DOI:
10.1039/C6RA09123G
(Paper)
RSC Adv., 2016,
6, 58203-58211
Electrolytes as a tuning parameter to control nano-emulsion and nanoparticle size†
Received
8th April 2016
, Accepted 9th June 2016
First published on 13th June 2016
Abstract
Currently, polymeric nanoparticles are widely used in a wide variety of biomedical applications, which requires different nanoparticle sizes. Their preparation by solvent evaporation from polymeric nano-emulsions has been described as an appropriate methodology. Nano-emulsions can be prepared by the phase inversion composition method, a low-energy emulsification method, apt for biomedical applications since it can be performed at low conditions. Using this methodology, nano-emulsions droplets and resulting nanoparticle properties depend on the selected components. Many studies have been performed varying some of them. In this work, a specific study has been performed for the first time to find out the effect that the presence of electrolytes in the aqueous phase of the template nano-emulsion has on nano-emulsion and nanoparticle physicochemical properties. PLGA nano-emulsions were prepared using the system: aqueous solution (W)/polysorbate 80 (S)/[4 wt% PLGA in ethyl acetate] (O). The addition of increasing concentrations of electrolytes (PBS) in the aqueous phase produced a decrease on droplet hydrodynamic radii from around 200 nm to around 35 nm. Nanoparticle sizes were determined by the droplet sizes of their template nano-emulsions. Nanoparticles dialysis to an electrolyte solution of 0.16 M, required for many biomedical applications to accomplish the physiological pH and osmolality, did not produce changes in nanoparticle sizes. Therefore, it has been demonstrated for the first time that nano-emulsion and nanoparticle size can be tuned by only varying the electrolyte concentration of the template nano-emulsions. Nano-emulsion templating is a versatile technology that enables the obtaining of tunable nanoparticle sizes; appropriate for a wide range of biomedical applications.
1 Introduction
The interest of polymeric nanoparticles prepared from nano-emulsion templating has experienced an exponential growth in the last few years as it is a versatile technology with potential application in a wide variety of fields from food to biomedical or pharmaceutical.1–3 Nano-emulsions are fine emulsions, i.e. dispersions of two immiscible liquids in which one is dispersed into the other forming small droplets, with sizes typically between 20–500 nm.4–7 Due to their nanometric size, they show transparent to translucent appearance and high kinetic stability against sedimentation or creaming. However, they are destabilized mainly by Ostwald ripening.7–10 As thermodynamically unstable systems, an energy input is required for their formation. The energy input can arise from external sources (high-energy emulsification) or from the internal chemical energy of the system (low-energy emulsification).6 The so-called low-energy emulsification methods are advantageous not only for energy issues, but also to obtain nano-emulsions with minimum droplet size and low polydispersity.6 Among the low-energy emulsification methods, the phase inversion composition (PIC) method is preferred when working with thermolabile compounds (e.g. lipophilic drugs such as dexamethasone or biomolecules), since it can be performed under mild temperature conditions (e.g. 25 °C).2,6 Moreover, it is a highly versatile technology that can be scaled-up for a large-scale production. Polymeric nanoparticles are usually obtained by solvent evaporation from template nano-emulsions.11,12 Therefore, nanoparticles with the desired characteristics can be obtained with an appropriate selection of nano-emulsion components and composition.3,6 Although it has been showed that changes on some components of O/W nano-emulsions produced changes in the nano-emulsion characteristics, namely on droplet size and stability,1,5 to our best knowledge, the effects of electrolyte concentration on nano-emulsion droplet size and consequently on nanoparticle size has not been studied systematically. In our previous studies, it was found that the use of a phosphate buffered saline (PBS) solution instead of water as the aqueous continuous phase markedly influenced nano-emulsion droplet size, which decreased almost one order of magnitude when the PBS solution was used.13,14 Therefore, it was found of interest to study more systematically the effect of electrolyte concentration on nano-emulsion and nanoparticle size. Other objectives of this work were to obtain nanoparticles apt for a wide variety of applications by tuning their characteristics, namely size, by means of changes in the template nano-emulsion aqueous phase composition and to find out if the properties of nanoparticles, once they have been formed, are influenced by the concentration of electrolytes of the dispersion medium.
2 Experimental section
2.1. Materials
Poly(lactic-co-glycolic acid), Resomer 752H in the following abbreviated as PLGA (polystyrene equivalent molecular weight PSE – MW ∼10
000 g mol−1, as determined by Gel Permeation Chromatography) was purchased from Boehringer Ingelheim. The lactic acid to glycolic acid ratio of the PLGA used was 75/25 and the end groups were free carboxylic acids. Ethyl acetate used as the organic volatile solvent was purchased from Merck. Polysorbate 80 is a nonionic surfactant kindly provided by Croda. It is a yellow liquid at room temperature and has an HLB value of 15.15 Salts, used for the electrolyte solution (pH = 7.4) preparation (sodium chloride 8 g L−1, sodium dihydrogen phosphate monohydrate 0.219 g L−1 and disodium monohydrogen phosphate dihydrate 2.983 g L−1) were purchased from Merck. Water was MilliQ filtered.
