DOI:
10.1039/C6RA11231E
(Paper)
RSC Adv., 2016,
6, 58212-58217
Towards DNA sensing polymers: interaction between acrylamide/3-(N,N-dimethylaminopropyl)-acrylamide and DNA phage λ at various N/P ratios†
Received
30th April 2016
, Accepted 9th June 2016
First published on 10th June 2016
Abstract
The present study strongly relates to ongoing research on the development of cationic polymers which are of great interest due to their enormous potential for biomedical applications, especially as non-viral vectors for gene therapy, antimicrobial agents and active components in DNA sensing devices. The current paper demonstrates that a functional group approach can be successfully realized in a free-radical copolymerization process to prepare cationic copolymers with a desired composition of amine groups, which can be protonated in water thus, providing electrostatic interactions between a polycation and DNA. Three replicas of the cationic copolymer, acrylamide/3-(N,N-dimethylaminopropyl)-acrylamide (AADMAPA), were synthesized using this strategy. The values of average molecular mass and polydispersity index, are similar for the replicas, averaged to 24
000 ± 2000 g mol−1 and 1.5 ± 0.1, respectively. The copolymer composition according to 1H-NMR (D2O), was corresponded to the molar ratio of initial monomers. The dynamic light scattering studies and zeta potential measurements confirmed that in water positively charged AADMAPA/DNA polyplexes are formed at N/P > 2.2: the formed particles have bimodal distributions with the average diameters of 70 and 700 nm. Zeta potential measurements indicated that the point of zero charge (isoelectric point) is close to N/P ≅ 2.2. According to the atomic force microscopy positively charged AADMAPA/DNA polyplexes have axially symmetric shapes.
Introduction
Why does a novel biomedical high-tech area pay special attention to cationic polymers? The unique physico-chemical and biochemical properties of cationic polymers give an answer to this question. Due to the presence of positively charged groups cationic polymers can interact with DNA's negatively charged phosphates groups by electrostatic attraction, resulting in the formation of compact, spherical-like complexes (polyplexes). These polymer systems can be employed in a number of various biomedical applications such as DNA sensing, controlled delivery of therapeutic agents or non-viral vectors for gene therapy etc.1–17 Due to ability of cationic polymers to condense large genes into smaller structures via electrostatic interactions, they can be used to transfer genes into cells.13,14 Thereby, a large number of various cationic polymers have been synthesized and characterized. The most widely used and studied cationic polymers include poly(ethyleneimine) (PEI) poly(lysine) (PLL), poly(2-(N,N-dimethylamino)ethyl methacrylate)] (PDMAEMA); poly(vinyl amine) (PVA).18–22 Recently, it has been suggested that novel homo and block copolymers based on poly(vinyl benzyl trimethylammonium chloride) may be used as gene delivery vectors.14
The majority of the cationic polymers, bearing an amine function, can be protonated; the relative number of the protonable amines differs for each polymer. The resultant characteristics (size and size distribution, transfection efficiency, stability, toxicity, etc.) of polycation/DNA polyplexes depend on many parameters including chemical structure and molar mass of an initial polycation, concentration of DNA solution, polymer to DNA molar ratio (N/P ratio), pH, etc.23,24 Moreover, the average size and the surface charge of the formed polyplexes as well as initial polymer architecture are known to have great impact on their transfection efficiency and biocompatibility.25,26 Besides, it was demonstrated that the greater the distance between the charged groups in a polycation, the higher its ability to deliver DNA into the cell safely and efficiently.27 On the other hand, it is clear that with higher amount of positively charged groups, the efficiency of DNA binding to a cationic copolymer should increase. Therefore, a synthetic strategy that permits an engineering of cheap, well-structured polycations with desired DNA holding properties has to be developed to be used in different applications of modern biomedicine such as DNA sensing and others. The above conclusions motivated us to employ a functional group approach in combination with free-radical copolymerization for preparing a new cationic copolymer with a well-controlled contribution of amine groups. Previously, using this synthetic strategy (Fig. 1) a water soluble bioactive cationic copolymer – acrylamide/3-(N,N-dimethylaminopropyl)-acrylamide (AADMAPA) was synthesized and its ability to form stable complexes with DNA was demonstrated.28,29 In relation to this result two fundamental questions have arisen: (1) does this synthetic procedure permit reproducible preparation of AADMAPA with a desired composition and (2) what is the correlation between N/P values and characteristics (size and charge) of AADMAPA/DNA complexes formed in water?
