealing the stability of the supramolecular nanoparticles.
The pH-sensitivity was also confirmed by 1H NMR. As shown in Fig. S7,† the structure of Dex-TMBA is stable both at pH 7.4 (A) and pH 6.8 (B). However, the benzylidene acetal linker was hydrolyzed at pH 5.3 (C). The new peaks appeared at 7.3–8.5 ppm (c) and 10.01 ppm (d) assigned to the benzene ring and the aldehyde group confirmed the hydrolysis of benzylidene acetal. The 1H NMR spectra of PIC in D2O at different pH values as shown in Fig. S8† further indicated that the acid-labile benzoic–imine bond would cleave.
3.6
In vitro drug loading and release
To verify the practicality of this kind supramolecular nanoparticles for intracellular drug delivery in cancer therapy, the encapsulation and release of DOX from the supramolecular nanoparticles were investigated. For comparison, anticancer drug DOX was also loaded in the pH-insensitive Dex-BA/PC and single pH-sensitive Dex-BA/PIC. The in vitro release of DOX was progressed at pH 5.3, 6.8 and 7.4 at 37 °C, mimicking the pH in the late endosome, the tumour extracellular microenvironment and the blood and normal tissue, respectively. The cumulative release percentages of DOX-loaded Dex-TMBA/PIC and DOX-loaded Dex-BA/PC are shown in Fig. 5 (the cumulative release percentages of Dex-BA/PIC is shown in Fig. S9†). As shown by the cumulative release behavior, at pH 5.3, nearly 85% DOX released from DOX-loaded Dex-TMBA/ PIC, which was higher than that from DOX-loaded Dex-BA/PC (47%) and DOX-loaded Dex-BA/PIC (49%) (Fig. S9†) because the cyclic benzylidene acetal bond of TMBA showed pH-sensitivity and would cleave at pH 5.3, resulting in the fast release of loaded DOX.
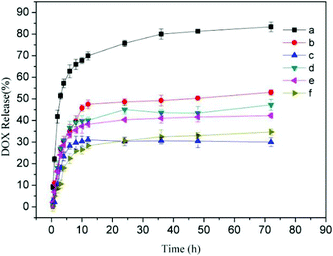 |
| Fig. 5
In vitro DOX release profiles for DOX-loaded Dex-TMBA/PIC in PBS at 37 °C and pH 5.3 (a), 6.8 (b), and 7.4 (c), respectively, as well as DOX-loaded Dex-BA/PC in PBS at 37 °C and pH 5.3 (d), 6.8 (e), and 7.4 (f). | |
3.7 Intracellular DOX release and cellular proliferation inhibition
To demonstrate the usability in intracellular drug delivery application, the potential toxicity of supramolecular nanoparticles was assayed (Fig. S10†). The viability of HeLa and HepG-2 cells treated with blank Dex-TMBA/PIC, Dex-BA/PIC and Dex-BA/PC for 72 h was over 90% at all test concentrations, these results suggested that these supramolecular nanoparticles have great biocompatibility and can be potentially used as drug delivery systems.
The in vitro cytotoxicity of DOX-loaded supramolecular nanoparticles toward HeLa and HepG-2 cells under neutral conditions (Fig. 6 and S11†) and at pH 6.8 culture medium (Fig. S12 and S13†) were evaluated by an MTT assay using free DOX as a control. Both the viabilities of HeLa and HepG-2 cells treated with free DOX and DOX-loaded nanoparticles exhibited a gradual decrease after incubation from 24 h to 72 h. Among them, DOX-loaded Dex-TMBA/PIC showed notably the highest cytotoxicity with the lowest cell viability than that of other DOX-loaded nanocarriers, which was only lower than free DOX. The highest cytotoxicity of DOX-loaded Dex-TMBA/PIC among all the DOX-loaded nanocarriers resulted from the cleavage of pH-sensitive cyclic benzylidene acetal bond of TMBA, which realizes the DOX release quickly. And more importantly, Dex-BA/PIC showed faster DOX release than Dex-BA/PC because the benzoic–imine linkages of Dex-BA/PIC could be triggered by the endosomal acidic environment, presenting the positively charged amino, then promoting Dex-BA/PIC nanocarrier entry into tumor cells and leading to higher inhibition of cell proliferation than Dex-BA/PC. When comparing the in vitro cytotoxicity of different pH culture media, the DOX-loaded Dex-TMBA/PIC and DOX-loaded Dex-BA/PIC at pH 6.8 showed higher cytotoxity than that of pH 7.4, but DOX-loaded Dex-BA/PC showed nearly no change. It also demonstrated that the imine linkage could promote the cell internalization. Above all, the dual pH-responsive Dex-TMBA/PIC shower higher anticancer efficacy than both single acid-sensitive Dex-BA/PIC and acid-insensitive Dex-BA/PC.
