An integrated sensing and wireless communications platform for sensing sodium in sweat†
Received
26th August 2015
, Accepted 18th October 2015
First published on 23rd October 2015
Abstract
The ability to non-invasively monitor sodium levels in sweat is of significant importance. Sodium is one of the preferred markers to diagnose and track the progression of cystic fibrosis, and knowledge of sodium levels could potentially enable personalised hydration strategies to be implemented for athletes or people working under severe environmental conditions. Herein we present a novel approach for the realisation of disposable potentiometric strips that allow for real-time monitoring of sodium in sweat. Our platform consists of a Solid-Contact Ion-Selective Electrode (SC-ISE) for Na+ detection and of a liquid-junction-free Reference Electrode (RE), combined together on a dual screen-printed substrate. Different poly-3,4-ethylenedioxythiophene (PEDOT) based films were tested as solid-contact, showing a significant impact on sensor characteristics such as sensitivity (i.e. differing from sub-Nernstian to Nernstian), dynamic range (i.e. 10−5 to 10−2.5 or 10−5 to 10−1aNa+), and especially within-batch reproducibility. The SC-ISE/RE combination was integrated into a microfluidic chip that was tested and optimised via on-bench trials. The Potentiometric Microfluidic Chip (PotMicroChip) was then connected to a wireless electronic platform to realise a wearable device whose performance was assessed during real-time stationary cycling sessions.
Introduction
Though Solid-Contact Ion-Selective Electrodes (SC-ISEs) have been used for many years as sensors for blood electrolyte analysis, their mode of operation makes them suitable for “single shot” devices that can be discarded after a single measurement is made. In recent years, driven by several improvements in SC-ISEs overall performance, interest has increasingly been focused on their integration within wearable applications. For this purpose, SC-ISEs should guarantee reliable continuous monitoring for extended periods of time. The stability of the sensors, that is the ability to maintain unaltered calibration parameters, is fundamental as it allows for accurate estimations of ion concentrations when analysing the overall cell potential. Stability has therefore understandably been the focus of several research activities over the past 10–15 years.1–4 Many studies have targeted the solid-contact material, since this layer controls the stability of the potentiometric signal and affects the dynamic range of the ISE.1,4–8 Carbon nanostructured materials like three dimensional ordered mesoporous carbon structures (3DOM)9 and colloidal imprinted mesoporous carbon (CIM),10 together with carbon cloth,11 carbon nanotubes12–14 and graphene15 have received special attention. Their hydrophobicity seems to improve the adhesion of the membrane and limits water uptake and aqueous layer formation at the inner SC/electrode interface. Furthermore, Conducting Polymers (CPs) have been suggested to produce effective solid-contacts.4 Particular attention was devoted to poly(3-octylthiophene) (POT)16–18 thanks to its hydrophobicity. However, manual drop-casting and the possibility of re-dissolution during capping membrane deposition19 are significant drawbacks, as these factors can lead to poor reproducibility. Electropolymerisation seems to be an attractive method to generate more reproducible SC layers, as the films can be grown on a variety of substrates under highly controlled conditions. The use of Ionic Liquids (ILs) as electropolymerisation media, rather than aqueous or organic electrolyte solutions, may offer interesting ways to impart particular properties to the CP film in terms of conductivity, electrochemical stability and mechanical features.20 For instance, the hydrophobicity of the CP layer and the possibility of a water layer building-up at this interface might be regulated through the choice of the IL. This approach has been minimally addressed in the literature, perhaps because the polymer growth is hampered by certain ILs. For instance, Danielsson et al.21 observed that electrodeposition of poly-3,4-ethylenedioxythiophene (PEDOT) films was largely hindered in butyl-3-methyl-imidazolium [bmim] diethylene glycol monomethyl ethersulfate [MDEGSO4] but occurred in [bmim] octyl sulfate [OctSO4]. This is indeed the layer that should affect most of the batch reproducibility while the membrane drop-cast would have almost no significant influence on the overall performance of the sensor.
If optimisation studies are of fundamental importance when realising working electrodes, reliable solid-contact miniaturised reference electrodes are the other component of the galvanic cell that needs to be optimised (e.g. when aiming at the implementation of wearable applications). An amperometric biosensor based on screen-printed electrodes was recently developed to monitor uric acid levels in the exudate of chronic wounds via a wearable, wireless device.22 There have also been some reports on the combination of SC-ISEs with solid-contact reference electrodes to realise low-cost potentiometric combination sensor ‘strips’ for monitoring K+ in saliva13,23 and pH levels in saliva24 and chronic wounds exudate.25 The implementation of screen-printed potentiometric sensors to monitor Cl−,26 the fabrication of sensorised fabrics (e.g. to estimate pH, K+, and NH4+)27 or sensorised tattoo sensor structures transferred on skin (e.g. to detect ammonium, pH, lactate, etc.)28 represent important achievements in the integration of chemical sensors into wearable designs (e.g. allowing for non-invasive monitoring). The only example devoted to detect Na+ variations in real-time was based on a tattoo potentiometric configuration connected to a wireless electronic device.29 The well known coated-wire configuration used to produce solid-state ion-selective electrodes unfortunately suffers from significant drift in the potential signal. Consequently, the approach here presented for real-time detection of Na+ in sweat is different. For the first time, optimisation studies on solid-contact materials for Na+ ISEs showed that conducting polymers based on PEDOT affect the response characteristics, i.e., sensitivity, dynamic range, and within-batch reproducibility of sensors. PEDOT grown in 1-ethyl-3-methylimidazolium bis-(trifluoromethanesulfonyl)imide [emim][NTf2] seemed to offer the best compromise and was employed while realising batches of solid-contact screen-printed sodium ISEs. All plastic potentiometric strips were then made with a dual electrode configuration based on solid-contact working and reference electrodes. Furthermore, they were integrated into a microfluidic chip, able to drive the fluid and allow the interaction between the sample and the sensitive area, thanks to a special configuration that behaves as a passive pump. After on bench trials, this Potentiometric Microfluidic Chip (PotMicroChip) was connected to a Macroduct® (slightly modified to fulfil the constraints of the platform here presented) which is a commercial product used to harvest sweat samples. The connection to the wireless electronic platform (Mote) finally enabled the implementation of a novel wearable device able to monitor in real-time sodium variations of volunteers undergoing stationary cycling sessions.