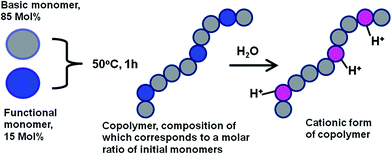 |
| Fig. 1 Schematic presentation of a functional group approach in combination with a free-radical copolymerization process for preparing cationic polymers with desired DNA trapping properties. | |
Here, using three synthetic replicas, we demonstrate that the developed approach for the preparation of AADMAPA is a well reproducible synthetic method which can be used for engineering new bioactive polymers with desired DNA trapping properties. Furthermore, the complexation behaviour of AADMAPA with DNA phage λ in water at various N/P ratios is discussed. In particular, the article reports physico-chemical properties of both AADMAPA and AADMAPA/DNA complexes studied by different characterisation methods: size exclusion chromatography (SEC), dynamic light scattering (DLS) and atomic force microscopy (AFM).
Experimental
Synthesis of the bioactive cationic copolymer: acrylamide/3-(N,N-dimethylaminopropyl)-acrylamide (AADMAPA)
N,N,N′,N′-Tetramethylethylenediamine (TEMED) (12 μl, 0.08 mmol) was added to a mixture of acrylamide (AA) and N-(3-dimethylaminopropyl)-acrylamide (DMAPA) preliminary dissolved in water (9.5 ml); the molar ratio AA
:
DMAPA was 0.85
:
0.15. The mixture was heated to 50 °C under stirring and ammonium persulfate (0.004 g, 0.0175 mmol) dissolved in the 0.5 ml of water, was added to the reaction mixture. Once the mixture was stirred for one hour at 50 °C, it was cooled down to the room temperature and dialyzed against low molecular weight (up to 10 kDa) fractions. The final fraction contained water solution of AADMAPA. Water was then removed by freeze-drying. Three replicas of this synthetic protocol have been performed (see Table 1). The copolymer was generated in high yields in the range from 71% to 76%. The chemical structure of the copolymer is shown in Fig. 2.
Table 1 Data on three replicas of the synthetic protocol developed for reproducible preparing acrylamide/3-(N,N-dimethylaminopropyl)-acrylamide copolymer (AADMAPA)
Replica |
Acrylamide (AA) |
N-(3-Dimethylaminopropyl)-acrylamide (DMAPA) |
Compositiona |
Yield% |
Grams |
10−3 mol |
Grams |
10−3 mol |
AA |
DMAPA |
Determined by 1H-NMR (D2O). |
1 |
0.300 |
4.22 |
0.116 |
0.75 |
0.85 |
0.15 |
71 |
2 |
0.330 |
4.64 |
0.128 |
0.82 |
0.85 |
0.15 |
76 |
3 |
0.360 |
5.06 |
0.140 |
0.89 |
0.85 |
0.15 |
74 |
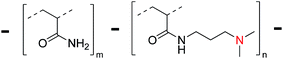 |
| Fig. 2 Skeletal formula of AADMAPA. | |
NMR spectra
Proton NMR spectra were recorded in D2O using a Bruker Model ARX (300 MHz) spectrometer. 1H-NMR data were additionally confirmed by copolymer's IR spectra collected on a Bruker Tensor 37 in the range 3500–1000 cm−1 (Table 2, Fig. 1 SI and 2 SI, ESI†).
Table 2 1H-NMR and IR spectral characteristics of AADMAPA
1H-NMR (D2O) spectrum |
IR (KBr) spectrum |
Chemical shift (δ) ppm |
Proton assignment |
Vibration band, ν, cm−1 |
Band assignment |
1.28–1.70 (broad) |
C 2 (propyl group, polymer backbone) |
1180–1040 |
N–C 2 |
3350–3205 |
N–H (NH2, NH) |
2.09 (broad) |
C (polymer backbone) |
2933 |
C–H (CH2) |
2870 |
C–H (CH3) |
2.32 (broad singlet) |
N(C 3)2 |
1665 |
C O |
1450–1320 |
C–H (CH, CH2, CH3) |
2.53, 3.10 (broad) |
N–C 2 |
1180–1040 |
C–N, C–C |
Size exclusion chromatography
Size exclusive chromatography was performed on SEC system (Waters Chromatography Division) using Hysdrogel (120 A and 250 A) column, mobile phase with 5% NaH2PO4 and 4.3% CH3CN, pH 4, flow rate 0.8 ml min−1, T = 36 °C, dRI M410 and UV M490 detectors, polystyrene calibration.