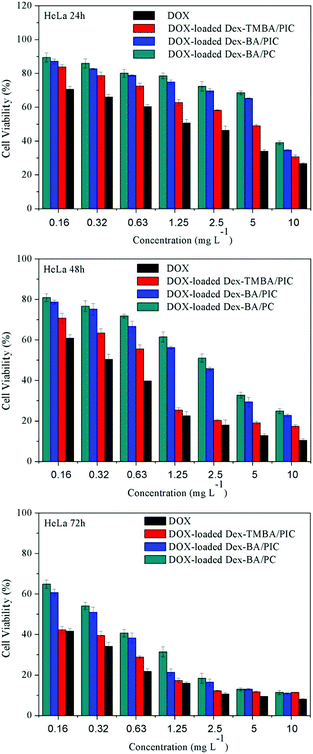 |
| Fig. 6 Cytotoxicity of DOX-loaded supramolecular nanoparticles and free DOX toward HeLa cells after incubation for 24 h, 48 h and 72 h at neutral culture medium. | |
The cellular uptake and intracellular release profiles of DOX-loaded supramolecular nanoparticles toward HeLa cells were also observed by the confocal laser scanning microscopy (CLSM). It is known that most extracellular pH at the solid tumor site is 6.8, therefore, the acid-labile linkage is active and responds to the pH effectively. In our designed drug delivery system, once the DOX-loaded nanocarriers are accumulated in the tumour tissue by the EPR effect, the benzoic–imine linkages of PIC is gradually broken under the first pH sensitivity such as the tumor extracellular condition at pH 6.8.13 Then the surface of DOX-loaded supramolecular nanoparticles will exhibit a positively charged amino. Generally the cell membranes are negatively charged, this positively charged nanoparticles will be easily uptaken by tumoral cells to enhance their internalization. To further confirm if the benzoic–imine linkage would facilitate cell internalization, HeLa cells were incubated with Dex-BA/PIC supramolecular nanoparticles for 3 h at 4 °C.38 The insensitive Dex-BA/PC was prepared as a contrast group. The cells exhibit low activity and no endocytosis for the Dex-BA/PC group. The cellular distribution at neutral culture media evaluated by CLSM analysis is shown in Fig. 7. Compared with the pH-insensitive Dex-BA/PC group (A), a stronger intracellular DOX fluorescence could be observed for the cells incubated with single pH-sensitive Dex-BA/PIC (B) at 4 °C, these results indicating the benzoic–imine bond can cleave at a mildly acidic environment such as tumor extracellular microenvironment, and present a positive charge transmembrane effect and then facilitate the cell internalization. However, the insensitive Dex-BA/PC cannot disconnect without positive charge, so Dex-BA/PC only can enter into cancer cells by endocytosis. As indicated, DOX-loaded Dex-TMBA/PIC was remarkably internalized at 37 °C and distributed intensively in the cytoplasm, which was rarely observed in the cells incubated with the identical Dex-BA/PC at 37 °C. Notably, the strongest intracellular DOX fluorescence detected in the cells treated by DOX-loaded Dex-TMBA/PIC could be attributed to the dual acid-responsive property. Firstly, PIC could respond to the mildly acidic environment, presenting positively charged amino and then promoting transmembrane effects and activating cellular specific uptake, showing that Dex-TMBA/PIC could be efficiently internalized by tumor cells. Secondly, at pH 5.3 the dis-conjugation of Dex-TMBA could trigger the release of the loaded drug. These results revealed that the dual pH-sensitive supramolecular nanoparticles could enhance the target effect on tumor sites and increase the therapeutic efficiency.