Experimental
Materials
C2030519P4 and D50706D2 from Gwent Inc. (Pontypool, UK) were used respectively as carbon and dielectric ink in the preparation of the screen-printed electrodes. 175 μm thick PET sheets by HiFi (Dublin, Ireland) were used without further treatment. 80 μm pressure-sensitive adhesive (PSA-AR9808) by Adhesives Research (Ireland), 500 and 1500 μm poly(methylmethacrilate) (PMMA) from GoodFellow (UK) were used for the realisation of the microfluidic chip layers. A nozzle (BDML210-9 from Value-Plastic, USA) was used as connector (i.e. preliminary design) between the microfluidic chip and the peristaltic pump or modified Macroduct® (Accuscience, Dublin). Highly absorbent material (Sponge Cloths from Dunnes Stores, Dublin, Ireland), cotton (Verbandwatten from Van Heek Medical, Lasser, Holland) and cotton threads removed from medical bandages (W.O.W. Bandage BP, 5 cm × 5 cm) were used to realise the passive pump system inserted in the microfluidic chip. Potassium chloride, sodium chloride, calcium chloride, lactic acid, hydrochloric acid, potassium ferricyanide(III) (99%), iron(III) chloride hexahydrate, 3,4-ethylenedioxythiophene (EDOT, 97%), high molecular weight poly(vinyl chloride) (PVC), tetrahydrofuran (THF, ≥99.5%), 4-tert-butylcalix[4]arene-tetraacetic acid tetraethyl ester (sodium ionophore X), potassium tetrakis(4-chlorophenyl)borate (KTFPB), bis(2-ethylhexyl)sebacate, 2,2-dimethoxy-2-phenylacetophenone (DMPA, >99%), butyl-acrylate (>99%) and 1,6-hexanediol diacrylate (HDDA, 80%) were purchased from Sigma-Aldrich (Dublin, Ireland). When possible, they were of selectophore grade. N-Decyl-methacrylate was obtained from Polysciences (Northampton, UK). 1-Ethyl-3-methylimidazolium bis(trifluoromethanesulfonyl)imide [emim][NTf2], [emim] tris(pentafluoroethyl)trifluorophosphate [FAP] and 1-hexyl-3-methylimidazolium [hmim] [FAP] were obtained from VWR (Dublin, Ireland). All the chemicals were used without any further purification. Adhesive poly-foam strips (employed for the preparation of gaskets) were obtained from Radionics (Ireland). FFC-FPC Molex connectors (gold contacts, 14 ways from Farnell, Ireland) were used to plug the potentiometric strip. Deionised water with resistivity of 18.2 MΩ cm obtained with a Milli-Q reagent-grade water system was used for making all aqueous solutions.