Preparation of AADMAPA/λ-phage DNA complexes
The DNA complexation experiments were conducted using λ-phage DNA (48.5 kbp, Fermentas). The corresponding AFM image of DNA is shown in Fig. 3 SI A (ESI).† The AADMAPA/λ-phage DNA complexes at different N/P ratios were prepared by mixing the estimated amount of the aqueous DNA solution with the concentration of 300 μg ml−1 (see Table 1 SI, ESI†) and the copolymer water solution (1 ml, 100 μg ml−1). After an addition of λ-phage DNA, the mixture was extensively shacked and the polyplexes were then incubated for 30 min at room temperature.
Particle size and zeta-potential measurements
The particles size and the zeta-potential measurements were performed using DLS (Zetasizer Nano ZS) with Dispersion Technology Software V.5.10 (Malvern Instruments Limited). Each sample was measured 8 times with 6 single runs per measurement. All measurements were performed in water at pH 6.5 and 25 °C.
Atomic force microscopy
Samples for AFM were prepared on the surface of freshly cleaved mica. A 2.5 μl droplet of the solution (polyplexes or initial DNA) was deposited onto mica and dried at room temperature for 24 h. AFM experiments were performed in air, at room temperature and in tapping mode using scanning probe microscope FemtoScan (Advanced Technologies Center) with 15 series MikroMasch cantilevers. Their average resonance frequency was 325 kHz, and the tip radius was about 10 nm. AFM images were processed and analysed by FemtoScan Online software.
Results and discussion
Preparation of the cationic polymer AADMAPA
As was mentioned in the introduction, very important feature of synthetic cationic polymers is that they can be synthesized to match required properties for the optimization of the gene delivery process or to be used in other applications. The synthetic strategy, used in our research, fully justifies this approach. Recently, our preliminary data had demonstrated that through controlled free radical copolymerization, using monomers such as AA and DMAPA, water soluble cationic acrylamide-based copolymer with a desired amount of functional groups can be prepared.28 In the context of our synthetic approach, one of the main questions is the reproducibility of the developed synthetic method. To address this question, three replicas of AADMAPA copolymers were prepared by controlled free radical copolymerization using red–ox system of ammonium persulfate – TEMED as a source of free radicals. All replicas of AADMAPA were characterized by SEC, 1H-NMR and IR spectra. The results are summarized in Tables 1 and 2 (see the Experimental part). All three replicas of AADMAPA were prepared in high yields (71–76%, Table 1). The values of average molar mass and polydispersity index are similar for the three replicas (Mn = 24
000 ± 2000 g mol−1, PDI = 1.5 ± 0.1). In Table 2, the characteristic signals of 1H-NMR and IR spectra for the polymer backbone and functional groups of the replicas are shown. Three samples of AADMAPA were found to have the same composition: the molar ratio of AA to DMAPA was determined as 0.85 to 0.15. This ratio was justified by the reference to integral intensities of the 1H-NMR signals corresponding to the protons of CH and N(CH3)2 groups. Importantly, the copolymer composition is corresponded to the molar ratio of the initial monomers. This outcome, coupled with the reproducible values of the yield of the cationic copolymer and its composition confirms that the developed synthetic strategy can be applied for the engineering of polycations with the desired amount of functional groups.