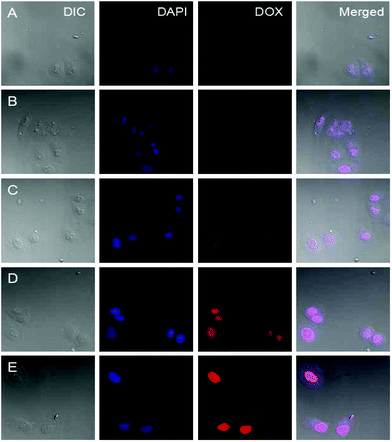 |
| Fig. 7 Representative CLSM images of HeLa cells incubated at neutral culture medium with DOX-loaded Dex-BA/PC at 4 °C (A), DOX-loaded Dex-BA/PIC at 4 °C (B), DOX-loaded Dex-BA/PC at 37 °C (C), DOX-loaded Dex-BA/PIC at 37 °C (D), and DOX-loaded Dex-TMBA/PIC at 37 °C (E). For each panel, the images from left to right show differential interference contrast (DIC) images, cell nuclei stained by DAPI (blue), DOX fluorescence in cells (red), and overlays of the three images. | |
And to further demonstrate whether DOX-loaded Dex-TMBA/PIC and DOX-loaded Dex-BA/PIC nanocarriers can be more efficiently internalized by tumor cells at pHe (∼6.8), we discussed the cellular uptake behaviors at pH 6.8.39 As demonstrated in Fig. S14,† DOX-loaded Dex-TMBA/PIC and DOX-loaded Dex-BA/PIC were more remarkably internalized at pH 6.8 than that at pH 7.4, but DOX-loaded Dex-BA/PC with a non-cleavable amide bond rarely showed DOX fluorescence, which further led to the conclusion that the imine bond definitely enhanced the cellular internalization at pH 6.8. Then the benzylidene acetal bond broke in the intracellular environment, releasing DOX and realizing the dual pH-sensitive feature.
The intracellular triggered drug release was further monitored by flow cytometry analysis toward HeLa cells. As shown in Fig. 8, compared with the cells incubated with other control groups, the flow cytometry histogram for the cells incubated with DOX-loaded Dex-TMBA/PIC at 37 °C obviously shifted to the highest fluorescence intensity position, which should be attributed to the sensitivity to the acidic intracellular microenvironment (pH ∼ 5.3). As expected, the flow cytometry histogram of the cells incubated with DOX-loaded Dex-BA/PIC at 4 °C (b ∼ 397) transferred to the higher fluorescence intensity position than that of DOX-loaded Dex-BA/PC at 4 °C (a ∼ 144) because the pH-sensitive property of the benzoic–imine bond would cleave under mildly acidic tumor extracellular conditions (pH ∼ 6.8) and produce positively charged amino to facilitate cell uptake. The flow cytometry analyses at pH 6.8 culture medium (Fig. S15†) further confirmed the enhanced internalization of DOX-loaded Dex-TMBA/PIC and DOX-loaded Dex-BA/PIC at pH 6.8, which were evidenced by the remarkably enhanced cellular DOX fluorescence. The fluorescence value of DOX-loaded Dex-TMBA/PIC increased from 2916 to 3989 when the pH changed from 7.4 to 6.8, and the value of DOX-loaded Dex-BA/PIC increased from 1658 to 2270, while there was nearly no change observed for insensitive DOX-loaded Dex-BA/PC. All these positive data confirmed that these dual pH-sensitive supramolecular nanoparticles could safely be employed as a drug delivery system with enhanced anticancer efficacy.
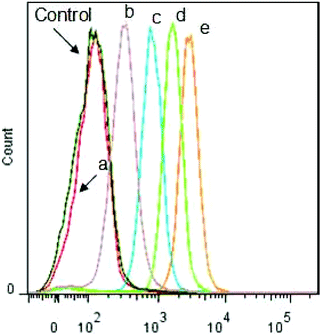 |
| Fig. 8 Flow cytometry profiles of HeLa cells incubated with PBS at pH 7.4 (Control), DOX-loaded Dex-BA/PC at 4 °C (a), DOX-loaded Dex-BA/PIC at 4 °C (b), DOX-loaded Dex-BA/PC at 37 °C (c), DOX-loaded Dex-BA/PIC at 37 °C (d), and DOX-loaded Dex-TMBA/PIC at 37 °C (e). | |
4 Conclusions
In this study, a kind of dual pH-responsive supramolecular nanoparticles had been fabricated by the host–guest recognition between the cyclodextrin (CD) of PIC and the benzene ring of Dex-TMBA, which could respond to both extracellular and intracellular pH environments to simultaneously enhance cellular uptake and promote acid-triggered intracellular drug release. The in vitro experiments indicated that Dex-TMBA/PIC had good biocompatibility and no toxicity towards normal cells. Compared with the control groups, DOX-loaded Dex-TMBA/PIC showed higher in vitro anticancer therapeutic activity, potential for treatment of cancer.