Na+ ISEs
All screen-printed electrodes (SPEs) were realised using a DEK 248 printer, as previously reported,24 in a combined configuration on PET substrates (see Fig. 1(a)). To prepare the conducting polymer SC film, EDOT solutions were first vortexed for ∼1 hour to facilitate solubilisation. PEDOT was deposited electrochemically directly on the carbon electrodes from a 0.05 M EDOT solution in pure [emim][FAP], or [emim][NTf2], or in aqueous 0.1 M KCl. Deposition was accomplished using cyclic voltammetry by scanning the potential 25 times from 0 to 1.0 V vs. Ag wire with a scan rate of 50 mV s−1. Alternatively, potentiostatic depositions were achieved applying a constant potential of 1.0 V vs. Ag wire (EDOT in IL solutions) or vs. Ag/AgCl reference electrode (EDOT in KCl solution) for 900 seconds. A PEDOT/Prussian Blue (PB) composite was also tested as solid-contact material. The composite deposition was carried out following the procedure previously reported by Lupu et al.23 A total of 26.25 μL Na+ selective membrane material dissolved in THF30 was drop-cast on top of the solid-contact PEDOT layer in the following sequence: 1 μL (once); 1.5 μL (3 times); 2.5 μL (3 times); 3 μL (twice); 3.25 μL (once) and 4 μL (once). When realising the PotMicroChip, because of the larger diameter of the gasket (3.6 mm), a total of 65 μL of Na+ selective membrane were drop-cast in the following sequence: 4 μL (11 times); 3 μL (5 times) and 2 μL (3 times). After each addition, the Na+ selective membrane solution was allowed to dry for 20 minutes before the next addition. All the resulting Na+ SC-ISEs were conditioned overnight in a 10 mM NaCl solution prior their calibration with NaCl solutions of increasing concentrations. The SCI-REs were prepared as elsewhere reported.19
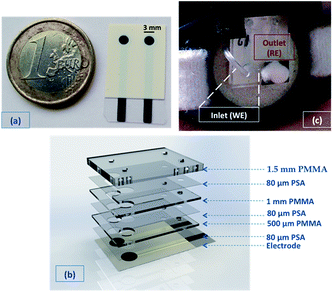 |
| Fig. 1 (a) Dual screen-printed electrodes on a PET substrate and (b) different layers employed to build up the microfluidics collecting system. (c) Enlargement of the PotMicroChip showing the inlet (on top of the working electrode) and the outlet (on top of the reference electrode), characterised by a reservoir containing a highly absorbent material. | |
Microfluidic chip realisation
The microfluidic chip was made up of 7 layers, as shown in Fig. 1(b), with the PSA and PMMA layers cut via a CO2 laser ablation system (see also Fig. SID1(a)†). The first PET layer contained the screen-printed electrodes, where 80 μm thick PSA (2nd layer), followed by 500 μm thick PMMA (3rd layer) were rolled on top. The RE membrane was photopolymerised following the procedure described by Zuliani et al.,19 followed by the drop-casting of the Na+ membrane on the other electrode (as described in Section Na+ ISEs). 80 μm thick PSA was then put on top (4th layer), creating a μ-channel between the two electrodes (see Fig. 1(b)), followed by a 1.5 mm thick layer of PMMA (5th layer) rolled on top. The highly absorbent material (that underwent through a washing step with deionised water to remove any surfactant or ionic impurities, and was then dried at 65 °C) was punched with the specular configuration of the 5th layer, to cover the electrodes and fill the channel. A PSA layer was transferred on top (6th layer, see Fig. 1(b)) and an additional PMMA layer of 1.5 mm (7th layer) was rolled on top to enclose the system.
The 7th layer was characterised by 2 holes through which a cotton thread was inserted (after being washed and dried like the highly absorbent material) to assist fluid movement into the microfluidic chip. The hole located in correspondence of the working electrode was the inlet of the system, connected to the Macroduct® (see Fig. 1(c)). The other was the outlet, and was covered by a punched poly-foam strip that worked as a reservoir filled with highly absorbent material (cotton bud, see Fig. 1(c)). This section was the ending part of the passive pump, which soaked up the sample delivered by the microfluidic chip. After removal, it can be employed to quantify and compare the Na+ levels obtained via standard analytical techniques with the ones measured using PotMicroChips.
Real-time cycling sessions
Healthy active male athletes participated in indoor exercise trials using stationary cycles (average room temperature of 22 °C) and a cycle ergometer (Monark Exercise, Sweden) at an intensity load high enough to induce sweating. A PotMicroChip was positioned on the upper left arm (the sampling area was first cleaned with alcohol swabs and deionised water) by means of Velcro® straps. The external forearm was selected as the primary sampling location since it provided a relatively flat surface to secure the collecting device (see Fig. 2). Trials were stopped when the athlete was no longer capable of maintaining the set intensity load.
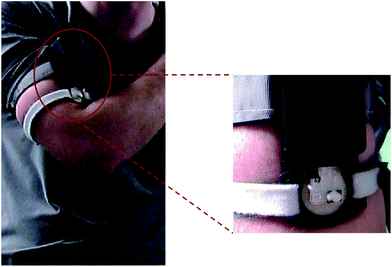 |
| Fig. 2 PotMicroChip connected to the Mote and positioned on the upper arm via Velcro® straps and enlargement of the sensing microfluidic area as worn during stationary cycling sessions. See also Fig. SID2† for more images of the system as worn, during trials. | |
Instrumentation and software
The potentiometric measurements were recorded using an EMF-16 channel system from Lawson Labs (USA), along with an in-house made silver/silver chloride (saturated KCl) or double-junction Ag/AgCl reference electrode (Sigma-Aldrich, Dublin), for conditioning and testing, respectively. Electropolymerisation was achieved using a CHI760d potentiostat (CH-instruments, USA). Photo-polymerisation of the acrylate monomers (i.e. for the realisation of reference electrodes) was obtained using the CL-1000 ultraviolet cross-linker UVP. The CO2 laser ablation system was an Epilog Zing Laser Engraver (Epilog, USA) whereas the thermal roller laminator (Titan-110) was from GBC Films (USA). A peristaltic pump (Ismatec Reglo ICC from IDEX Wertheim, Germany) was used at a constant flow rate (0.2 mL min−1) to test PotMicroChips on the bench. The wireless electronic platform (Mote) was developed by the Tyndall National Institute, Cork, Ireland, as previously described by O'Flynn et al.31 The Mote stack consisted of 3 layers: a lithium polymer rechargeable 950 mA h battery, a ZigBee transceiver and a customised ISE interfacing layer. The mote measured the differential input analogue voltage (potentiometric bias) with a variable gain through high-input impedance and converted it to a digital signal through the ADC component. The digital signal was then wirelessly transmitted to a base-station (see O'Flynn31 for more details). Low power consumption protocols were used during communication, signal processing, etc. (e.g. the radio powering consumption was lower of a factor of nearly a thousand when in sleep mode than in active mode) (see O'Flynn et al.31 for more details). The remote sensing range went from 10 to 100 m in open field best conditions. A PotMicroChip was connected to the Mote (and encapsulated in a 3D printed encasing) via a simple push-in socket (Molex FFC-FPC) and was calibrated in 2 sessions (using 10−5 M, 10−3 M and 10−1 M NaCl solutions) both before and after real-time measurements, to take into consideration possible potential offsets that might affect the signal reading after exposure to sweat samples. Following real-time measurements, the sweat Na+ levels were interpolated from calibration curves recorded after real-time cycling sessions.
