DOI:
10.1039/C5AN02302E
(Paper)
Analyst, 2016,
141, 892-901
Therapeutic drug monitoring in dried blood spots using liquid microjunction surface sampling and high resolution mass spectrometry†
Received
7th November 2015
, Accepted 5th January 2016
First published on 5th January 2016
Abstract
Dried blood spots (DBS) are a versatile and stable tool for direct clinical blood analysis. Ambient high-resolution mass spectrometry is emerging as a method of choice for their quantitative analysis, for instance in therapeutic drug monitoring. Here, we coupled liquid microjunction surface sampling technology, a so-called Flowprobe, with an Orbitrap mass spectrometer and demonstrated the utility of this set-up for direct quantification of multiple drugs in DBS on filter paper. A three-layer set-up that we had introduced earlier enabled introduction of internal standards into DBS. We furthermore took an established point-of-care test system a step further and analyzed disposable test fields for blood glucose monitoring also for Flowprobe-based acetaminophen screening without additional sample preparation. Using as little as 2 μL blood, the method had an LOD of 1 μg mL−1 (coefficient of variation of ≤15%) and acetaminophen recoveries of 82 to 119% for blinded samples, as assessed by LC-MS/MS. Half an hour after ingestions of a single 1000 mg acetaminophen dose, indistinguishable drug levels were measured in three healthy volunteers by LC-MS/MS and Flowprobe-Orbitrap MS analysis of DBS. Flowprobe analysis of DBS was 6- to 100-times more sensitive than corresponding desorption electrospray ionization MS analysis for four drugs. For instance, the LOD for salicylic acid analysis was 0.07 ng mL−1 with Flowprobe measurement. Furthermore, we showed that multi-component analysis of five different substances, which may mimic polypharmacy in diabetes patients, in one blood sample for screening purposes was feasible. Taken together, our study suggests that microjunction surface sampling of DBS on filter paper and disposable point-of-care test fields may be developed into routine methods for near-patient multi-compound therapeutic drug monitoring that may advance blood screening analysis for patients with polypharmacy.
Introduction
Acetaminophen is a widely used analgesic and antipyretic drug. Therapeutic drug monitoring (TDM) of acetaminophen is critical in situations where overdosing from self-medication or abuse is suspected. Depending on risk factors like alcoholism or prolonged fasting, acutely hepatotoxic plasma concentrations (>200 μg mL−1 four hours after ingestion) are reached after oral uptake of >4 g or repeated uptake of >4 g d−1.1,2 In comparison, the therapeutic acetaminophen concentration in blood ranges from 10–20 μg mL−1.3 Severe hepatotoxicity can lead to acute liver failure (ALF), and acetaminophen intoxication accounts for at least 42% of ALF cases in the US.4 Rapid assessment of acetaminophen concentrations is very important, as current therapy can prevent liver injury, if given within 8–12 h after oral overdose.2,5,6 In contrast, severe clinical symptoms are detected no earlier than 24 h after overdosing.5 LC-MS/MS analysis is currently the most common way to quantify biomarkers or therapeutic agents in whole blood.7 However, dried blood spot (DBS) sampling is increasingly becoming a method of choice for TDM,8 incl. that of acetaminophen.9 In this study, we included the three most common prescription-free antipyretics acetaminophen, aspirin,10 and ibuprofen, the common type 2 diabetes mellitus (T2DM) therapeutic tolbutamide11 and the endogenous pre-diabetes indicator uric acid.12 Advantages of DBS sampling include its ease of use (i.e. home sampling), the small sample volume (<20 μL), low cost, enhanced analyte stability and improved safety.8,13
TDM of acetaminophen is especially necessary for groups like geriatric patients and/or diabetes patients, as they are at greater risk of analgesic drug toxicity or drug–drug interaction due to polypharmacy.14–17 For example, administration of high concentrations acetaminophen in combination with aspirin are associated with increased toxicity.18 Furthermore, in T2DM patients with high plasma uric acid concentrations are often treated with the oral hypoglycaemic drug tolbutamide.17 Renal impairment, also indicated by uric acid levels, is common for T2DM patients and can influence the elimination rate of many therapeutics. Parallel intake of antipyretics and hypoglycaemic therapeutics is very likely for diabetes patients and should be closely monitored due to possible drug–drug interactions or overdosing in combinatorial therapy. Multi-component analysis of drug concentrations could simplify risk assessment by rapid DBS screening and subsequent medication evaluation of patients with polypharmacy.
In contrast to obvious DBS advantages, conventional indirect LC-MS/MS quantification of drugs in DBS can be substantially more difficult for laboratories, as it involves multiple handling steps such as punching, extraction, centrifugation, aliquot transfer, drying and dissolution in an appropriate solvent. As a consequence, a lot of attention has been drawn in recent years to methods for direct analyte extraction and ambient analysis of DBS that require minimal or no sample preparation.19–21 Wiseman et al. used desorption electrospray ionization (DESI)-MS on DBS for drug quantification,21 and our team recently introduced pre-manufactured three-layer cartridges, which introduce internal quantification standards, for near-patient TDM.22 Another approach for direct ambient MS analysis of surfaces, are liquid microjunction sampling probes (Fig. 1).23,24 However, to date, their use in DBS analysis has been limited to hemoglobin determination by continuous-flow liquid microjunction surface sampling probe23 and sitamaquine quantification by NanoMate surface sampling probe.24 Simultaneous monitoring of multiple compounds in DBS by direct analysis methods has not been widely explored yet.25 In most cases DBS are prepared and stored on filter paper. Disposable point-of-care (POC) test fields, like those frequently used for blood glucose measurement,26 have not yet been evaluated as DBS sampling devices for direct MS-based blood screening or for application in TDM. Comparable to DBS, their main advantage is patient-autonomous home sampling by finger pricking.