2.2. Methods
2.2.1. Nano-emulsion and nanoparticle formation. Polymeric O/W nano-emulsions were prepared using the phase inversion composition method (PIC).6 A total of 4 g of each sample was prepared. A mixture of oil and surfactant was mixed and the aqueous phase was stepwise added (at different electrolyte concentrations). The region of O/W nano-emulsion formation was assessed visually, in the pseudoternary water/surfactant/oil diagram, observing the macroscopic appearance of the samples under a spotlight. Samples with transparent, translucent or slightly opaque aspect, having a bluish or reddish shine were considered nano-emulsions.The ethyl acetate was evaporated from the nano-emulsion droplets under reduced pressure (43 mbar, 45 min, 25 °C) to enable the formation of polymeric nanoparticles using the solvent evaporation method.16
2.2.2. Phase inversion assessment. Two methodologies were used to assess phase inversion, at 25 °C. For those samples prepared without electrolytes, the conductivity was measured using a Conductimeter Crison model 525, with a Pt/platinized electrode. For those samples with electrolytes, colorimetric assays were carried out, as inversion is not detected by conductivity. A hydrophilic dye (Rhodamine B) and a lipophilic dye (Sudan Black) were used.
2.2.3. Nano-emulsion droplet and nanoparticles size determination by light scattering. The mean nano-emulsion droplet and nanoparticle size were determined by dynamic light scattering (DLS), using a spectrometer (LS Instruments, 3D cross correlation multi-scattering) equipped with a He–Ne laser (632.8 nm) with variable intensity. Measurements were carried out at a scattering angle of 90°, and at a constant temperature of 25 °C in triplicates, without diluting the samples. Data were treated by cumulant analysis.17 The characterization was performed by the Nanostructured Liquid Characterization Unit of the Spanish National Research Council (CSIC) and the Biomedical Networking Center (CIBER-BBN), located at IQAC-CSIC.
2.2.4. Surface charge determination. The surface charge of nano-emulsions and nanoparticles was assessed by electrophoretic mobility measurements with Zetasizer NanoZS instrument (Malvern Co. Ltd., UK), equipped with a He–Ne red light laser (λ = 633 nm). This instrument measures the velocity of the droplets or particles in the electric field (electrophoretic mobility) using the laser Doppler electrophoresis method.18,19 The zeta potential (ζ) was calculated from the electrophoretic mobility applying the Hückel–Onsager equation (eqn (1); modified from the Smoluchowsky equation20–22): |
 | (1) |
where εr represents the relative dielectric constant of water, ε0 is the vacuum permittivity and η is the viscosity of the liquid. As this equation is only valid if the mobility is low, samples were diluted 1
:
20 with distilled water.
2.2.5. Stability assessment. Nano-emulsion and nanoparticle stability was assessed by two different methodologies, at 25 °C. Their macroscopic visual appearance was observed, under a spotlight, as a function of time. Dispersions were defined as stable until a sediment appeared. Droplet/nanoparticle sizes were also measured as a function of time. Size measurements were carried out as described in Section 2.2.3.
2.2.6. Nanoparticles transference to PBS. The dialysis bag method was used, as described elsewhere,13 but in this case, to change nanoparticles dispersion media to the PBS buffer (0.16 M electrolyte solution), at a pH = 7.4. Thermojacketted glasses were used to maintain the desired temperature (25 °C). They were filled with 50 mL of PBS. Spectra/Por® Float-A-Lyzer® G2 (Spectrum®Labs) devices with a membrane composed of Biotech Grade Cellulose Ester with a MWCO of 3.5–5 kDa, filled with 2 mL of sample and soaked into the PBS receptor solution. After 24 h, dialysis was stopped and nanoparticles were characterized to determine size and surface charge.
3 Results and discussion
3.1. Influence of electrolytes on nano-emulsion formation and properties
Nano-emulsions were prepared by the PIC method (i.e. slow addition of the aqueous phase to oil/surfactant mixtures), at 25 °C. The system electrolyte solution (W)/polysorbate 80 surfactant (S)/[4 wt% PLGA in ethyl acetate] (O) was chosen for this study.
The influence of electrolytes on the nano-emulsion domain was determined for systems with electrolyte aqueous solutions of 0.03 M and 0.08 M. Fig. 1 shows the results obtained and, for comparative purposes, the nano-emulsion domains for the systems without electrolytes (0 M) and with a 0.16 M electrolyte solution are also included.13,14 The upper limit considered (0.16 M electrolyte) was chosen based on a previous study14 as it accomplishes the physiological blood conditions (pH = 7.4 and 300 mOsm kg−1), required to administer nanosystems by the parenteral route.23 Fig. 1 shows that the nano-emulsion domain is small for nano-emulsions without electrolytes, comprising O/S ratios between 40/60 and 70/30 and water contents above 85 wt%. In the presence of electrolytes, nano-emulsion domains are considerably bigger (broader O/S ratios and water contents). The dependency of nano-emulsion formation region on the electrolyte concentration was not linear, since at the highest electrolyte concentration (0.16 M), nano-emulsion domain is smaller than that at 0.08 M (Fig. 1).