Laboratory trials
All experiments involving human subjects were performed in compliance with the Irish Law and Institutional Guidelines, see https://www4.dcu.ie/researchsupport/research_ethics/guidelines.shtml, and were approved by DCU Research Ethics Committee. Informed consent was obtained for all experiments involving human subjects.
Results and discussion
Solid-contact Na+ ISEs
Table 1 summarises the impact of the material chosen for the solid contact of analytical characteristics such as sensitivity, dynamic range and sensor batch reproducibility. The overall response of sensors having a PEDOT solid-contact layer (electrodeposited from aqueous KCl) was linear (R2 > 0.98) within the Na+ activity range 10−5 to 10−1, with an average slope and offset of 55.5 mV/log
aNa+ and 474.8 mV (n = 3), respectively. The standard deviations of slope and offset extrapolated from the averaged calibration curve were quite large, i.e., 4.9 mV/log
aNa+ and 23.1 mV, respectively.
Table 1 Dynamic range, slope and offset listed to describe the performance of Na+ ISEs realised using different materials as solid-contact: PEDOT grown in aqueous KCl (PEDOTKCl); the organic/inorganic composite of PEDOT and Prussian Blue (PEDOTPB); PEDOT grown in an ionic liquid media such as [emim][FAP] or [emim][NTf2]
Solid contact |
Dynamic range (M) |
Slope (mV/log aNa+) |
Offset (mV) |
PEDOT(KCl) (n = 3) |
10−5 to 10−1 |
55.5 ± 4.9 |
474.8 ± 23.1 |
PEDOT(PB) (n = 4) |
10−5 to 10−1 |
53.4 ± 3.0 |
524.1 ± 14.4 |
PEDOT[emim][FAP] (n = 3) |
10−5 to 10−2.5 |
40.6 ± 2.4 |
235.2 ± 12.4 |
PEDOT[emim][NTf2] (n = 4) |
10−5 to 10−1 |
53.1 ± 0.8 |
525.5 ± 3.6 |
Improving the within-batch reproducibility of the Na+ SC-ISE is a key step to obtain large batches of sensors that can be employed without individual calibration. For instance, highly reproducible sensors would be ideal for use in the field of sport sciences (e.g. for body fluid analysis) because of the limited time available to screen exercising athletes, and the ease of use of calibration free devices. It seems, therefore, that PEDOT electrodeposited from KCl aqueous solutions (see Table 1) was not suitable to prepare sensors with these ideal characteristics.
PEDOT/PB23 is an organic/inorganic composite material with potentially superior performance due to the well-defined potential of the PB redox couple. Sensors provided with the PEDOT/PB film as SC-layer showed good linear calibration (R2 > 0.98) within the 10−5 to 10−1 Na+ activity range. By averaging the calibration curves, the slope and offset values were found to be equal to 53.4 ± 3.0 mV/log
aNa+ and 524.1 ± 14.4 mV (n = 4), respectively (see Table 1). Although the standard deviations were smaller than the ones obtained with a PEDOT SC-layer grown from aqueous KCl, they were yet too large to be considered for use in a calibration free mode. These differences dictate the requirement for at least one point calibration before use and the need for further screening of other types of materials to be used to produce solid-contacts with better reproducibility.
ILs employed as media for the SC-layer electropolymerisation might provide new means to tailor the physico-chemical properties of this layer.20 For instance, ILs have a low-water content and therefore provide a water-free environment during the CP electrodeposition. In addition, since water can percolate through the membrane during the sensor operation time, the incorporation of the IL within the SC-layer may hinder the formation of a water-layer at the SC/membrane interface in relation to the IL hydrophobicity. By electrodepositing PEDOT from ILs, we speculated that the water uptake within the sensors would be reduced and that perhaps this factor may help improving the sensor batch reproducibility. [emim][FAP] and [emim][NTf2] were selected for this purpose since these ILs have very different physico-chemical properties (e.g. viscosity and conductivity). PEDOT films electrodeposited using these two ILs showed very different morphologies (as previously discussed by Zuliani et al.19).