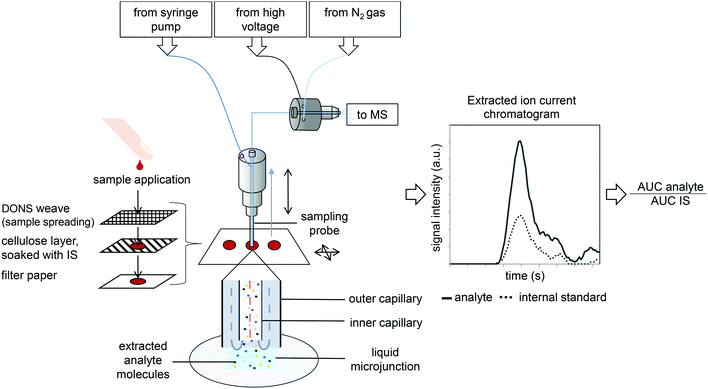 |
| Fig. 1 Schematic representation of the three-layer set-up for sample preparation of DBS and of the Flowprobe in situ liquid micro extraction system for direct and fast MS analysis of analytes on various surfaces. After finger pricking, blood is delivered to a premanufactured three-layer set-up that distributes the blood, introduces the internal standard (IS) and facilitates DBS formation on a filter paper. For Flowprobe analysis, an extraction solvent is constantly delivered to the sample surface via a syringe pump through the outer capillary of the probe tip. In the liquid microjunction formed between the probe tip and sample surface, molecules are extracted and mobilized. This extract is aspirated into the inner capillary and carried on to the ion source. Subsequently, ions are measured by MS. For each analysed spot, an extracted ion chromatogram is obtained with a peak for the characteristic m/z of the substance of interest. For data evaluation the peak areas (AUC) are calculated. If an internal standard (IS) is used, the AUC ratio is determined. | |
In this work, we provide proof-of concept that a Flowprobe liquid microjunction surface sampling probe can be used for direct quantification of acetaminophen in DBS on filter paper. We demonstrate that a three-layer cartridge containing internal standard that we recently presented22 can facilitate TDM and pharmacokinetic studies with a Flowprobe device. Furthermore, we show the feasibility of multi-component quantification experiments that may be useful for blood analysis of geriatric- and diabetes patients with polypharmacy. Our data suggests that a liquid microjunction extraction device may evolve into a rapid and sensitive alternative to perform personalized TDM in specialized facilities after a home-based blood sampling on glucose test strips.
Material and methods
Chemicals, materials and blood samples
LC-MS grade Chromasolv® methanol was purchased from Sigma-Aldrich (Steinheim, Germany) and ammonium solution (25%, UPLC/MS grade) was supplied by Merck (Darmstadt, Germany). Water for all solutions was prepared by an in-house Milli-Q® Synthesis A10™ system (Merck, Schwalbach, Germany). Physiological sodium chloride solution was purchased from Roche/Hitachi (Mannheim, Germany). Acetaminophen (≥98.0–101.0%, powder), ibuprofen (≥98%), sodium salicylate (USP testing spec.), tolbutamide, lithium hydroxide (≥98%, reagent grade) and uric acid (≥99%; BioXtra) were obtained from Sigma-Aldrich or a local pharmacy. [2H4]-acetaminophen and [13C6]-salicylic acid used as internal standards (IS) were from Alsachim (Illkirch Graffenstaden, France). Chemically untreated Whatman™ 589/3 from VWR International (Darmstadt, Germany) was used for DBS analysis. For method validation by LC-MS/MS sample card and prep (SCAP) DBS cards type 2B were purchased from Prolab GmbH (Reinach, Switzerland). Glucose measurement test fields (Accu-Chek® Mobile blood glucose monitoring system) were manufactured and provided by Roche Diabetes Care GmbH (Mannheim, Germany). Both materials were fixed on multi-well microscope slides (5 mm) obtained from Thermo Fisher Scientific (Waltham, USA) using double-sided tape. Human EDTA blood samples were kindly provided by the outpatient services of Roche Diagnostics GmbH (Mannheim, Germany).
Sample preparation of DBS on filter paper and glucose measurement test fields
Acetaminophen and [2H4]-acetaminophen stock solutions (10 mg mL−1 each) were prepared in MeOH/H2O (1
:
1), and further diluted in physiological sodium chloride solution. Blood samples were homogenized by rolling on a multi-axle-rotating-mixer (CAT RM5 Zipperer, Staufen, Germany) for at least 10 min before use. Acetaminophen and IS were diluted in a final step 1
:
100 into whole blood (cacetaminophen = 1, 5, 10, 20, 40, 100, 200, 250 μg mL−1, cIS = 0.75, 1 μg mL−1) and were inverted using a tube rotator (VWR International). Blood spots were generated by dispensing 2 μL blood onto filter paper or glucose measurement test fields. Samples were allowed to dry for at least 2 h at room temperature prior to analysis. Three-layer slides were prepared as described,22 with these modifications: [2H4]-acetaminophen in saline was used as IS (100 μg mL−1) for the second layer, and 4.5 μL blood was applied. Stock solutions of ibuprofen (10 mg mL−1 in methanol), salicylic acid (10 mg mL−1 in saline), tolbutamide (15 mg mL−1 in ethanol) and uric acid (10 mg uric acid and 10 mg LiOH in saline) were prepared for the multi-component analysis. Dilution series were made in the respective solvent. All solutions were diluted in a final step with whole blood 1
:
100 (Table S1†). Calibration curves for acetaminophen, Ibuprofen, salicylic acid, tolbutamide and uric acid were recorded on filter paper using the three-layer method and [13C6]-salicylic acid as IS in the second layer.
Flowprobe and DESI mass spectrometry
All desorption electrospray ionization (DESI) MS experiments were performed as described.22 The continuous in situ micro-extraction and electrospray ionization source Flowprobe (Prosolia, Indianapolis, USA) was mounted on a high resolution Exactive Orbitrap mass spectrometer (Thermo Fischer Scientific, Braunschweig, Germany) (Fig. 1). For single spot analysis, nMotion (version 1.0.0.58, Prosolia) and Xcalibur software (version 2.2.44, Thermo Fisher Scientific) was used. The inner capillary was retracted ≤100 μm relative to the outer capillary and the probe-to-surface distance was set to 100 μm. To provide a continuous flow of extraction solvent (MeOH/H2O + 0.1% ammonia (9
:
1)), a Fusion 100T syringe pump (Chemyx, Stafford, USA) with a 1750 RNR 500 μl syringe from Hamilton Bonaduz (Bonaduz, Switzerland) was used. The solvent flow rate was 15 μL min−1, and gas pressure was 25–30 psi. Nitrogen gas (N5, 99.99%) for solvent aspiration and for ESI, was supplied with 125 ± 5 psi. DBS were extracted for 1 min, followed by at least 20 s of flushing.