The complexation behavior of AADMAPA with DNA phage λ
The study of the AADMAPA/DNA polyplex formation is crucial for further enhancement of the rational design and functionality of the polymer and as a consequence its applications. Since positively charged polycation/DNA complexes may be used for gene transfection, the main attention was given to the definition of the N/P values, at which stable, positively charged AADMAPA/DNA phage λ complexes can be formed. The complexes were formed in water at different N/P ratios (see Experimental section and Table 1 SI, ESI†). Both Fig. 3A and Table 2 SI (ESI)† clearly show that for N/P ratios in range of 3.3 to 10, zeta-potential is positive and practically constant (20–21 eV) which, in turn, indicates the formation of positively charged AADMAPA/DNA complexes. The corresponding size distribution is bimodal with the peaks at ≈70 and ≈700 nm (Fig. 3B). The hydrodynamic radius of the initial λ-phage DNA is about 130 nm, and slightly differs in the concentration range from 25 μg ml−1 to 100 μg ml−1 (Fig. 4 SI, ESI†). In this context, it can be assumed that the first peak can be assigned to the complexes of AADMAPA with the single DNA molecule. Such initial complexes tend to aggregate into the higher order structures resulting in the second peak observed at 700 nm. According to literature data, polyplexes with the sizes around 80 nm demonstrate the highest transfection efficiency in comparison with complexes of other sizes.18
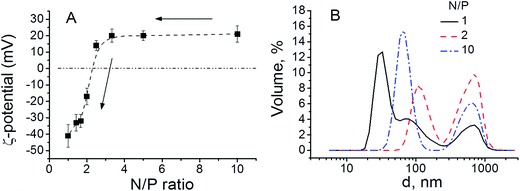 |
| Fig. 3 (A) Zeta-potential of the polyplexes at different N/P ratios and (B) size distribution of polyplexes at various N/P ratios. | |
When N/P values decrease from 3.3 to 1, zeta-potential decreases dramatically; the effective charge of the resulting complexes changes from positive to negative values. The zeta-potential measurements indicate that nearly all the DNA chains are complexed with AADMAPA when N/P ratio reaches ≈2.2. This correlates well with previously reported data, which have repeatedly shown that the DNA molecules are completely complexed with polycations containing amine groups at N/P ≈ 2–3.30,31 Fig. 3B shows that the bimodal size distribution of the AADMAPA/DNA nanocomplexes is observed in the range 1.5 ≤ N/P ≤ 10, when N/P < 1.5 the size distributions of the particles demonstrates three distinct peaks at ≈20 nm, ≈100 nm and ≈700 nm. A new particle population with a very low average diameter could be a product of the polyplexes decomposition.
Polyplex morphology
The morphology of the polyplexes was investigated by AFM, and the corresponding results are presented in Fig. 4. AFM data demonstrated that a smooth film was formed on the surface of mica after drying the solution of the initial copolymer (Fig. 3 SI B, ESI†). The mean square roughness of the film is about 0.4 nm. Any signs of the copolymer self-aggregation were not observed.
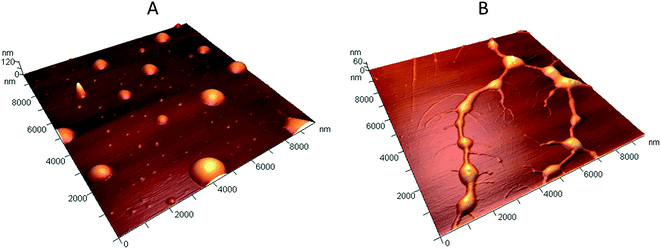 |
| Fig. 4 3D AFM images of (A) the positively charged polyplexes with N/P ratio of 5, (B) the negatively charged polyplexes with N/P ratio of 1.25. | |
DLS and zeta-potential measurements showed that stable, positively charged polyplexes are formed at N/P ratio in the range from 3.3 to 10. In this context, the AFM study was carried out for samples with N/P ratio of 5. The obtained results revealed the axial symmetry of the polyplexes and their multi-modal size distribution (Fig. 4A). It can be assumed that the large particles with the diameter of 500–1000 nm and the height of 30–80 nm are agglomerates of small/initial complexes with the diameter of 50–300 nm and the height of 1–25 nm, which is in good correlation with the DLS data. The latter may consist of a single DNA molecule surrounded by the positively charged copolymer. Most likely, the particles have a spherical shape in the solution, which is flattened due to the adsorption on mica. The same effect was observed when polyplexes were investigated by AFM in liquid environment.32 Moreover, it has been reported previously that long DNA chains favors the formation of spherical nanoparticles, which is consistent with our results for the λ-phage DNA.33 To compare AFM and DLS data, the diameter of spheres with a volume equal to the volume of particles found on mica was calculated. The relatively small and large particles have the diameter of 30 ± 20 nm and 260 ± 90 nm, respectively. These values are 2–3 times lower than the polyplex sizes measured by DLS. As the polyplex sizes measured by DLS and AFM in liquid phase are in the same range, such inconsistency may be explained by the decreasing of the polyplex volume upon drying on a mica surface.34
In order to explain the appearance of the third peak in the size distribution, the samples with N/P ratio of 1.25 were studied by AFM. The polyplexes obtained at N/P = 1.25 have a more complex structure (Fig. 4B). They consist of a dense core with the diameter of 300–1000 nm, and the height of 15–45 nm. The core is surrounded by a crown comprising of filamentous fragments, with the height of several nanometers, which could be attributed to the not fully complexed DNA chains. The diameter of the equivalent spheres with the same volume as the volume of the polyplexes dense core is 200 ± 60 nm. Thus, at low N/P the complexes lost their compact spherical shape. A new population of particles with sizes of about 20 nm most probably corresponds to DNA strands, partly bound with the copolymer molecules.