Na+ SC-ISEs prepared with a PEDOT SC-layer potentiodynamically deposited from [emim][FAP] were characterised by a markedly sub-Nernstian average calibration slope (40.6 ± 2.4 mV/log
aNa+, n = 3) and a linear dynamic range between 10−5 and 10−2.5. Moreover, Fig. 3 presents the calibration curve obtained with sensors having a PEDOT SC-layer potentiostatically electrodeposited from [emim][NTf2]. In this case, the trends were linear (R2 > 0.99) within the Na+ activity range 10−5 to 10−1 and the average values of the slope and offset are 53.1 ± 0.8 mV/log
aNa+ and 525.5 ± 3.6 mV, respectively (n = 4) (see Table 1).
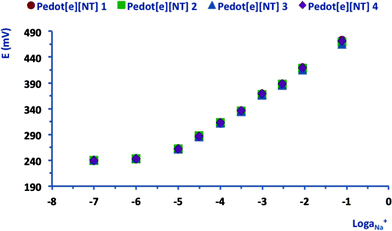 |
| Fig. 3 Calibration curves of Na+ ISEs with a solid-contact made of PEDOT potentiostatically grown in [emim][NTf2] ( , , , ), n = 4. The electrodes were conditioned overnight in 10 mM NaCl before the calibration was performed. For convenience, the plots were normalised to responses at a Na+ activity of 10−5. | |
Furthermore, a significant decrease in the standard deviation values of the slope and offset was recorded. The improved batch reproducibility likely arose from the type of solid-contact layer employed since the Na+ ion-selective membrane was the same in all cases. Additionally, the ISEs exhibited a low drift of −0.04 ± 0.01 mV min−1, measured over 4 hours. Thus, [emim][NTf2] seemed to confer better properties to the solid-contact when compared to KCl or potassium ferricyanide (used for the PEDOT/PB composite preparation). Most likely, this result was achieved not only by avoiding the presence of water during the CP polymerisation process, but also, perhaps, by hampering or slowing down the CP de-doping process. Furthermore, the insertion/expulsion of ions during the redox changes of the CP depends on the bulkiness and physico-chemical nature of these ions.20,32 It is noteworthy that Bobacka et al.33 observed that anions entrapped in the PEDOT backbone affected the drift of the potential in K+ SC-ISEs. In addition, Michalska et al.34,35 reported that spontaneous charging/discharging may occur at the CP layer underlying that a PVC based ion-selective membrane in turn affects the response pattern of the sensor. Na+ ISEs characterised by a solid-contact layer made of PEDOT grown in [emim][NTf2] were also tested at different concentrations of NaCl in solutions mimicking sweat composition (5 mM CaCl2, 3 mM KCl, 14 mM lactic acid). Small variations in sensitivity were observed, with an average decrease of −4.1 ± 2.8 mV/log
aNa+ (n = 7). The optimised Na+ ISEs were then combined with a Solid-Contact Ionogel Reference Electrode (SCI-RE) to realise a disposable potentiometric strip. In this strip, the SC layers were deposited at once on both electrodes and the SC-ISE and SCI-RE differed only in the capping membrane.
Realisation and testing of PotMicroChips
Following the optimisation of the potentiometric platform, we worked to build a system able to deliver the sample to the electrodes. The microfluidic device adopted during the first trials is shown in Fig. SI1(a) of the ESI.† The inlet of the platform was connected via a nozzle to a benchtop peristaltic pump, and the electrodes were inserted in the Molex FFC-FPC, which was electrically connected to the Mote (see Fig. SI1(b) for the experimental set up in the ESI†). The difference of potential was wirelessly measured for a 3 points calibration (i.e. concentrations of 10−5, 10−3 and 10−1 M NaCl) (see Fig. SI1(c)†), at a constant flow rate (0.2 mL min−1). The calibration slope was sub-Nernstian (43.9 mV/log
aNa+) but suitable for real-time monitoring. However, after the device was connected to the modified Macroduct® via the sampling nozzle and positioned on the upper arm of a volunteer (see Fig. SI2(a), ESI†), we observed that sweat was not able to enter the microfluidic chip. The system was not able to sample the sweat, and this failure was likely due to the high surface tension generated at the interface between the Macroduct® tubing and the nozzle, and the smaller driving force of the sweat flow compared to the pump. The recorded signal showed a characteristic flat line with spikes arising from noise artefacts (see Fig. SI2(b), ESI†). These trials underlined the need for a system endowed with a more effective passive pumping mode of operation, which would be better able to overcome the surface tension barriers to sample movement at the various fluidic interfaces. We thus adopted a strategy where a highly absorbent material was put in contact with the electrodes within the channel. Additionally, a cotton thread going from the inlet to the outlet, as already implemented in previous studies,36,37 was used to assist fluid movement through the system due to enhanced capillary forces and enhanced hydrophilicity (see Fig. 4(a)).