All measurements were performed in negative ion mode. Acetaminophen and IS were identified by their exact mass m/z 150.0555 (±5 ppm) and m/z 154.0799 (±5 ppm) for [M − H]−, respectively. Mega 8 (Octanoyl-N-methylglucamide), ibuprofen, salicylic acid, tolbutamide, uric acid and [13C6]-salicylic acid were identified and evaluated according to their exact masses of m/z 320.2085 (±5 ppm), m/z 205.1229 (±5 ppm), m/z 137.0239 (±5 ppm), m/z 269.0960 (±5 ppm), m/z 167.0205 (±5 ppm) and m/z 143.0439 (±5 ppm), respectively. MS settings were: scan range m/z 100–2000, injection time 250 ms, spray voltage 3.5 kV and capillary temperature 250 °C.
Reproducibility of measurement was investigated by analysing DBS (N = 5) containing acetaminophen and [2H4]-acetaminophen as IS (1 μg mL−1 each on filter paper, 0.75 μg mL−1 each on test fields) from one blood donor. 1, 10, 20, 40, 100, 200, 250 μg mL−1 acetaminophen (N = 3 spots per concentration; 1 μg mL−1 IS; whole blood with saline was used as blank) in DBS on filter paper and glucose measurement test fields were used for calibration. Donor-to-donor variability was investigated on each surface for DBS from three donors. To generate donor-independent calibration curves, analyte peak areas were normalized against IS on filter paper and on glucose measurement test fields against Mega 8 detergent, which is a defined component of these. For method verification in a single-blind study, defined acetaminophen concentrations (10, 20, 100, 200 μg mL−1) were spiked into blood, and N = 3 spots were applied on filter paper using the three-layer set-up or glucose measurement test fields. The target acetaminophen concentrations were concealed from the operator during the experiment.
LC-MS/MS
After drying, DBS filter paper was placed on a Harris cutting mat (Sigma Aldrich), and the complete blood spot was removed using a handheld punch (8 mm diameter). In case of glucose measurement strips, the complete test field was cut out. Punch-outs and test fields were vortexing for 20 s in 1 mL MeOH/H2O (9
:
1) and extracted overnight. The supernatant was diluted 1
:
1 with H2O + 0.1% formic acid (= Eluent A). LC-MS/MS analysis was performed on a Waters Acquity UPLC system coupled to a TQD Triple-Quadruple (both Waters, Eschborn, Germany) and an Acquity BEH C18, 100 × 2.1 mm, 1.7 μL column (Eluent B = acetonitrile) at a flow rate of 0.3 mL min−1. The gradient started at 98% A, followed by 70% A till 3 min, 10% A till 8 min, and 98% A from minute 8.5 till 10 min. The injection volume was 10 μL. For selected reaction monitoring, the following precursor [M − H]− to-product ion transitions were used for acetaminophen (m/z 151.7 to 109.8 and 151.7 to 92.7). External calibration was used for quantification. LC-MS/MS data was acquired using MassLynx V4.1 and processed using TargetLynx V4.1 (Waters). LC-MS/MS calibration used acetaminophen (0.2, 0.5, 1, 2, 5, 10, 20, 50, 100, 200, 500 ng mL−1) in methanol. A volume correction factor for filter paper and glucose measurement test field was calculated by dividing the area of the dried blood for each sample by the blood spot area for a 2 μL or 4.5 μL blood spot.
Near-patient endpoint pharmacology study
Blank samples were obtained from three healthy volunteers by finger pricking and direct application of blood onto the three-layer set-up or glucose measurement test fields (N = 3 spots per surface). The study was approved by the medical ethics commission II of Medical Faculty Mannheim of University of Heidelberg (2015-644N-MA) and all study participants were informed about paracetamol. A single oral dose of 1000 mg acetaminophen was administered. Blood samples were collected 30 min post-dose on both surfaces. After drying for at least 2 h at room temperature, DBS where analysed by micro extraction or processed for LC-MS/MS measurement as described above (N = 3 spots per method and surface).
Multi-component analysis
For multi-component analysis, all five substances were spiked into one blood sample. Two evaluate whether sensitivity of detection of one compound was influenced by high concentrations of the others, we defined two concentrations: “High” constitutes the upper limit of a drug's therapeutic concentration in whole blood. “Low” represents a concentration between the lower limit of the therapeutic range and the LOD for a given substance (see Table S2†). “High” concentrations were acetaminophen 20 μg mL−1, ibuprofen 100 μg mL−1, salicylic acid 300 μg mL−1, tolbutamide 150 μg mL−1 and uric acid 72 μg mL−1. “Low” concentrations were 10, 1.56, 2.3, 9.38 and 9 μg mL−1, respectively. It is expected that “Low” concentrations will be affected by ion suppression the most, when all other concentrations are “High”. Therefore, we set up seven different mixtures (Table S2†): in mixtures 1 to 5, one substance is “Low”, whereas all others are “High”. Mixture 6 contained all compounds in their “High”-, mixture 7 all in their “Low” concentration. Additionally, one blank sample with spiked-in solvent was prepared.
Data processing and statistical analysis
Data processing and statistical analysis were essentially done as described.22
The accuracy should not deviate by more than 20% and the precision around the mean value should not exceed 20% coefficient of variation (CV).27 One-way ANOVA (Tukey) test with p < 0.01 and a 99% confidence interval was performed to determine if there were significant differences between more than two measurements.
Results and discussion
Method development: quantification of acetaminophen in DBS by Flowprobe micro extraction
For microjunction surface extraction of acetaminophen from DBS and subsequent quantification by high resolution MS (Fig. 1) we optimized the following parameters: solvent flow rate (15 μl min−1), gas pressure (25–30 psi), probe-to-surface distance (100 μm), retraction of the inner capillary (≤100 μm) as well as the extraction solvent (MeOH/H2O + 0.1% ammonia (9
:
1)) and extraction time (1 min).