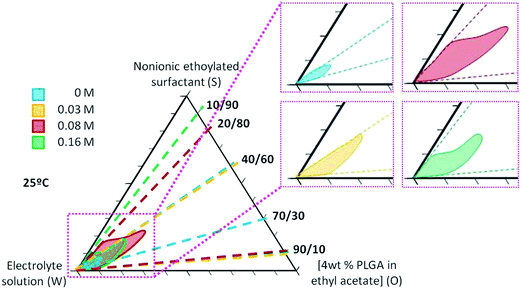 |
| Fig. 1 Region of nano-emulsion formation in the system electrolyte solution with different molarities (W)/nonionic ethoxylated surfactant (S)/[4 wt% PLGA in ethyl acetate] (O). Magnification of the nano-emulsion domains is shown on the upper right of the figure (pink square). | |
To confirm that phase inversion took place during emulsification, conductivity and dye diffusion experiments were performed (Fig. S1 and S2, ESI†). Inversion from W/O to O/W structures was confirmed by both techniques. It should be noted that conductivity could only be used to detect phase inversion in the system without electrolytes.
The visual appearance of nano-emulsions (transparency and shining) was dependent on both, the composition of the nano-emulsion and the electrolyte concentration. Nano-emulsions with 90 wt% water showed the most transparent appearance (as compared with those with lower water content) (Fig. S3†) and they were chosen for further studies. The effect of electrolyte concentration on the macroscopic appearance was evaluated in nano-emulsions with 90 wt% aqueous solution and O/S of 70/30. This O/S ratio was selected on the basis of previous studies as a compromise of low surfactant concentration and small droplet size.13,14 The visual aspect of the nano-emulsions is shown in Fig. 2. With no electrolytes, nano-emulsion shining tended to reddish, with a translucent appearance, while in the presence of electrolytes nano-emulsions displayed a bluish transparent shine, independently on the electrolyte concentration. However, they showed increasing transparency with the increase of electrolyte concentration. These differences on the macroscopic appearance of nano-emulsions are attributed to differences in droplet sizes3,24 and/or differences between the refractive indexes of the aqueous and the oil phase.25,26 In this work, the first hypothesis, differences in droplet size, is more likely, since the oil component was the same (ethyl acetate with 4 wt% PLGA) for all nano-emulsions and the refractive index of the aqueous phase only experienced a slight increase when electrolytes were incorporated (Table S1†).
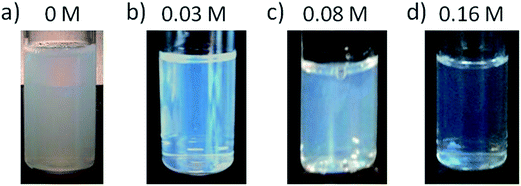 |
| Fig. 2 Nano-emulsions with 90 wt% aqueous phase and an O/S ratio of 70/30. The electrolyte concentration in the aqueous phase is: (a) 0 M; (b) 0.03 M; (c) 0.08 M and (d) 0.16 M. | |
Visual observation of the nano-emulsions as a function of time showed that no phase separation was produced for a period of 60 days. However, a sediment (a white powder) appeared with time. The sediment was attributed to an early formation of nanoparticles. Since ethyl acetate is a volatile organic solvent partially water soluble, solvent diffusion to the aqueous phase as well as solvent evaporation might occur promoting an early formation of nanoparticles, which sediment due to their higher density. The time taken to observe sedimentation is shown in Table 1. For nano-emulsions formulated with water, the lower the O/S ratio, the higher the time taken to observe sedimentation which could be attributed to the surfactant stabilizing effect. The presence of electrolytes produced a drastic increase of the time to observe sedimentation, especially at intermediate O/S ratios. The considerable increase in the time to observe sedimentation in the presence of electrolytes could be attributed to the smaller size of the droplets (inferred from the transparency and confirmed from the data in Section 3.2).1
Table 1 Time taken to observe sedimentation, at 25 °C, of samples with 90% water or aqueous solution, as a function of O/S ratio and electrolyte concentration
O/S |
Time taken to observe a sediment (days) |
Water |
Electrolyte solution |
0.032 M |
0.08 M |
0.16 M |
10/90 |
— |
— |
— |
0.5 |
20/80 |
— |
— |
1 |
0.5 |
30/70 |
— |
— |
31.5 |
0.5 |
40/60 |
— |
2 |
>60 |
16.5 |
50/50 |
4 |
31.5 |
>60 |
16.5 |
55/45 |
1.5 |
42 |
>60 |
16.5 |
60/40 |
0.5 |
>60 |
>60 |
16.5 |
65/35 |
0.5 |
>60 |
>60 |
16.5 |
70/30 |
0.5 |
>60 |
>60 |
16.5 |
80/20 |
— |
>60 |
11 |
32 |
85/15 |
— |
31.5 |
11 |
22.5 |
90/10 |
— |
2 |
11 |
11.5 |
In spite of the high variation in the time to observe sedimentation depending on electrolyte concentration and O/S ratio, it should be noted that all nano-emulsions showed enough stability to be used as nanoparticle templates, a process that takes around 1 hour.