Acknowledgements
This research was financially supported by the National Natural Science Foundation of China (Projects 21474012, 51273037), Jilin Science and Technology Bureau (20130206074GX, International Cooperation Project 20120729), New Century Excellent Talents in University of Jilin Province (2013-6, Jilin Provincial Education Department), Jilin Human Resources and Social Security Bureau (201125020), Jilin Environmental Protection Bureau (201127).
Notes and references
- P. T. Wong and S. K. Choi, Mechanisms of Drug Release in Nanotherapeutic Delivery Systems, Chem. Rev., 2015, 115(9), 3388–3432 CrossRef CAS PubMed.
- X. Zhang, S. Malhotra, M. Molina and R. Haag, Micro- and nanogels with labile crosslinks - from synthesis to biomedical applications, Chem. Soc. Rev., 2015, 44(7), 1948–1973 RSC.
- X. Ma and Y. Zhao, Biomedical Applications of Supramolecular Systems Based on Host–Guest Interactions, Chem. Rev., 2015, 115(15), 7794–7839 CrossRef CAS PubMed.
- Y. Jia and J. Li, Molecular Assembly of Schiff Base Interactions: Construction and Application, Chem. Rev., 2015, 115(3), 1597–1621 CrossRef CAS PubMed.
- E. R. Gillies, A. P. Goodwin and J. M. J. Fréchet, Acetals as pH-Sensitive Linkages for Drug Delivery, Bioconjugate Chem., 2004, 15(6), 1254–1263 CrossRef CAS PubMed.
- W. Hu, L. Cheng, L. Cheng, M. Zheng, Q. Lei, Z. Hu, M. Xu, L. Qiu and D. Chen, Redox and pH-responsive poly (amidoamine) dendrimer–poly (ethylene glycol) conjugates with disulfide linkages for efficient intracellular drug release, Colloids Surf., B, 2014, 123(0), 254–263 CrossRef CAS PubMed.
- T. Sun, Y. S. Zhang, B. Pang, D. C. Hyun, M. Yang and Y. Xia, Engineered Nanoparticles for Drug Delivery in Cancer Therapy, Angew. Chem., Int. Ed., 2014, 53(46), 12320–12364 CAS.
- Y. Wang, H. Wang, Y. Chen, X. Liu, Q. Jin and J. Ji, pH and hydrogen peroxide dual responsive supramolecular prodrug system for controlled release of bioactive molecules, Colloids Surf., B, 2014, 121(0), 189–195 CrossRef CAS PubMed.
- M. Hrubý, S. K. Filippov and P. Štěpánek, Smart polymers in drug delivery systems on crossroads: Which way deserves following?, Eur. Polym. J., 2015, 65(0), 82–97 CrossRef PubMed.
- J.-Z. Du, X.-J. Du, C.-Q. Mao and J. Wang, Tailor-Made Dual pH-Sensitive Polymer–Doxorubicin Nanoparticles for Efficient Anticancer Drug Delivery, J. Am. Chem. Soc., 2011, 133(44), 17560–17563 CrossRef CAS PubMed.
- S.-S. Han, Z.-Y. Li, J.-Y. Zhu, K. Han, Z.-Y. Zeng, W. Hong, W.-X. Li, H.-Z. Jia, Y. Liu, R.-X. Zhuo and X.-Z. Zhang, Dual-pH Sensitive Charge-Reversal Polypeptide Micelles for Tumor-Triggered Targeting Uptake and Nuclear Drug Delivery, Small, 2015, 11(21), 2543–2554 CrossRef CAS PubMed.