The reproducibility of sampling by Flowprobe micro extraction was investigated by analyzing five replicate DBS samples containing constant acetaminophen and IS on filter paper and glucose measurement test fields. On both surfaces %CV was <10 (Fig. S1†) and, hence, reproducible by internationally accepted criteria for bioanalytical validation.27 To investigate donor-to-donor variance and linearity over the therapeutic and hepatotoxic range of acetaminophen, spiked blood samples (N = 3 spots per concentration) of three donors were investigated on filter paper and glucose measurement test fields.
Although acetaminophen peak areas showed linear correlations with nominal acetaminophen concentrations in DBS on both filter paper and glucose measurement test fields (Fig. S2,† coefficient of determination R2 0.9477 to 0.9943), the %CV for individual acetaminophen concentrations in blood samples from different donors was greater than 20%, presumably as a result of matrix effects28 (Fig. S3†). Untreated filter paper rapidly absorbs the extraction solvent that is constantly delivered to the sample surface and thereby prevents the formation of the micro junction. This was not observed for DBS extraction. Presumably, blood components render the filter paper more hydrophobic, thus enabling the formation of the micro junction. For reliable quantification, [2H4]-acetaminophen (1 μg mL−1) was included as IS for experiments on filter paper. Manual addition of IS by donors themselves would have moved the procedure away from simple and fast DBS sampling. Therefore, we used a new three-layer set-up for introduction of IS into DBS on filter paper.22 The detergent Mega 8, a constant constituent of glucose test fields, was used for calibration of DBS measurements on that surface. Donor-independent calibration curves for both surfaces were linear in a concentration range of 1–250 μg mL−1 acetaminophen and displayed correlation coefficients R2 > 0.99 (Fig. 2). LOD and LLOQ were 1 μg mL−1 and 5 μg mL−1 acetaminophen, respectively, for both surfaces. Hence, therapeutically relevant as well as toxic acetaminophen concentrations could be quantified by the Flowprobe-MS method. In ion traps such as the Exactive Orbitrap used in this work, signals can decrease due to c-trap overloading. Especially when analysing complex matrices without post-sampling processing (e.g. by HPLC), this can result in smaller numbers of analyte ions of interest in the trap and provoke ion suppression post-ionization.29–31 Consequently, coupling the Flowprobe system to a HPLC-Exactive-MS, would likely increase sensitivity of Flowprobe-based quantification methods.
 |
| Fig. 2 Donor-independent calibration curves for quantification of acetaminophen in the therapeutic and hepatotoxic concentration range. A, Donor-independent calibration curve for acetaminophen quantification in DBS on filter paper using the three-layer set-up. Acetaminophen to [2H4]-acetaminophen AUC ratios were plotted versus nominal acetaminophen concentrations. Note the linear correlation with R2 = 0.9936 over a concentration range from 5–250 μg mL−1. B, Donor-independent calibration curve for acetaminophen quantification in DBS on glucose measurement test fields. Acetaminophen to Mega 8 AUC ratios were plotted versus nominal acetaminophen concentrations. Note a linear correlation with R2 = 0.9958 over a concentration range from 5–250 μg ml−1. The LOD was 1 μg mL−1 and the LLOQ 5 μg mL−1 on both filter paper and test fields. Therapeutically relevant (10–20 μg mL−1) as well as hepatotoxic acetaminophen concentrations (≥200 μg mL−1) fall within the dynamic range on both investigated surfaces. Error bars indicate mean ± standard deviation (SD). | |
Method verification: good recovery in a single-blind study for quantification of acetaminophen by Flowprobe-Exactive MS
To verify the Flowprobe-Exactive MS method, an experimenter agnostic to the acetaminophen concentrations present in DBS quantified the drug using either the new method or conventional LC-MS/MS. For the latter, DBS were punched out and test fields were cut out, followed by an extraction in 1 mL MeOH/H2O (9
:
1) over night and analysis of the supernatant. Reproducibility of both methods was good (CV% <10) in most cases and acceptable (CV% <15) in all cases (Fig. 3). Recovery rates for Flowprobe micro extraction followed by Exactive-MS were of 80% to 107% and 85% to 107% for filter paper and glucose test fields, respectively. Recovery rates for LC-MS/MS were virtually indistinguishable, namely 85% to 100% and 88% to 104% for filter paper and glucose test fields, respectively. There was no significant difference (P < 0.01) for acetaminophen quantification in DBS on both surfaces using either method (Fig. 3).
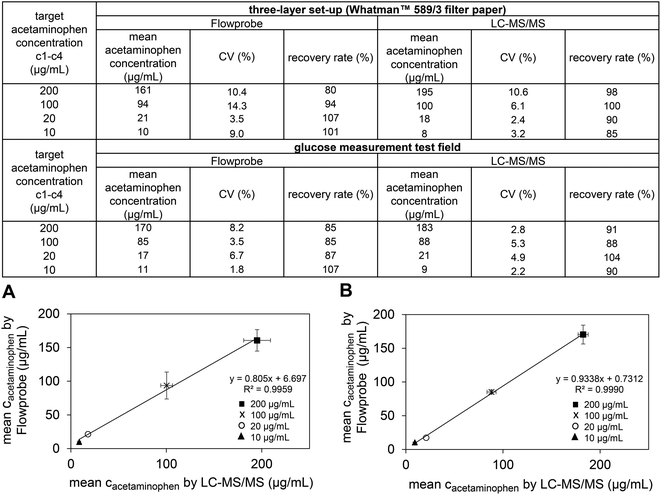 |
| Fig. 3 Method verification for acetaminophen measurements showed good recovery in a single-blind study using Flowprobe-Exactive MS. Acetaminophen concentrations unknown to the operators were quantified in DBS on filter paper using the three-layer set-up or glucose measurement test fields by Flowprobe-Exactive MS or LC-MS/MS. Different acetaminophen concentrations (200 μg mL−1, 100 μg mL−1, 20 μg mL−1, 10 μg mL−1; concealed from the operator during the experiment) were prepared in blood samples. For quantification by Flowprobe micro extraction, the unknown acetaminophen concentrations were calculated based on the corresponding donor-independent calibration curves. For LC-MS/MS analysis, punched-out DBS from filter paper or complete test fields were eluted in solvent prior to quantification. For DBS on filter paper the sampling reproducibility (N = 3) was <15% for both methods and the recovery rates were 80–107% for Flowprobe-MS and 85–100% for LC-MS/MS. For glucose measurement test fields, the sampling reproducibility (N = 3) was <15% for both methods and the recovery rates were 85%–107% for Flowprobe-MS and 88–104% for LC-MS/MS. The coefficient of determination for quantification with Flowprobe-MS versus LC-MS/MS was R2 = 0.9959 on filter paper using the three-layer set-up (A) and R2 = 0.9990 on test fields (B). Error bars indicate mean ± standard deviation (SD). | |
The Flowprobe system can be considered as an alternative for time consuming and extensive LC-MS/MS-based quantification of DBS samples. Consequently, sample run time and cost is reduced, and TDM in DBS is simplified further. Analyses performed on filter paper using the three-layer set-up or on glucose measurement test fields were comparable, and no significant difference in acetaminophen quantification were observed. Our study suggests that, besides their original purpose, glucose measurement test fields could be utilized for home sampling-based TDM by Flowprobe micro extraction.