3.2. Influence of electrolytes on nano-emulsion droplet and nanoparticle size
The effect of electrolytes on nano-emulsion droplet size was systematically studied for nano-emulsions with 90 wt% aqueous phase with 0, 0.016, 0.032, 0.04, 0.05, 0.08, 0.1, 0.132 and 0.16 M electrolyte concentrations and various O/S ratios. These nano-emulsions were used to obtain nanoparticles by solvent evaporation (as described in Section 2.2.1) and nanoparticle size was also evaluated. The results are shown in Fig. 3.
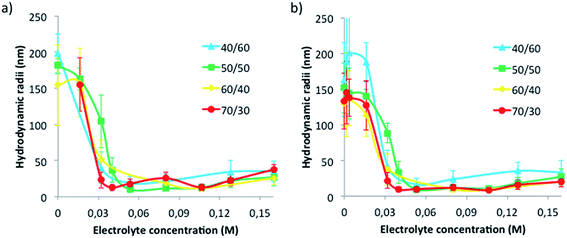 |
| Fig. 3 Hydrodynamic radius (in nm) as a function of electrolyte concentration (in M) of (a) nano-emulsions prepared with 90 wt% of aqueous phase and different O/S ratios and (b) nanoparticles prepared from these template nano-emulsions. | |
Fig. 3a shows nano-emulsion hydrodynamic droplet radii as a function of electrolyte concentration. With no electrolytes, nano-emulsion droplet radii are in the range 150–200 nm. The addition of electrolytes produced a marked size decrease, of nearby one order of magnitude, independently on the O/S ratio, resulting in sizes of around 20 nm at an electrolyte concentration of around 0.04 M. Above 0.04 M electrolyte concentration and up to the highest electrolyte concentration tested (0.16 M), hydrodynamic sizes were fairly constant and did not exceed 30 nm (Fig. 3a). The size decrease as a consequence of the electrolytes can be attributed to several factors. One is the solvent diffusion effect.27,28 As mentioned above, since ethyl acetate is a partially water-soluble volatile solvent, it can diffuse from the dispersed to the continuous phase, thus producing a decrease in the droplet size. This effect is enhanced when electrolytes are present, due to an increase of the osmotic pressure,6 which is directly proportional to the electrolyte concentration (see Explanation ES1†). The higher osmotic gradient enhances the diffusion of ethyl acetate to the continuous phase. Therefore, the higher the electrolyte concentration, the higher the osmotic gradient and, as a result, a higher reduction of droplet size should be expected. Another effect of the presence of electrolytes to be taken into account is the retardation of Oswald ripening since the higher the electrolyte concentration the lower the solubility of ethyl acetate in the aqueous phase (see data from Table S2†). This effect could also produce smaller droplet sizes. Another factor induced by the presence of electrolytes is the reduction of the oil/water interfacial tension. It has been reported in previous studies,29,30 that the addition of electrolytes to water/surfactant/oil systems produces a decrease on the oil/water interfacial tension, which favors the formation of smaller droplet sizes. Therefore, all these effects, osmotic gradient, decrease in ethyl acetate solubility and reduction in the oil/water interfacial tension, may contribute to the size reduction. It is worth noting that the reduction of droplet size (Fig. 3) takes only place up to an electrolyte concentration of 0.04 M. Above this concentration, the aqueous phase is probably saturated of ethyl acetate and no further diffusion takes place with addition of more electrolytes. Consequently, at high electrolyte concentrations changes on droplet size are not produced.
Concerning the size dependence on the O/S ratio, an increase in droplet size with the increase of O/S ratio has been reported for nano-emulsions with non-polar oils, attributed to the reduction of surfactant molecules stabilizing the droplets.6 However, in systems with polar oils, as that reported in the present study, a different tendency is observed.3 Fig. 3 shows a size decrease when increasing the O/S ratio, which is more noticeable at low electrolyte concentrations (Fig. S4†). This effect was attributed to the combination of two factors: the decrease in the surfactant concentration when increasing the O/S ratio and the above-mentioned solvent diffusion effect. It is remarkable the small nanometric droplet sizes obtained, as compared with previous bibliography,1,31–33 which are desired, since they are expected to be less affected by migrational destabilization processes.6,10 Only few studies were found in the literature reporting so small droplet sizes.3,4,6,34 The small nanometric droplet sizes are attributed to the use of the PIC nano-emulsification method, as previously described.1,2
As expected, the effect of electrolytes on nanoparticle size followed the same tendency as that on the template nano-emulsions (Fig. 3b). Nanoparticle sizes, at null and low electrolyte concentrations, were high (around 140 nm), regardless of the O/S ratio of the template nano-emulsion. The increase in electrolyte concentration from 0.02 M to 0.04 M produced a marked nanoparticle size decrease down to radii around 20 nm. Further electrolyte concentration increase produced only slight size increase.