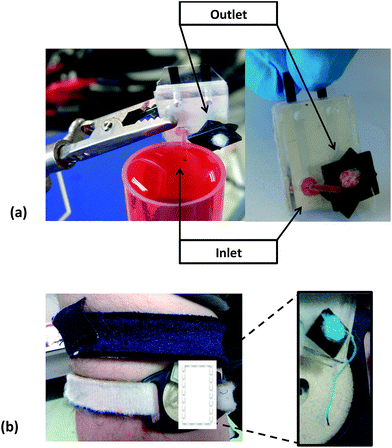 |
| Fig. 4 PotMicroChip featured by a passive pump system integrated within the microfluidic channel and used to provide a driving force for fluid movement based on capillary attraction from the sampling point through the fluidic system to a highly adsorbant material. (a) PotMicroChip tested on the bench (red solution and sampling tube). (b) Real-time testing of a PotMicroChip during stationary cycling sessions (i.e. the blue dye is used to track the progress of sweat through the commercial Macroduct® sweat sampler, see enlargement). | |
A reservoir was finally positioned on top of the outlet, containing a hydrophilic cotton bud, which was the final element of the whole passive pump. The cotton bud provides a high capability for continuous transfer of water through the system, which in turn allows continuous real-time monitoring during cycling sessions. Furthermore, the harvested sweat in the adsorbant material is available for subsequent validation measurements via standard analytical techniques to verify the Na+ levels measured by the potentiometric platform (i.e. the adsorbant material can be removed and the amount of sweat and concentration of sodium measured using reference analytical techniques to give an overall picture of the sweat sodium level).
This configuration was first examined on the bench (see Fig. 4(a)), to assess its efficacy. It was then tested during real-time sessions using exercise bicycles that proved the feasibility of the microfluidic passive pump approach (see Fig. 4(b)), as shown by the blue colour characterising the cotton thread and the cotton bud positioned at the outlet. Fig. 4(b) shows that the fluid is able to travel along the system, from the inlet to the outlet.
It was then possible to test the performance of the fully integrated PotMicroChips platforms (i.e. potentiometric sensor, microfluidics and wireless electronics). The electrodes were conditioned in 10−2 M NaCl and then the microfluidic unit was mounted on top, and the system closed with the last PMMA layer. PotMicroChips were first tested on the bench using a 3 point calibration (Fig. SID1(b) and (c)†) in a concentration range consistent with real sweat samples (i.e. 10–60 mM and 65–90 mM for normal subjects and patients affected by cystic fibrosis, respectively). Fig. 5 shows the average calibration of the PotMicroChips (n = 3), characterised by a slight sub-Nernstian behaviour (50.23 ± 7.7 mV/log
aNa+) in the range 10−3 to 10−1 M.
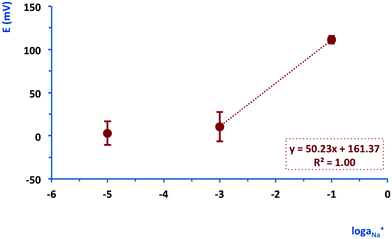 |
| Fig. 5 Example of the average of an on-bench calibration of 3 PotMicroChips. The error bars represent the standard deviation. | |
Before being used to monitor sweat samples, the chips were flushed with deionised water to remove any ionic background. A PotMicroChip was then connected to the modified Macroduct®. The length of the tubing was shortened of 22.7 mm to allow for the connection with the top PMMA layer of the microfluidics and minimise the dead volume of the platform (i.e. decreased from 110.8 μL to 4.6 μL). The wearable device was finally positioned on the upper arm of volunteers. Fig. 6(a) shows real-time potential variations of PotMicroChips during cycling sessions and Fig. 6(b) the corresponding sodium levels (i.e. computed from calibration). The potential variations in Fig. 6(a) were characterised by a baseline value that described the environment in contact with the electrodes before the collected sample entered the μ-channel (as indicated by the arrows). Both potentials then featured a rapid increase that occurred at different times, i.e., after nearly 20 minutes (see Fig. 6(a), left) and 8 minutes (see Fig. 6(a), right), indicating that perspiration started at different stages in the two subjects (as suggested by previous studies29). The signal then reached a steady state within about 2 minutes (see Fig. 6(a), left) and 5 minutes (see Fig. 6(a), right), which more or less endured until the end of the trial.
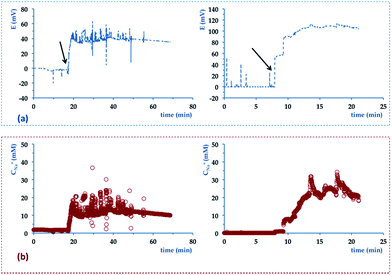 |
| Fig. 6 Example of signal recorded in real-time during a stationary cycling session with the wearable device used to monitor Na+ levels of two different volunteers. (a) shows real-time variations of potential vs. time (e.g. the arrows indicate when sweat enters the PotMicroChip). (b) shows real-time changes of Na+ concentrations vs. time. The device was positioned as shown in Fig. 2. | |
Fig. 6(b) shows real-time variations of Na+ concentrations during exercise sessions. When sweat started to be harvested by the PotMicroChips, Na+ levels increased for 2 minutes (see Fig. 6(b), left) and 5 minutes (Fig. 6(b), right). Thanks to previous work done by Buono et al.,38 we can speculate that enhancements in Na+ levels might be related to contemporary augmented sweat rates, until a steady state was attained by both parameters.
Na+ levels then levelled off and reached average values of 10.3 ± 0.2 mM (see Fig. 6(b), left) and 24.2 ± 2.7 mM (see Fig. 6(b), right) (both of them within the normal physiologic range). Despite similarities on the overall trends, some differences can be noticed, e.g. in terms of duration and of Na+ concentrations observed (see Fig. 6). One of the trials (see Fig. 6(a) left) was characterised by potential readings (and consequently decreased Na+ concentrations) that decreased over time. This might be due to variations in sweat production rates, an observation that will be better clarified in future studies when the whole device will be endowed with an additional system able to simultaneously track this parameter.39
This was expected because diverse athletes (e.g. featured by different genetics, hydration, diet, heat acclimation, etc.) will produce personalised sweat profiles. Additionally, different workloads were chosen during the trials, according to the fitness level of the volunteer involved.