Near-patient endpoint pharmacology study in healthy volunteers following a single dose of acetaminophen
An endpoint pharmacology study of acetaminophen from healthy volunteers after oral uptake of 1000 mg acetaminophen was performed. Dried blood samples on filter paper and test fields were analysed by Flowprobe micro extraction and LC-MS/MS. Depending on the fasting state of the person, the acetaminophen peak concentration is reached within 0.5–1 h.2,32
Absence of acetaminophen was ensured by analysing blank blood samples. The calculated concentrations of acetaminophen 30 min post-dose based on Flowprobe micro extraction in DBS were 15 μg ml−1 for volunteer 1, 13 μg mL−1 for volunteer 2 and 22 μg ml−1 for volunteer 3. For quantification of DBS by LC-MS/MS the determined acetaminophen concentrations were 14 μg mL−1 for volunteer 1, 12 μg mL−1 for volunteer 2 and 21 μg mL−1 for volunteer 3 (Table 1).
Table 1 Endpoint pharmacology study using dried blood samples from three healthy volunteers following a single dose of acetaminophen. 30 min after oral uptake of 1000 mg acetaminophen by three volunteers, DBS were prepared on glucose test fields and on filter paper using the three-layer set-up. Acetaminophen from DBS was quantified by Flowprobe-Exactive-MS and LC-MS/MS based on the corresponding donor-independent calibration curves. Sampling reproducibility for both methods was <15% and no significant difference was observed for different surfaces. Acetaminophen concentrations are within the therapeutic range of 10–20 μg mL−1. N = 3 spots per method and surface
Volunteer |
Three-layer set-up (Whatman™ 589/3 filter paper) |
Flowprobe™ |
LC-MS/MS |
Mean acetaminophen concentration (μg mL−1), 30 min post-dose |
CV (%) |
Mean acetaminophen concentration (μg mL−1), 30 min post-dose |
CV (%) |
1 |
15 |
10.4 |
14 |
4.4 |
2 |
13 |
10.3 |
12 |
9.6 |
3 |
22 |
1.3 |
21 |
14.7 |
Volunteer |
Glucose measurement test field |
Flowprobe |
LC-MS/MS |
Mean acetaminophen concentration (μg mL−1), 30 min post-dose |
CV (%) |
Mean acetaminophen concentration (μg mL−1), 30 min post-dose |
CV (%) |
1 |
11 |
5.8 |
12 |
7.0 |
2 |
12 |
10.5 |
12 |
6.5 |
3 |
18 |
12.4 |
19 |
3.7 |
The calculated concentrations of acetaminophen 30 min post-dose on test fields were 11 μg mL−1 for volunteer 1, 12 μg mL−1 for volunteer 2 and 18 μg mL−1 for volunteer 3 based on Flowprobe micro extraction analysis. For LC-MS/MS analysis the determined acetaminophen concentrations were 12 μg mL−1 for volunteer 1, 12 μg mL−1 for volunteer 2 and 19 μg mL−1 for volunteer 3 (Table 1).
The coefficient of variation (N = 3) was less than 15% for Flowprobe micro extraction and LC-MS/MS on both surfaces. Both methods correlated well with R2 > 0.98 for the analysis of dried blood on filter paper and test fields (Fig. S4†). Similar to the single-blind-study, there was no significant quantitative difference between measurements on filter paper using the three-layer set-up or test fields for both methods (P < 0.01). The determined acetaminophen concentrations in blood samples 30 min post-dose were within the expected therapeutic range of 10–20 μg mL−1.3 Differences between the volunteers can be explained by individual fasting states, age, weight and sex.2,33
Based on the obtained data it can be suggested, that the Flowprobe micro extraction system is an alternative for commonly used more time consuming LC-MS/MS analysis in pharmacology studies.26,34,35 Both surfaces, filter paper and test fields, are suitable to perform a personalized endpoint pharmacology study of acetaminophen with blood samples generated by the patient. In case of acetaminophen intoxication the blood sampling should be performed 4 h post-ingestion, as the intoxication can lead to delays in peak concentrations.32
Improved sensitivity with Flowprobe-Exactive MS compared to desorption electrospray (DESI) MS enabled multi-component analysis
Since we had earlier observed limited sensitivity for salicylic acid quantification using DESI-Exactive MS,22 we wondered if longer extraction times in the Flowprobe set-up improved sensitivity. To this end we analysed dilution series of salicylic acid with both ion sources (but identical sample preparation and mass analyser) and noted a 100-fold improvement of the LOD (to 0.07 μg mL−1) with Flowprobe-Exactive MS (Table 2; Fig. S6†). The slopes and CVs (≤15%) of the calibration curves were comparable. We hypothesized that micro extraction in combination with the ESI process leads to higher ionization efficiency, thereby improving sensitivity. To examine whether this finding was substance-specific, we also compared acetaminophen analysis performance with both ion sources. However, the LOD for acetaminophen in DBS was only 6-fold improved (5 μg mL−1 with Flowprobe-MS compared to 30 μg mL−1 with DESI-MS measurements; Fig. S5;†Table 2). This result suggested that the Flowprobe micro extraction source generally provided more sensitive small molecule quantification in DBS compared to the commercially available 2D DESI source, but that the sensitivity difference was substance-specific.