- J. Sankaranarayanan, E. A. Mahmoud, G. Kim, J. M. Morachis and A. Almutairi, Multiresponse Strategies To Modulate Burst Degradation and Release from Nanoparticles, ACS Nano, 2010, 4(10), 5930–5936 CrossRef CAS PubMed.
- W. Wu, M. Chen, J. Wang, Q. Zhang, S. Li, Z. Lin and J. Li, Nanocarriers with dual pH-sensitivity for enhanced tumor cell uptake and rapid intracellular drug release, RSC Adv., 2014, 4(58), 30780–30783 RSC.
- B. Kim, E. Lee, Y. Kim, S. Park, G. Khang and D. Lee, Dual Acid-Responsive Micelle-Forming Anticancer Polymers as New Anticancer Therapeutics, Adv. Funct. Mater., 2013, 23(40), 5091–5097 CrossRef CAS PubMed.
- K. A. V. Zubris, Y. L. Colson and M. W. Grinstaff, Hydrogels as Intracellular Depots for Drug Delivery, Mol. Pharm., 2012, 9(1), 196–200 CrossRef CAS PubMed.
- S. Binauld and M. H. Stenzel, Acid-degradable polymers for drug delivery: a decade of innovation, Chem. Commun., 2013, 49(21), 2082–2102 RSC.
- G. H. Gao, Y. Li and D. S. Lee, Environmental pH-sensitive polymeric micelles for cancer diagnosis and targeted therapy, J. Controlled Release, 2013, 169(3), 180–184 CrossRef CAS PubMed.
- T. Thambi, V. G. Deepagan, C. K. Yoo and J. H. Park, Synthesis and physicochemical characterization of amphiphilic block copolymers bearing acid-sensitive orthoester linkage as the drug carrier, Polymer, 2011, 52(21), 4753–4759 CrossRef CAS PubMed.
- Y. Wang, Q. Luo, R. Sun, G. Zha, X. Li, Z. Shen and W. Zhu, Acid-triggered drug release from micelles based on amphiphilicoligo(ethylene glycol)-doxorubicin alternative copolymers, J. Mater. Chem. B, 2014, 2(43), 7612–7619 RSC.
- M. J. S. Phipps, T. Fox, C. S. Tautermann and C.-K. Skylaris, Energy decomposition analysis approaches and their evaluation on prototypical protein-drug interaction patterns, Chem. Soc. Rev., 2015, 44, 3177–3211 RSC.
- L. Peng, M. You, C. Wu, D. Han, I. Öçsoy, T. Chen, Z. Chen and W. Tan, Reversible Phase Transfer of Nanoparticles Based on Photoswitchable Host–Guest Chemistry, ACS Nano, 2014, 8(3), 2555–2561 CrossRef CAS PubMed.
- W. Yuan, J. Shen and W. Guo, Thermoresponse and light-induced reversible self-assembly/disassembly of supra-amphiphiles from azobenzene- and β-cyclodextrin-containing copolymers, Mater. Lett., 2014, 134(0), 259–262 CrossRef CAS PubMed.
- L. M. C. Ferreira, D. Grasseschi, M. S. F. Santos, P. R. Martins, I. G. R. Gutz, A. M. C. Ferreira, K. Araki, H. E. Toma and L. Angnes, Unveiling the Structure of Polytetraruthenated Nickel Porphyrin by Raman Spectroelectrochemistry, Langmuir, 2015, 31(14), 4351–4360 CrossRef CAS PubMed.
- R. D. Poulsen, J. Overgaard, A. Schulman, C. Østergaard, C. A. Murillo, M. A. Spackman and B. B. Iversen, Effects of Weak Intermolecular Interactions on the Molecular Isomerism of Tricobalt Metal Chains, J. Am. Chem. Soc., 2009, 131(22), 7580–7591 CrossRef CAS PubMed.
- R. Ji, J. Cheng, C.-C. Song, F.-S. Du, D.-H. Liang and Z.-C. Li, Acid-Sensitive Polypseudorotaxanes Based on Ortho Ester-Modified Cyclodextrin and Pluronic F-127, ACS Macro Lett., 2015, 4(1), 65–69 CrossRef CAS.