Signals were however characterised by some spikes that represent noise artefacts, most likely due due to sudden movements of the wearer. However, the Mote was endowed with a 3D printed casing able to keep its position reasonably stable and fixed on the body, and minimising movement of the internal circuitry, and during some of the cycling sessions, we performed body movement tests and they did not appear to unduly affect the sensor stability.
From these trials, the average interpolated sodium concentration at the end of cycling sessions was found to be 18.2 ± 8.9 mM, (n = 4) and it was possible to capture unique “over time sodium profiles” for each athlete, as it was possible to track changes in Na+ levels from the initial point of sweat contact with the sensor, through to the completion of the trial.
Due to the short duration of these studies, issues related to biofouling are minimal. The variations in sweat volume production from person to person, and the selection sampling location on the body, are more important and need to be investigated in more detail. On-body continuous evaluation of sodium in sweat has potential applications in the field of sport sciences2 or in clinical practice to diagnose and treat cystic fibrosis (CF) patients40,41 or during acclimation studies.38
In the near future, PotMicroChips will be validated through larger scale trials. The detected Na+ levels will be compared with measurements obtained via standard analytical techniques (e.g. atomic absorption spectroscopy or ion chromatography), or commercial sodium meters (e.g. AquaTwin®)42 to bypass tedious sampling and post-treatment protocols.
Conclusions
A detailed optimisation study was carried out to ascertain which is the best solid-contact material to employ during the realisation of Na+ ISEs. Our attention was mainly focused on PEDOT grown in media with different ionic backgrounds. The optimised solid-contact potentiometric strips were integrated with microfluidic chips (PotMicroChip) endowed with a passive pump system for the effective delivery of sweat samples. The system was connected to a miniaturised wireless communications platform encased in a 3D printed wearable casing. After positioning on the arm of volunteers, variations in Na+ concentrations were monitored in real-time during stationary cycling sessions. We are currently working on reducing the overall size of the PotMicroChips through a re-design of the platform. The next generation platform will be based on a watch-like configuration will allow the realisation of a much more innocuous miniaturised wearable device with broad potential to monitor various sweat components in real-time.
Acknowledgements
The authors wish to acknowledge support from Science Foundation Ireland under the Insight Centre Initiative (12/RC/2289).
References
-
J. Bobacka and A. Ivaska, in Electrochemical Sensor Analysis, ed. S. Alegret and A. Merkoçi, Elsevier, 2007, pp. 72–81 Search PubMed.
- C. Zuliani and D. Diamond, Electrochim. Acta, 2012, 84, 29–34 CrossRef CAS.
- S. Anastasova, A. Radu, G. Matzeu, C. Zuliani, U. Mattinen, J. Bobacka and D. Diamond, Electrochim. Acta, 2012, 73, 93–97 CrossRef CAS.
- E. Lindner and R. E. Gyurcsányi, J. Solid State Electrochem., 2009, 13, 51–68 CrossRef CAS.
- J. Bobacka, A. Ivaska and A. Lewenstam, Chem. Rev., 2008, 108, 329–351 CrossRef CAS PubMed.
-
J. Bobacka and A. Ivaska, in Electropolymerisation. Concepts, Materials and Applications, ed. A. K. Cosnier, Wiley-VCH, Weinheim, 2010, p. 173 Search PubMed.
- T. Lindfors, L. Höfler, G. Jágerszki and R. E. Gyurcsányi, Anal. Chem., 2011, 83, 4902–4908 CrossRef CAS PubMed.
- J.-P. Veder, R. de Marco, G. Clarke, S. P. Jiang, K. Prince, E. Pretsch and E. Bakker, Analyst, 2011, 136, 3252–3258 RSC.
- C.-Z. Lai, M. A. Fierke, A. Stein and P. Bühlmann, Anal. Chem., 2007, 79, 4621–4626 CrossRef CAS PubMed.
- J. Hu, X. U. Zou, A. Stein and P. Bühlmann, Anal. Chem., 2014, 86, 7111–7118 CrossRef CAS PubMed.
- U. Mattinen, S. Rabiej, A. Lewenstam and J. Bobacka, Electrochim. Acta, 2011, 56, 10683–10687 CrossRef CAS.
- T. Kakiuchi, T. Yoshimatsu and N. Nishi, Anal. Chem., 2007, 79, 7187–7191 CrossRef CAS PubMed.
- F. X. Rius-Ruiz, G. A. Crespo, D. Bejarano-Nosas, P. Blondeau, J. Riu and F. X. Rius, Anal. Chem., 2011, 83, 8810–8815 CrossRef CAS PubMed.
- U. Guth, F. Gerlach, M. Decker, W. Oelßner and W. Vonau, J. Solid State Electrochem., 2009, 13, 27–39 CrossRef CAS.
- R. Hernández, J. Riu, J. Bobacka, C. Vallés, P. Jiménez, A. M. Benito, W. K. Maser and F. X. Rius, J. Phys. Chem. C, 2012, 116, 22570–22578 Search PubMed.