Table 2 Comparison of LODs for various exogenous and endogenous substances determined by Flowprobe and DESI mass spectrometry. Analysis of acetaminophen, ibuprofen, salicylic acid, tolbutamide and uric acid showed a 4- to 100-fold increased sensitivity when using the Flowprobe source. The highest improvement in sensitivity was observed for salicylic acid (LOD 0.07 μg mL−1 instead of 8 μg mL−1). DESI-MS analysis was performed in accordance to Siebenhaar et al. for all five substances
|
LOD (μg mL−1) |
Fold-change |
|
Flowprobe |
DESI |
Experiment for LOD determination was optimized to the m/z of acetaminophen, i.e. the MS was tuned to m/z 150.06 and solvent MeOH/H2O + NH3 (9 : 1 + 0.1%). Determination of the LOD for acetaminophen (DESI), ibuprofen, SA, tolbutamide and uric acid was achieved by optimization to m/z 137.0239 (salicylic acid) using MeOH/H2O (1 : 1).
|
Acetaminophen |
1.0a |
30 |
6 |
Ibuprofen |
1.6 |
20 |
13 |
Salicylic acid |
0.07 |
8 |
>100 |
Tolbutamide |
1.2 |
19 |
16 |
Uric acid |
4.5 |
20 |
4 |
Nevertheless, we also sought to investigate whether multicomponent analysis and, hence, polypharmacy monitoring was possible using the three-layer setup in combination with Flowprobe-Exactive MS. For future clinical or industrial applications, multi-component analysis of several different medications or physiological parameters is an interesting diagnostic option. As an exemplary application, we investigated five compounds implicated in polypharmacy for type 2 diabetes mellitus patients: the oral sulfonylurea hypoglycemic drug tolbutamide, endogenous uric acid (levels are increased in those patients36) and three common prescription-free pain killers (acetaminophen, ibuprofen and salicylic acid) were investigated.
For those five substances, the LODs were determined by DESI- and Flowprobe-MS. All experiments were performed using the three-layer setup with 13C-labelled salicylic acid as internal standard contained in the second layer. Improved sensitivity was observed for all substances (Table 2). Calibration curves for DESI- and Flowprobe-MS quantification are depicted in Fig. S8 and S7,† respectively. For all calibration curves, R2 was >0.99. The CVs of Flowprobe-MS measurements were <8% for acetaminophen, ≤13% for ibuprofen, ≤13% for tolbutamide and ≤14% for uric acid. LODs were calculated to be 5, 1.56, 1.17 and 9 μg mL−1, respectively. The highest improvement in sensitivity was obtained for salicylic acid (see above), whereas it was 4- to 16-fold for the other compounds tested.
A prerequisite for multi-component analysis, we were able to detect all five substances in parallel (Fig. S9†). Recovery rates were in the range of 124% (acetaminophen) to 91% (salicylic acid) when all other substances (at their upper therapeutic or physiological concentrations) were present in the same sample. CVs for all substances were below 15%. For low analyte concentrations (Fig. S9,† mixture 7) ibuprofen, salicylic acid and tolbutamide showed good recovery rates between 89% (tolbutamide) and 122% (ibuprofen) with CVs ≤21%. However, acetaminophen concentrations were generally overestimated, and uric acid signals were completely suppressed. To get a more complete picture of ion suppression, we analyzed five compound mixtures, in which a “low” concentration of one analyte was determined in the presence of “high” concentrations of the other four substances (Table S2†). In this setting, no significant ion suppression effects were observed for salicylic acid and tolbutamide. Recovery rates for these “low” concentrations ranged from 84% to 114% for salicylic acid and 83% to 114% for tolbutamide (Fig. 4, green and black). CVs were ≤14%. For “high” uric acid we obtained good recovery rates between 89% and 108% (CV ≤18%) (Fig. 4, red). Acceptable results were also obtained for ibuprofen (Fig. 4, orange), with recovery rates ranging from 119% to 129% for “high” ibuprofen. In contrast, “low” uric acid concentrations were not detectable, probably because of ion suppression by the other spiked-in substances. Acetaminophen analysis was also strongly affected by the other four substances. Variance (CVs ≤24%) was higher than in single component analysis (Fig. 4, blue) and acetaminophen concentrations were consistently overestimated (recovery rates up to 200%, even when all other substances were present in “low” concentrations).