3.3. Influence of electrolytes on nano-emulsions and nanoparticles surface charge
The influence of electrolytes on nano-emulsion and nanoparticles surface charge was assessed by means of ζ potential calculation from electrophoretic mobility determinations, as described in the experimental part (Section 2.2.4). Surface charges (Fig. 4) were negative, due to the carboxylic groups of the PLGA polymer, irrespective of the electrolyte concentration. These results agree with other studies reporting the production of PLGA nanoparticles, in which negative ζ potentials were also found.35–37
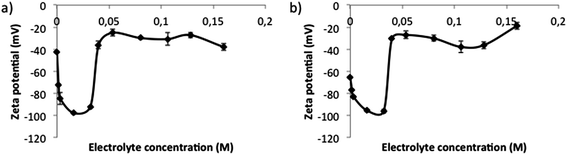 |
| Fig. 4 Zeta potential as a function of electrolyte concentrations of: (a) nano-emulsions with 90 wt% of aqueous phase and 50/50 O/S ratio and (b) nanoparticles prepared from these template nano-emulsions. | |
Surface charge (Fig. 4) suffered a marked decrease (down to −100 mV) when electrolyte concentrations were low (up to around 0.04 M). However, above 0.04 M, an abrupt increase on the surface charge (up to −20 mV) is produced and above 0.05 M surface charge remained practically constant.
A continuous decrease in the surface charge (increase on the absolute value) with the increase in electrolyte concentration was expected, due to the presence of ionic species. However, it was not found. This could be due to the compensation between negative and positive charges of the salts, whose contribution is counteracted not only by the charges of the solution but also by the PLGA charges. Moreover, some authors have reported that the ζ potential is dependent on the pH of the solvent: the lower the pH, the higher the ζ potential absolute value.23 This is in good agreement with our results (Fig. 4): at 0 M electrolyte concentration (pH around 5.5), the ζ potential is higher in absolute value than that at 0.04–0.16 M electrolyte concentration (pH around 7.4).
3.4. Influence of electrolytes on nano-emulsion and nanoparticle stability
The hydrodynamic radii of nano-emulsions without electrolytes and 50/50 O/S ratio (Fig. 5a) showed fluctuations for the first two days but remained practically constant up to 6 days; and after this time, the size decreased progressively, in good agreement with visual observations. This size reduction is also concomitant with the appearance of a sediment, which, as described above, is attributed to an early formation of nanoparticles. Since the sedimentation is a phenomenon that acts proportionally to the mass of the molecules, the bigger nanoparticles will be those that sediment first, thus resulting in a decrease on the mean nano-emulsion droplet size. The initial size fluctuations could be caused by the combination of Ostwald ripening/coalescence and solvent diffusion effects. The addition of long-chain hydrocarbons (squalane and hexadecane) to reduce Ostwald ripening6,10 did not affect the fluctuations in size at short times. Nevertheless, after the initial size variations, nano-emulsion droplet size was constant for longer times in the presence of squalene or hexadecane (Fig. S5†).
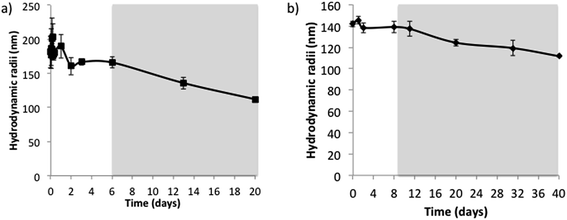 |
| Fig. 5 Hydrodynamic radii as a function of time of: (a) a nano-emulsion with 90 wt% of water (0 M) and an O/S ratio of 50/50; and (b) nanoparticles from this template nano-emulsion. Grey zone indicates the presence of a sediment in the samples. | |
The nanoparticle dispersion prepared from the nano-emulsion without electrolytes was stable for around 12 days (Fig. 5b), and after this time, it experienced sedimentation, which would explain the slight decrease in size with time.
The presence of electrolyte, in the concentration range studied, resulted in an improvement of stability for both nano-emulsions and nanoparticles. For nano-emulsions formulated with 0.16 M electrolyte concentration, at 50/50 and 70/30 O/S ratios, after a slight size decrease, droplet sizes kept fairly constant, at around 30 nm and 20 nm, respectively up to 40 days (Fig. 6a). These results are in good agreement with previous studies, which reported an increased stability of diverse types of emulsions when they were formulated containing salts in the aqueous phase.38–40 Kunieda et al.,38 for example, attributed this stability enhancement to a higher salting out effect, leading to the dehydration of the surfactant polar heads, due to a higher affinity of water molecules for the electrolyte than for the surfactant molecules, which, in turn, produce a more compact interfacial film caused by an increase in the polar heads lateral interactions, which ends up with a reduction in diffusion of the solvent. Jiang et al.,40 in contrast, attributed the enhanced stability to interactions between the surfactant and the electrolytes, increasing the interfacial tension and enhancing droplet stability. In addition, the decrease in the stability when the electrolyte concentration was further increased (to 0.16 M in our study) was also reported in previous bibliography.15,40 Stolnik et al.,15 found nanoparticle aggregation when they added high salt concentration in the aqueous phase, which was attributed to a deionization of the surface chemical groups, such as carboxylic groups of the PLGA polymer. Rao et al.39 attributed this stability decrease to a colloid aggregation due to an electrostatic attraction of the negative charges of the droplets with electrolytes at high salt concentrations, enhancing flocculation or aggregation.