Conclusions
A simple synthetic strategy for engineering novel bioactive acrylamide-based cationic polymers with a desired contribution of amine groups was established. This strategy may open up a new way for the construction/formulation of polymer systems for DNA-based applications. It was demonstrated that a functional group approach can be successfully realized in a free-radical copolymerization process for preparing cationic copolymers with the copolymer composition that corresponds to the molar ratio of initial monomers. Besides, it was shown that in the range of 1 ≤ N/P ≤ 10 the cationic acrylamide/3-(N,N-dimethylaminopropyl)-acrylamide copolymer forms stable complexes with DNA; the charge neutralization point was found at N/P = 2.2. The compact positively charged spherical polyplexes formed at the excess of the copolymer have prospects as intelligent nanocarriers for drug and gene delivery.
Acknowledgements
The authors are grateful to Prof. Babushkina T. A., Mrs Klimova T. P. for helpful discussion and NMR spectral data. The authors also acknowledge Mrs Klemenkova Z. S. for IR spectral data. E. Laukhina acknowledges the support from Instituto de Salud Carlos III, through “Acciones CIBER”. The Networking Research Center on Bioengineering, Biomaterials and Nanomedicine (CIBER-BBN), an initiative funded by the VI National R&D&I Plan 2008-2011, Iniciativa Ingenio 2010.
References
- A. Kowalczuk, R. Trzcinska, B. Trzebicka, A. H. E. Müller, A. Dworak and C. B. Tsvetanov, Prog. Polym. Sci., 2014, 39, 43–86 CrossRef CAS.
- I. Tabujew and K. Peneva, in Cationic Polymers in Regenerative Medicine, The Royal Society of Chemistry, Cambridge, 2015, pp. 1–29, 10.1039/9781782620105-00001.
- M. Ahmed and R. Narain, in Cationic Polymers in Regenerative Medicine, The Royal Society of Chemistry, Cambridge, 2015, pp. 62–98, 10.1039/9781782620105-00062.
- E. Bilensoy, G. Isk and C. Varan, in Cationic Polymers in Regenerative Medicine, The Royal Society of Chemistry, Cambridge, 2015, pp. 268–295, 10.1039/9781782620105-00268.
- E. Haladjova, N. Toncheva-Moncheva, M. D. Apostolova, B. Trzebicka, A. Dworak, P. Petrov, I. Dimitrov, S. Rangelov and C. B. Tsvetanov, Biomacromolecules, 2014, 15, 4377–4395 CrossRef CAS PubMed.
- S. Y. Wong, J. M. Pelet and D. Putnam, Prog. Polym. Sci., 2007, 32, 799–837 CrossRef CAS.
- C. Tuncer, E. Canavar, G. Congur, H. Karadeniz, A. Erdem and V. Butun, Talanta, 2012, 100, 270–275 CrossRef CAS PubMed.
- X. Liu, Q. Fan and W. Huang, Biosens. Bioelectron., 2011, 26, 2154–2164 CrossRef CAS PubMed.
- H. Peng, L. Zhang, C. Soeller and J. Travas-Sejdic, Biomaterials, 2009, 30, 2132–2148 CrossRef CAS PubMed.
- W. Jaeger, J. Bohrisch and A. Laschewsky, Prog. Polym. Sci., 2010, 35, 511–577 CrossRef CAS.
- G. Kwon and T. Okano, Pharm. Res., 1999, 16, 597–600 CrossRef CAS.
- M. A. Wolfert, E. H. Schacht, V. Toncheva, K. Ulbrich, O. Nazarova and L. W. Seymour, Hum. Gene Ther., 1996, 7, 2123–2133 CrossRef CAS PubMed.
- F. Delisavva, G. Mountrichas and S. Pispas, J. Phys. Chem. B, 2013, 117, 7790–7796 CrossRef CAS PubMed.
- E. Haladjova, G. Mountrichas, S. Pispas and S. Rangelov, J. Phys. Chem. B, 2016, 120, 2586–2595 CrossRef CAS PubMed.
- T. Krasia-Christoforou and T. K. Georgiou, J. Mater. Chem. B, 2013, 1, 3002–3025 RSC.
- P. Occhetta, C. Malloggi, A. Gazaneo, A. Redaelli, G. Candiani and M. Rasponi, RSC Adv., 2015, 5, 5087–5095 RSC.