 |
| Fig. 4 Multi-component analysis of acetaminophen, ibuprofen, salicylic acid, tolbutamide and uric acid in human whole blood using Flowprobe micro extraction coupled to Exactive high resolution MS. Five different sample mixtures spiked into whole blood with varying analyte concentrations were investigated on filter paper using the three-layer setup. Nominal concentrations are indicated by short lines. Mean analyte concentrations (N = 3 Spots/datapoint; ±SD)) are represented by filled circles. Salicylic acid (green) and tolbutamide (black) showed good recovery rates in the range of 83% up to 114% with coefficients of variation ≤14%. Uric acid (red) was measured with recovery rates between 89% and 108% with CV% ≤18 for analyte mixtures with high uric acid concentrations. Low uric acid concentrations were not detectable, most probably due to ion suppression effects. Due to method optimization to salicylic acid analysis (tuned to m/z 1 370 239 and using MeOH/H2O as spray solvent), LOD and CV% for acetaminophen measurement were worse (5 μg mL−1 and <24%, respectively; blue) compared to previous results. High acetaminophen concentrations were overestimated (116% to 129% recovery), as was ibuprofen (orange; but with lower variance CV% ≤14). Low acetaminophen concentrations were vastly exaggerated (∼200% recovery of the nominal concentration). Flowprobe-MS parameters were: nitrogen gas pressure 29 psi; spray voltage −3.5 kV; solvent flow rate 15 μL min−1; mass resolution 50 000; max. ion inlet time 250 ms; inlet capillary temperature 250 °C. DBS were analyzed in spot sampling mode with 10 s sampling time and a total acquisition time of 1.07 min. Solvent composition was 1 : 1 methanol to water. | |
Conclusion
For the first time Flowprobe micro extraction coupled to an Orbitrap Exactive MS with high resolving power was successfully implemented and verified for analysis of DBS on filter paper and on glucose measurement test fields to perform near-patient TDM and endpoint pharmacology studies. A similar set-up has recently been used for drug analysis in tissue sections in conjunction with Orbitrap XL-based MS imaging by Prideaux et al.,37 suggesting that this set-up may have wider utility. When applied to DBS, one major advantage is that blood samples can be collected by patients themselves or their guardians with minimal training, requiring substantially smaller blood volumes than with conventional methods. The three-layer set-up used to introduce internal standard into DBS on filter paper represents a sampling option that could conceivably be pre-manufactured. Besides DBS analysis, this study also demonstrated that existing disposable POC systems such as frequently used test fields for blood glucose monitoring could, in addition, be used for TDM and pharmacology studies. For better patient comfort, near patient TDM of acetaminophen would not need any additional sample preparation. This approach might be applicable also to other disposable test strips used for POC.
Furthermore, we demonstrated that use of the Flowprobe micro extraction source significantly improved the LOD of acetaminophen by 6-fold and that of salicylic acid even by 100-fold. With this improvement in comparison to DESI analysis, we achieved parallel quantification of three different commercially available analgesics (acetaminophen, ibuprofen, salicylic acid), the hypoglycemic drug tolbutamide for type 2 diabetes and the endogenous metabolite uric acid, a combination that may resemble polypharmacy encountered in these patients. In a proof-of-concept study, we showed that parallel monitoring of multiple substances may be possible. Although this study clearly pinpointed the limitations of multicomponent quantitative analysis by Flowprobe-Exactive MS that result from ion suppression in the absence of additional HPLC separation, our results suggest that such multicomponent analysis could at least serve screening or semi-quantitative purposes in polypharmacy studies for specific target patient groups. It is evident though that similar ion suppression effects may hamper quantitative statements in MS imaging experiments using a similar experimental set-up. In case of DBS, ionization effects would have to be investigated in detail before applying the method to other medications. Moreover, other surface extraction methods such as the Advion NanoMate system may substantially increase sensitivity and reproducibility when used in combination with the three-layer method. The NanoMate system reduces matrix composition by additional HPLC separation between the micro extraction and the ionization step24 and thereby like decrease ion suppression. In subsequent studies additional aspects contributing to analyte distribution in DBS, e.g. influence of differing hematocrit values, will have to be evaluated for micro extraction systems. Abu-Rabie et al. recently showed, that an automated internal standard spray addition technique to DBS samples prior to extraction nullifies the effect of hematocrit-based assay bias.38,39 Further investigations are necessary to investigate and confirm this effect also for flowprobe analysis using the three-layer set-up. For example, the stability of analytes in DBS cards or analyte degradation during the extraction process should be considered for specific applications.
Flowprobe micro extraction- and LC-MS/MS methods correlated well. This finding along with its ease of use and substantial time savings demonstrate the potential of the Flowprobe system as an alternative for commonly used LC-MS/MS analysis in quantitative DBS screening. We do not consider the Flowprobe micro extraction system as a standard technique in its present form, but it could be used for special purpose with obvious benefits. Moreover, the optimal drying time was 2 h for the three-layer method using DESI-MS.22 Similar investigations of the drying time and its impact on recovery rates need to be performed for micro extraction systems. Shorter drying times would be desirable for higher throughput of automated methods, but microjunction formation may be compromised when blood spots are not entirely dry. In general, the method reduces bioanalytical effort associated with DBS analysis by eliminating the manual extraction, further dilution- and internal standard-spiking steps completely. The method (cycle times of 1.5 min for acetaminophen) might be applied, if rapid assessment of drug concentrations is required. The small required blood volume is an advantage for pharmacokinetic studies in animals or infants. For better sensitivity and quantification, we suggest, however, to combine the Flowprobe set-up with chromatographic separation of the DBS extract.
Acknowledgements
M.S., V.T. and V.H. are employees of Roche Diabetes Care GmbH, Mannheim. The authors thank Dr Tobias Müller (SGS, Germany) for performing the LC-MS/MS reference measurements and Anke Senz for the assistance in performing the single-blind-study. We also acknowledge Dr Kai Küllmer (Roche Diabetes Care, Germany) for helpful comments on the manuscript.
References
- R. C. Dart, A. R. Erdman, K. R. Olson, G. Christianson, A. S. Manoguerra, P. A. Chyka, E. Martin Caravati, P. M. Wax, D. C. Keyes, A. D. Woolf, E. J. Scharman, L. L. Booze and W. G. Troutman, Clin. Toxicol., 2008, 44, 1–18 Search PubMed.
- L. F. Prescott, Br. J. Clin. Pharmacol., 1980, 10, 291S–298S CrossRef PubMed.
-
L. L. Brunton, B. A. Chabner and B. C. Knollman, Goodman and Gilman's The Pharmacological Basis of Therapeutics, McGraw-Hill, New York, 2011 Search PubMed.
- A. M. Larson, J. Polson, R. J. Fontana, T. J. Davern, E. Lalani, L. S. Hynan, J. S. Reisch, F. V. Schiødt, G. Ostapowicz, A. O. Shakil and W. M. Lee, Hepatology, 2005, 42, 1364–1372 CrossRef CAS PubMed.
- L. J. Chun, M. J. Tong, R. W. Busuttil and J. R. Hiatt, J. Clin. Gastroenterol., 2009, 43, 342–349 CrossRef CAS PubMed.
- P. I. Dargan and A. L. Jones, Crit. Care, 2002, 6, 108–110 CrossRef PubMed.
-
M. S. Lee, W. Li, P. Wong and C. A. James, Dried Blood Spots - Application and Techniques, John Wiley & Sons, Inc., New Yersey, 2014 Search PubMed.