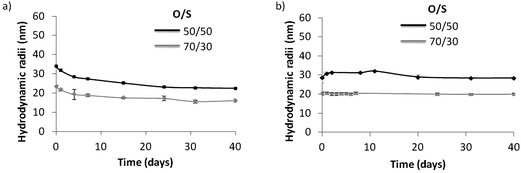 |
| Fig. 6 Hydrodynamic radii as a function of time at different O/S ratios of (a) nano-emulsions with aqueous solution (0.16 M); and (b) nanoparticles obtained from these template nano-emulsions. | |
Concerning the stability of nanoparticles obtained from the nano-emulsions with electrolytes, the size, similar to that of the template nano-emulsions, was practically unchanged for at least 40 days (Fig. 6b).
Summarizing, comparing the change of the initial sizes of nano-emulsions with their resulting nanoparticles, at 50/50 O/S ratios (Fig. 3 and S4†), it can be appreciated that, while a marked size decrease takes places for those systems formulated with water, size is almost constant when electrolytes are incorporated to nano-emulsions, which is consistent with the hypothesis of the solvent diffusion. When electrolytes are incorporated to the nano-emulsion aqueous phase, the ethyl acetate tends to diffuse to the continuous phase to equilibrate the higher gradient of osmotic pressure, as compared to water system. Therefore, at 0.16 M electrolyte concentration, when nanoparticles are formed, the amount of solvent that remained and can be evaporated from nano-emulsions is smaller than that from nano-emulsions with water, whose size is more reduced when forming nanoparticles.
3.5. Versatility of nano-emulsion templating to obtain nanoparticles that accomplish size and physiological requirements for parenteral administration
Currently, a vast number of polymeric nanoparticle studies are devoted to biomedical applications.1,2,12,14,31 In most of these studies, nanoparticles are intended for their administration by the parenteral route. Therefore, they should accomplish the physiological blood conditions (pH = 7.4 and osmolality = 300 mOsm kg−1) to be compatible with biological tissues.23 The aim of this part of the study was to achieve a nanoparticle production platform that enables obtaining nanoparticles with tuned sizes accomplishing physiological pH and osmolality conditions for parenteral administration. With this purpose, nanoparticles using template nano-emulsions with different electrolyte concentrations (i.e. different controlled size) were prepared and then the effect of changing the nanoparticle dispersant medium to 0.16 M electrolyte concentration, which fulfills physiological pH and osmolality, was studied.
The sizes and surface charge of nanoparticles obtained from nano-emulsions at four initial electrolyte concentrations (0 M, 0.03 M, 0.08 M and 0.16 M) were measured after changing the nanoparticle dispersant medium to 0.16 M electrolyte concentration by dialysis (Table 2). For comparison purposes, the results before dialysis are also shown.
Table 2 Hydrodynamic radius (nm) and surface charge (mV) of nanoparticles, prepared from nano-emulsions with 70/30 O/S ratio and 90 wt% of aqueous phase content at different electrolyte concentrations, before and after dialysis with 0.16 M electrolyte concentration
Initial dispersant |
Hydrodynamic radius (nm) |
ζ potential (mV) |
Before dialysis |
After dialysis (0.16 M PBS) |
Before dialysis |
After dialysis (0.16 M PBS) |
Water |
139 ± 4 |
134 ± 0.4 |
−62 ± 3 |
−69 ± 2 |
PBS 0.03 M |
20 ± 0.1 |
17 ± 0.1 |
−26 ± 0.6 |
−33 ± 3 |
PBS 0.08 M |
12 ± 5 |
11 ± 0.5 |
−24 ± 0.9 |
−31 ± 2 |
PBS 0.16 M |
20 ± 3 |
24 ± 0.7 |
−19 ± 0.7 |
−19 ± 1 |
Table 2 shows that no significant variations are produced on the hydrodynamic radii of nanoparticles before and after the dialysis. Therefore, these results clearly show that once nanoparticle sizes have been tailored by using the appropriate electrolyte concentration in the template nano-emulsion, they maintain their size when transferred to a physiological solution that has the appropriate pH and osmolality for parenteral administration.
The fact that nanoparticle sizes are maintained after their transfer to another dispersant medium was expected, as no solvent remained in the nanoparticles as determined by head-space gas chromatography in a previous study.41 This proof is important in terms of formulation safety.23 In addition, these results support the assumption that the effect of the size reduction observed in Fig. 5 is not caused by solvent diffusion to the dispersant medium.
Concerning surface charge (Table 2), it increased in absolute value when the dispersant medium was changed to 0.16 M electrolyte solution, thus tending to more negative surface charges except, as expected, for those already dispersed in 0.16 M electrolyte solution. These results confirmed that dialysis took place, achieving a change of the dispersant medium to the appropriate electrolyte concentration (PBS 0.16 M), since the surface charge depends on the electrolytes present in the sample. It is worth noting that these results seem controversial with Fig. 4, where surface charge decreased in absolute value when increasing the electrolyte concentration. Nevertheless, although all nanoparticles are suspended in 0.16 M electrolyte concentration, an effect of the species that form nanoparticles, mainly PLGA, could be hypothesized, which contribute to the surface charge. Since nanoparticles are different in size, the charges of PLGA could be differentially exposed to the dispersant medium, thus contributing to the different surface charges.
Summarizing this last study, the nanoparticle size was a property defined by the template nano-emulsion droplet size, with slightly reduced size due to the solvent evaporation. Once nanoparticles are formed, they maintain their sizes even if electrolyte concentration is changed. The surface charge, in contrast, depends on the dispersant medium, as the surface charge depends on the interactions that surface molecules have with ions of the dispersant medium. Therefore, the increase on the surface charge when changing the nanoparticle dispersant medium is produced by the presence of more electrolytes, which increase the absolute value of the surface charge (Table 2).