- K. Y. Chumbimuni-Torres, N. Rubinova, A. Radu, L. T. Kubota and E. Bakker, Anal. Chem., 2006, 78, 1318–1322 CrossRef CAS PubMed.
-
A. Radu and D. Diamond, in Electrochemical Sensor Analysis, ed. S. Alegret and A. Merkoçi, Elsevier, 2007, vol. 2007, pp. 25–27 Search PubMed.
- J.-P. Veder, R. de Marco, G. Clarke, R. Chester, A. Nelson, K. Prince, E. Pretsch and E. Bakker, Anal. Chem., 2008, 80, 6731–6740 CrossRef CAS PubMed.
- C. Zuliani, G. Matzeu and D. Diamond, Talanta, 2014, 125, 58–64 CrossRef CAS PubMed.
- P. Hapiot and C. Lagrost, Chem. Rev., 2008, 108, 2238–2264 CrossRef CAS PubMed.
- P. Danielsson, J. Bobacka and A. Ivaska, J. Solid State Electrochem., 2004, 8, 809–817 CrossRef CAS.
- P. Kassal, J. Kim, R. Kumar, W. R. de Araujo, I. M. Steinberg, M. D. Steinberg and J. Wang, Electrochem. Commun., 2015, 56, 6–10 CrossRef CAS.
- S. Lupu, B. Lakard, J.-Y. Hihn, J. Dejeu, P. Rougeot and S. Lallemand, Thin Solid Films, 2011, 519, 7754–7762 CrossRef CAS.
- C. Zuliani, G. Matzeu and D. Diamond, Electrochim. Acta, 2014, 132, 292–296 CrossRef CAS.
- T. Guinovart, G. Valdés-Ramírez, J. R. Windmiller, F. J. Andrade and J. Wang, Electroanalysis, 2014, 26, 1345–1353 CrossRef CAS.
- J. Gonzalo-Ruiz, R. Mas, C. de Haro, E. Cabruja, R. Camero, M. A. Alonso-Lomillo and F. J. Muñoz, Biosens. Bioelectron., 2009, 24, 1788–1791 CrossRef CAS PubMed.
- T. Guinovart, M. Parrilla, G. A. Crespo, F. X. Rius and F. J. Andrade, Analyst, 2013, 138, 5208–5215 RSC.
- A. J. Bandodkar, W. Jia and J. Wang, Electroanalysis, 2015, 27, 562–572 CrossRef CAS.
- A. J. Bandodkar, D. Molinnus, O. Mirza, T. Guinovart, J. R. Windmiller, G. Valdés-Ramírez, F. J. Andrade, M. J. Schöning and J. Wang, Biosens. Bioelectron., 2014, 54, 603–609 CrossRef CAS PubMed.
- B. Schazmann, D. Morris, C. Slater, S. Beirne, C. Fay, R. Reuveny, N. Moyna and D. Diamond, Anal. Chem., 2010, 2, 342–348 CAS.
-
B. O'Flynn, S. Bellis, K. Delaney, J. Barton, S. C. O'Mathuna, A. M. Barroso, J. Benson, U. Roedig and C. Sreenan, 2005.
-
G. G. Wallace, G. M. Spinks and L. A. P. Kane-Maguire, in Conductive Electroactive Polymers: Intelligent Polymer Systems, CRC Press, 2009, pp. 59–101 Search PubMed.
- J. Bobacka, Anal. Chem., 1999, 71, 4932–4937 CrossRef CAS PubMed.
- A. Michalska, M. Wojciechowski, E. Bulska and K. Maksymiuk, Talanta, 2010, 82, 151–157 CrossRef CAS PubMed.
- A. Michalska and K. Maksymiuk, J. Electroanal. Chem., 2005, 576, 339–352 CrossRef CAS.
- V. F. Curto, S. Coyle, R. Byrne, N. Angelov, D. Diamond and F. Benito-Lopez, Sens. Actuators, B, 2012, 175, 263–270 CrossRef CAS.
- P. K. Yuen, Lab Chip, 2013, 13, 1737–1742 RSC.
- M. J. Buono, K. D. Ball and F. W. Kolkhorst, J. Appl. Physiol., 2007, 103, 990–994 CrossRef CAS PubMed.
- G. Matzeu, C. Fay, A. Vaillant, S. Coyle and D. Diamond, IEEE Trans. Biomed. Eng., 2015, 1, DOI:10.1109/tbme.2015.2477676.
- A. Lynch, D. Diamond and M. Leader, Analyst, 2000, 125, 2264–2267 RSC.
- B. J. Rosenstein and G. R. Cutting, J. Pediatr., 1998, 132, 589–595 CrossRef CAS PubMed.
-
http://www.horiba.com/uploads/media/concept_catalog_en_04.pdf, (accessed 28 April 2015).
Footnotes |
† Electronic supplementary information (ESI) available. See DOI: 10.1039/c5ay02254a |
‡ The current affiliation of C. Zuliani is Department of Electric and Electronic Engineering, Imperial College London, London SW7 2AZ, UK. |
|
This journal is © The Royal Society of Chemistry 2016 |
Click here to see how this site uses Cookies. View our privacy policy here.