- A. J. Wilhelm, J. C. G. den Burger and E. L. Swart, Clin. Pharmacokinet, 2014, 53, 961–973 CrossRef CAS PubMed.
- W. Li, J. P. Doherty, K. Kulmatycki, H. T. Smith and F. L. S. Tse, Bioanalysis, 2012, 4, 1429–1443 CrossRef CAS PubMed.
- R. Nirogi, V. Kandikere, K. Mudigonda, D. Ajjala, R. Suraneni and P. Thoddi, Arzneimittelforschung, 2011, 61, 301–311 CrossRef CAS PubMed.
- R. E. Ferner, M. L. Antsiferov, A. W. Kelman, K. G. Alberti and M. D. Rawlins, Eur. J. Clin. Pharmacol., 1991, 40, 163–168 CrossRef CAS PubMed.
- S. Kodama, K. Saito, Y. Yachi, M. Asumi, A. Sugawara, K. Totsuka, A. Saito and H. Sone, Diabetes Care, 2009, 32, 1737–1742 CrossRef CAS PubMed.
- J. Déglon, A. Thomas, P. Mangin and C. Staub, Anal. Bioanal. Chem., 2012, 402, 2485–2498 CrossRef PubMed.
- E. R. Hajjar, A. C. Cafiero and J. T. Hanlon, Am. J. Geriatr. Pharmacother., 2007, 5, 345–351 CrossRef PubMed.
- R. P. Austin, Diab. Spectr., 2006, 19, 13–16 CrossRef.
- A. Emslie-Smith, J. Dowall and A. Morris, Br. J. Diabetes Vasc. Dis., 2003, 3, 54–56 CrossRef.
- M. Runganga, N. M. Peel and R. E. Hubbard, Clin. Interventions Aging, 2014, 9, 1453–1462 Search PubMed.
- L. E. Schmidt and K. Dalhoff, Br. J. Clin. Pharmacol., 2002, 53, 535–541 CrossRef CAS PubMed.
- P. Abu-Rabie and N. Spooner, Bioanalysis, 2011, 3, 2769–2781 CrossRef CAS PubMed.
- H. Wang, J. Liu, R. G. Cooks and Z. Ouyang, Angew. Chem., Int. Ed., 2010, 49, 877–880 CrossRef CAS PubMed.
- J. M. Wiseman, C. A. Evans, C. L. Bowen and J. H. Kennedy, Analyst, 2010, 135(4), 720–725 RSC.
- M. Siebenhaar, K. Küllmer, N. M. de Barros Fernandes, V. Hüllen and C. Hopf, Anal. Bioanal. Chem., 2015, 407, 7229–7238 CrossRef CAS PubMed.
- G. J. Van Berkel and V. Kertesz, Rapid Commun. Mass Spectrom., 2013, 27, 1329–1334 CrossRef CAS PubMed.
- V. Kertesz and G. J. Van Berkel, J. Mass Spectrom., 2010, 45, 252–260 CrossRef CAS PubMed.
- N. M. Lafrenière, S. C. Shih, P. Abu-Rabie, M. J. Jebrail, N. Spooner and A. R. Wheeler, Bioanalysis, 2014, 6, 307–318 CrossRef PubMed.
- J. Hönes, P. Müller and N. Surridge, Diabetes Technology & Therapeutics, 2008, 10, S-10 Search PubMed.
- V. P. Shah, K. K. Midha, J. W. A. Findlay, H. M. Hill, J. D. Hulse, I. J. McGilveray, G. McKay, K. J. Miller, R. N. Patnaik, M. L. Powell, A. Tonelli, C. T. Viswanathan and A. Yacobi, Pharm. Res., 2000, 17, 1551–1557 CrossRef CAS.
- A. Furey, M. Moriarty, V. Bane, B. Kinsella and M. Lehane, Talanta, 2013, 115, 104–122 CrossRef CAS PubMed.
- H. Henry, H. R. Sobhi, O. Scheibner, M. Bromirski, S. B. Nimkar and B. Rochat, Rapid Commun. Mass Spectrom., 2012, 26, 499–509 CrossRef CAS PubMed.
- C. M. Chavez-Eng, M. L. Constanzer and B. K. Matuszewski, J. Pharm. Biomed. Anal., 2004, 35, 1213–1229 CrossRef CAS PubMed.
- C. Pistos, M. Koutsopoulou and I. Panderi, Anal. Chim. Acta, 2004, 514, 15–26 CrossRef CAS.
- S. White and S. H. Y. Wong, Clin. Chem., 1998, 44, 1110–1123 CAS.
- J. A. H. Forrest, J. A. Clements and L. F. Prescott, Clin. Pharmacokinet., 1982, 7, 93–104 CrossRef CAS PubMed.
- L. Tretzel, A. Thomas, H. Geyer, P. Delahaut, W. Schänzer and M. Thevis, Anal. Bioanal. Chem., 2015, 407, 4709–4720 CrossRef PubMed.
- M. Wagner, D. Tonoli, E. Varesio and G. Hopfgartner, Mass Spectrom. Rev., 2014, 1–78 Search PubMed.
- N. Katsiki, N. Papanas, V. A. Fonseca, E. Maltezos and D. P. Mikhailidis, Curr. Pharm. Des., 2013, 18, 4930–4937 CrossRef.
- B. Prideaux, M. S. ElNaggar, M. Zimmerman, J. M. Wiseman, X. Li and V. Dartois, Int. J. Mass Spectrom., 2015, 377, 699–708 CrossRef CAS PubMed.
- P. Abu-Rabie, P. Denniff, N. Spooner, B. Z. Chowdhry and F. S. Pullen, Anal. Chem., 2015, 87, 4996–5003 CrossRef CAS PubMed.
- P. Abu-Rabie, N. Spooner, B. Z. Chowdhry and F. S. Pullen, Bioanalysis, 2015, 7, 2763–2775 CrossRef CAS PubMed.
Footnote |
† Electronic supplementary information (ESI) available. See DOI: 10.1039/c5an02302e |
|
This journal is © The Royal Society of Chemistry 2016 |