4 Conclusions
Polymeric nano-emulsions with a controlled and wide range of droplet sizes have been obtained by the PIC method, in the presence of different concentration of electrolytes. The presence of electrolytes in the aqueous phase produced a marked decrease of droplet sizes, which was attributed mainly to a diffusion of the solvent to the aqueous phase, enhanced by the increased osmotic gradient produced by the increase of electrolyte concentration. Nano-emulsions with the smallest droplet size were highly stable. Regardless of the electrolyte concentration, all the nano-emulsions were stable to be used as nanoparticle templates by the solvent evaporation method. Nanoparticle size can be finely tuned in a wide range of sizes (from around 20 nm to around 150 nm), by controlling the size of their template nano-emulsions by means of adding electrolytes. The change to the dispersant media of nanoparticles to a physiological solution with the appropriate pH and osmolality did not significantly vary nanoparticle properties. Therefore, the nano-emulsion templating approach is a very versatile and promising methodology to prepare tunable nanoparticles with the desired sizes, and physiological dispersant characteristics appropriate for the intravenous administration. By the use of this technology, it is possible to design polymeric nanoparticles appropriate for biomedical applications with the desired sizes by means of selecting the appropriate electrolyte concentration in the aqueous phase of the template nano-emulsion.
Acknowledgements
Financial support from MINECO (grants CTQ2011-29336-CO3-O1); Generalitat de Catalunya (grant 2009-SGR-961), CIBER-BBN are acknowledged. CIBER-BBN is an initiative funded by the VI National R&D&I Plan 2008–2011, IniciativaIngenio 2010, Consolider Program, CIBER Actions and financed by the Instituto de Salud Carlos III with assistance from the European Regional Development Fund. Cristina Fornaguera is grateful to AGAUR for their Predoctoral Fellowship (grant FI-DGR 2012). Authors acknowledge Alba Ortega for her kind support in some experimental part. Authors also acknowledge Dr Carlos Rodríguez-Abreu for the revision of the manuscript.
References
- C. Vauthier and K. Bouchemal, Pharm. Res., 2009, 26(5), 1025–1058, DOI:10.1007/s11095-008-9800-3.
- N. Anton, J. P. Benoit and P. Saulnier, J. Controlled Release, 2008, 128, 185–199, DOI:10.1016/j.jconrel.2008.02.007.
- G. Calderó, M. J. García-Celma and C. Solans, J. Colloid Interface Sci., 2011, 353, 406–411, DOI:10.1016/j.jcis.2010.09.073.
- H. Nakajima, in Industrial Applications of Microemulsions, ed. C. Solans and H. Kunieda, Marcel Dekker, New York, 1997 Search PubMed.
- K. Bouchemal, S. Briançon, E. Perrier and H. Fessi, Int. J. Pharm., 2004, 280(1–2), 241–251, DOI:10.1016/j.ijpharm.2004.05.016.
- C. Solans and I. Solè, Curr. Opin. Colloid Interface Sci., 2012, 17, 246–254, DOI:10.1016/j.cocis.2012.07.003.
- I. Lifshitz and V. Slyozov, J. Phys. Chem. Solids, 1961, 19, 35–50, DOI:10.1016/0022-3697(61)90054-3.
- P. Taylor, Adv. Colloid Interface Sci., 1998, 75, 107–163, DOI:10.1016/S0001-8686(98)00035-9.
- C. Wagner, Theorie der Alterung von Niederschlägen durch Umlesen (Ostwald-Reifung), Zeitschrift für elektrochemie, Berichte der Bunsengesellschaft für physikalische Chemies, 1961, vol. 65, pp. 581–591 Search PubMed.
- T. F. Tadros, P. Izquierdo, J. Esquena and C. Solans, Adv. Colloid Interface Sci., 2004, 108–109, 303–318, DOI:10.1016/j.cis.2003.10.023.
- N. Anton and T. F. Vandamme, Int. J. Pharm., 2009, 377, 142–147, DOI:10.1016/j.ijpharm.2009.05.014.
- C. Fornaguera, A. Dols-Perez, G. Calderó, M. J. García-Celma, J. Camarasa and C. Solans, J. Controlled Release, 2015, 211, 134–143, DOI:10.1016/j.jconrel.2015.06.002.
- C. Fornaguera, M. Llinàs, C. Solans and G. Calderó, Colloids Surf., B, 2015, 125(1), 58–64, DOI:10.1016/j.colsurfb.2014.11.006.
- C. Fornaguera, S. Grijalvo, M. Galán, E. Fuentes-Paniagua, F. J. de la Mata, R. Gómez, R. Eritja, G. Calderó and C. Solans, Int. J. Pharm., 2015, 478(1), 113–123, DOI:10.1016/j.ijpharm.2014.11.031.
- S. Stolnik, S. E. Dunn, M. C. Garnett, M. C. Davies, A. G. A. Coombes, D. C. Taylor, M. P. Irving, S. C. Prkiss, T. F. Tadros, S. S. Davis and L. Illum, Pharm. Res., 1994, 11(12), 1800–1808 CrossRef CAS.