- Y. Takechi-Haraya, K. Tanaka, K. Tsuji, Y. Asami, H. Izawa, A. Shigenaga, A. Otaka, H. Saito and K. Kawakami, Molecular Complex Composed of β-Cyclodextrin-Grafted Chitosan and pH-Sensitive Amphipathic Peptide for Enhancing Cellular Cholesterol Efflux under Acidic pH, Bioconjugate Chem., 2015, 26(3), 572–581 CrossRef CAS PubMed.
- R. Ji, J. Cheng, T. Yang, C. C. Song, L. Li, F.-S. Du and Z.-C. Li, Shell-Sheddable, pH-Sensitive Supramolecular Nanoparticles Based on Ortho Ester-Modified Cyclodextrin and Adamantyl PEG, Biomacromolecules, 2014, 15(10), 3531–3539 CrossRef CAS PubMed.
- Q.-D. Hu, G.-P. Tang and P. K. Chu, Cyclodextrin-Based Host–Guest Supramolecular Nanoparticles for Delivery: From Design to Applications, Acc. Chem. Res., 2014, 47(7), 2017–2025 CrossRef CAS PubMed.
- K. Srinivasan, K. Sivakumar and T. Stalin, 2,6-Dinitroaniline and β-cyclodextrin inclusion complex properties studied by different analytical methods, Carbohydr. Polym., 2014, 113(0), 577–587 CrossRef CAS PubMed.
- L. Yang, X. Tan, Z. Wang and X. Zhang, Supramolecular Polymers: Historical Development, Preparation, Characterization, and Functions, Chem. Rev., 2015, 115(15), 7196–7239 CrossRef CAS PubMed.
- X. Liao, G. Chen, X. Liu, W. Chen, F. Chen and M. Jiang, Photoresponsive Pseudopolyrotaxane Hydrogels Based on Competition of Host–Guest Interactions, Angew. Chem., Int. Ed., 2010, 49(26), 4409–4413 CrossRef CAS PubMed.
- D. Wang, G. Tong, R. Dong, Y. Zhou, J. Shen and X. Zhu, Self-assembly of supramolecularly
engineered polymers and their biomedical applications, Chem. Commun., 2014, 50(81), 11994–12017 RSC.
- G. Yu, K. Jie and F. Huang, Supramolecular Amphiphiles Based on Host–Guest Molecular Recognition Motifs, Chem. Rev., 2015, 115(15), 7240–7303 CrossRef CAS PubMed.
- Z. Lin, J. Li, H. He, H. Kuang, X. Chen, Z. Xie, X. Jing and Y. Huang, Acetalated-dextran as valves of mesoporous silica particles for pH responsive intracellular drug delivery, RSC Adv., 2015, 5(13), 9546–9555 RSC.
- Y. Wu, W. Chen, F. Meng, Z. Wang, R. Cheng, C. Deng, H. Liu and Z. Zhong, Core-crosslinked pH-sensitive degradable micelles: A promising approach to resolve the extracellular stability versus intracellular drug release dilemma, J. Controlled Release, 2012, 164(3), 338–345 CrossRef CAS PubMed.
- W. Chen, F. Meng, F. Li, S.-J. Ji and Z. Zhong, pH-Responsive Biodegradable Micelles Based on Acid-Labile Polycarbonate Hydrophobe: Synthesis and Triggered Drug Release, Biomacromolecules, 2009, 10(7), 1727–1735 CrossRef CAS PubMed.
- Z. Zhao, X. Yao, Z. Zhang, L. Chen, C. He and X. Chen, Boronic Acid Shell-Crosslinked Dextran-b-PLA Micelles for Acid-Responsive Drug Delivery, Macromol. Biosci., 2014, 14(11), 1609–1618 CrossRef CAS PubMed.
- X. Yao, X. Chen, C. He, L. Chen and X. Chen, Dual pH-responsive mesoporous silica nanoparticles for efficient combination of chemotherapy and photodynamic therapy, J. Mater. Chem. B, 2015, 3(23), 4707–4714 RSC.
- C.-S. Lee, W. Park, Y. U. Jo and K. Na, A charge-switchable, four-armed polymeric photosensitizer for photodynamic cancer therapy, Chem. Commun., 2014, 50(33), 4354–4357 RSC.
Footnote |
† Electronic supplementary information (ESI) available. See DOI: 10.1039/c5bm00235d |
|
This journal is © The Royal Society of Chemistry 2016 |
Click here to see how this site uses Cookies. View our privacy policy here.