- S. Berry, P. Mastorakos, C. Zhang, E. Song, H. Patel, J. S. Suk and J. Hanes, RSC Adv., 2016, 6, 41665–41674 RSC.
- M. A. Mintzer and E. E. Simanek, Chem. Rev., 2009, 109, 259–302 CrossRef CAS PubMed.
- A. V. Slita, N. A. Kasyanenko, O. V. Nazarova, I. I. Gavrilova, E. M. Eropkina, A. K. Sirotkin, T. D. Smirnova, O. I. Kiselev and E. F. Panarin, J. Biotechnol., 2007, 127, 679–693 CrossRef CAS PubMed.
- D. W. Pack, A. S. Hoffman, S. Pun and P. S. Stayton, Nat. Rev. Drug Discovery, 2005, 4, 581–593 CrossRef CAS PubMed.
- M. A. Islam, T. Ä. Park, B. Singh, S. Maharjan, J. Firdous, M.-H. Cho, S.-K. Kang, C.-H. Yun, Y. Ä. Choi and C.-S. Cho, J. Controlled Release, 2014, 193, 74–89 CrossRef CAS PubMed.
- O. Boussif, F. Lezoualch, M. A. Zanta, M. D. Mergny, D. Scherman, B. Demeneix and J. P. Behr, Proc. Natl. Acad. Sci. U. S. A., 1995, 92, 7297–7301 CrossRef CAS.
- M. Byrne, D. Victory, A. Hibbitts, M. Lanigan, A. Heise and S.-A. Cryan, Biomater. Sci., 2013, 1, 1223–1234 RSC.
- A. Namvar, A. Bolhassani, N. Khairkhah and F. Motevalli, Biopolymers, 2015, 103, 363–375 CrossRef CAS PubMed.
- A. V. Ulasov, Y. V. Khramtsov, G. A. Trusov, A. A. Rosenkranz, E. D. Sverdlov and A. S. Sobolev, Mol. Ther., 2011, 19, 103–112 CrossRef CAS PubMed.
- A. C. Rinkenauer, S. Schubert, A. Traeger and U. S. Schubert, J. Mater. Chem. B, 2015, 3, 7477–7493 RSC.
- W. Fischer, B. Brissault, S. Prévost, M. Kopaczynska, I. Andreou, A. Janosch, M. Gradzielski and R. Haag, Macromol. Biosci., 2010, 10, 1073–1083 CrossRef CAS PubMed.
- N. K. Davydova, O. V. Sinitsyna, I. V. Yaminsky, E. V. Kalinina and K. E. Zinoviev, Macromol. Symp., 2012, 321–322, 84–89 CrossRef CAS.
- N. K. Davydova, O. V. Sinitsyna and K. E. Zinoviev, AIP Conf. Proc., 2012, 1459, 220–222 CrossRef CAS.
- I. Y. Perevyazko, M. Bauer, G. M. Pavlov, S. Hoeppener, S. Schubert, D. Fischer and U. S. Schubert, Langmuir, 2012, 28, 16167–16176 CrossRef CAS PubMed.
- Y. Yue, F. Jin, R. Deng, J. Cai, Y. Chen, M. C. M. Lin, H.-F. Kung and C. Wu, J. Controlled Release, 2011, 155, 67–76 CrossRef CAS PubMed.
- M. S. Shim, X. Wang, R. Ragan and Y. J. Kwon, Microsc. Res. Tech., 2010, 73, 845–856 CrossRef CAS PubMed.
- S. Danielsen, G. Maurstad and B. T. Stokke, Biopolymers, 2005, 77, 86–97 CrossRef CAS PubMed.
- C. Troiber, J. C. Kasper, S. Milani, M. Scheible, I. Martin, F. Schaubhut, S. Küchler, J. Rädler, F. C. Simmel, W. Friess and E. Wagner, Eur. J. Pharm. Biopharm., 2013, 84, 255–264 CrossRef CAS PubMed.
Footnote |
† Electronic supplementary information (ESI) available: NMR spectrum of AADMAPA. IR spectrum of AADMAPA. AFM images of DNA phage λ and pure AADMAPA. The dependence of ζ-potential and the hydrodynamic radius of DNA phage λ on its concentration in aqueous solution. Table with DNA amounts and the volume of its water solution added to the water solution of AADMAPA to obtain AADMAPA/DNA complexes at different N/P ratios. Table with ζ-potential, average diameter and volume of polyplexes at different N/P ratios. See DOI: 10.1039/c6ra11231e |
|
This journal is © The Royal Society of Chemistry 2016 |