- S. Desgouilles, C. Vauthier, D. Bazile, J. Vacus, J. L. Grossiord, M. Veillard and P. Couvreur, Langmuir, 2003, 19(22), 9504, DOI:10.1021/la034999q.
- W. Brown, Dynamic Light Scattering: The Method and Some Applications, Oxford University Press, New York, 1993 Search PubMed.
- H. A. Lieberman, M. M. Rieger and G. S. Banker, Pharmaceutical Dosage Forms: Disperse Systems Marcel Dekker, New York, 1988 Search PubMed.
- R. J. Hunter, Zeta potential in colloidal Science, Principles and Applications; Colloidal Science, A series of Monographs, Academic Press Inc, San Diego, 1988 Search PubMed.
- R. W. O'Brien and L. R. White, Faraday Trans., 1988, 2, 1607–1626, 10.1039/F29787401607.
- H. Ohshima and K. Makino, Colloids Surf., A, 1996, 109, 71–75, DOI:10.1016/0927-7757(95)03452-8.
- A. V. Delgado, F. González-Caballero, R. J. Hunter and L. K. Koopal, J. Colloid Interface Sci., 2007, 309, 194–224, DOI:10.1016/j.jcis.2006.12.075.
- M. A. Dobrovolskaia and S. E. McNeil, Handbook of Immunological Properties of Engineered Nanomaterials Frontiers in Nanobiomedical Research, ISBN: 978-981-4390-25-5, 2013, vol. 1 Search PubMed.
- L. Spernath, O. Regev, Y. Levi-Kalisman and S. Magdassi, Colloids Surf., A, 2009, 331(1), 19–25, DOI:10.1016/j.colsurfa.2008.08.026.
- P. Schalbart, M. Kawajia and K. Fumoto, Int. J. Refrig., 2010, 33(8), 1612–1624, DOI:10.1016/j.ijrefrig.2010.09.002.
- P. Heunemann, S. Prévost, I. Grillo, C. M. Marino, J. Meyer and M. Gradzielski, Soft Matter, 2011, 7, 5697–5710, DOI:0.1039/c0sm01556c.
- H. Fessi, F. Puisieux, J. P. Devissaguet, N. Ammoury and S. Benita, Int. J. Pharm., 1989, 55, 1–4, DOI:10.1016/0378-5173(89)90281-0.
- J. C. López-Montilla, P. E. Herrera-Morales, S. Pandey and D. O. Shah, J. Dispersion Sci. Technol., 2002, 23(1–3), 219–268, DOI:10.1080/01932690208984202.
- B. P. Binks, W. G. Cho, P. D. I. Fletcher and D. N. Petsev, Langmuir, 2000, 16, 1025–1034 CrossRef CAS.
- P. Dayal, V. Pillay, R. J. Babu and M. Singh, AAPS PharmSciTech, 2005, 6(4), E573–E585 CrossRef PubMed.
- J. Kreuter, J. Anat., 1996, 189(3), 503–505 CAS.
- M. L. Hans and A. L. Lowman, Curr. Opin. Solid State Mater. Sci., 2002, 6(4), 319–327, DOI:10.1016/s1359-0286(02)00117-1.
- P. Couvreur and C. Vauthier, Pharm. Res., 2006, 23(7), 1417–1450 CrossRef CAS PubMed.
- O. Sonneville-Aubrun, D. Babayan, D. Bordeaux, P. Lindner, G. Rata and B. Cabane, Phys. Chem. Chem. Phys., 2009, 11, 101–110, 10.1039/b813502a.
- S. Stolnik, M. C. Garnett, L. Illum, M. Bousta, M. Vert and S. S. Davis, Colloids Surf., A, 1995, 97, 235–245 CrossRef CAS.
- O. Harush-Frenkel, N. Debotton, S. Benita and Y. Altschuler, Biochem. Biophys. Res. Commun., 2007, 353, 26–32 CrossRef CAS PubMed.
- R. P. Singh and P. Ramarao, Toxicol. Sci., 2013, 136(1), 131–143, DOI:10.1093/toxsci/kft179.
- H. Kunieda, N. Yano and C. Solans, Colloids Surf., 1989, 36(3), 313–322, DOI:10.1016/0166-6622(89)80246-x.
- J. Rao and D. J. McClements, Food Hydrocolloids, 2011, 25(6), 1413–1423, DOI:10.1016/j.foodhyd.2011.02.004.
- J. Jiang, Z. Mei, J. Xua and D. Sun, Colloids Surf., A, 2013, 429, 82–90, DOI:10.1016/j.colsurfa.2013.03.039.
- G. Calderó, R. Montes, M. Llinàs, M. J. García-Celma, M. Porras and C. Solans, Studies on the formation of polymeric nano-emulsions obtained via low-energy emulsification and their use as templates for drug delivery nanoparticle dispersions, Colloids Surf., B, DOI:10.1016/j.colsurfb.2016.06.013.
Footnote |
† Electronic supplementary information (ESI) available. See DOI: 10.1039/c6ra09123g |
|
This journal is © The Royal Society of Chemistry 2016 |