DOI:
10.1039/C4TX00090K
(Paper)
Toxicol. Res., 2015,
4, 132-142
Palm oil tocotrienol-rich fraction attenuates testicular toxicity induced by fenitrothion via an oxidative stress mechanism
Received
11th August 2014
, Accepted 23rd September 2014
First published on 24th September 2014
Abstract
Palm oil tocotrienol-rich fraction (TRF) is a well known antioxidant that has shown potential in reducing oxidative stress under various pathological conditions. Exposure to organophosphates such as fenitrothion (FNT) has been reported to cause testicular oxidative damage. The present study was conducted to determine whether TRF could prevent this testicular damage. Parameters evaluated included the oxidative stress status, heat shock protein-70 (HSP70) expression, germ cell apoptosis, reproductive biochemical levels, and morphological alteration. Mature male Sprague-Dawley rats (n = 40) were given FNT (20 mg kg−1), TRF (200 mg kg−1) and TRF + FNT daily via gavage for 28 consecutive days. Co-administration of TRF in FNT-treated rats increased the activities of enzymatic antioxidants such as superoxide dismutase, catalase, and glutathione s-transferase (p < 0.05). TRF also increased the ferric reducing antioxidant power (FRAP) as well as the levels of non-enzymatic antioxidants such as glutathione, compared with the FNT group alone (p < 0.05). Further, TRF reduced lipid peroxidation and protein oxidation by significantly lowering the malondialdehyde and protein carbonyl levels in FNT-treated rats (p < 0.01). HSP70 expression and apoptotic germ cell count in the testis were significantly reduced in the TRF + FNT group (p < 0.05). TRF also ameliorated the biochemical changes in rat testis by reducing sialic acid, total cholesterol, and total protein at p < 0.05. Histological and ultrastructural observations revealed that supplementation with TRF improved the morphological changes in testis in the TRF + FNT group. In conclusion, TRF was able to partially mitigate testicular damage in FNT-intoxicated rats.
Introduction
Environmental toxicants such as organophosphate (OP) insecticides have been identified as non-genetic factors contributing to testis damage.1 Fenitrothion [O,O-dimethyl-O-(3-methyl-4-nitrophenyl) phosphorothioate] (FNT) is a broad-spectrum OP insecticide, widely used for pest control in food products such as fruit, rice, vegetables, and cereals. It is also employed in the public health sector for controlling vector-borne diseases.2 Most OPs, including FNT, exert their toxic effects by inhibiting acetylcholinesterase (AChE) activity. However, oxidative stress has been recognized as the main mechanism underlying OP toxicity from repeated exposure.3 FNT at a dose of 20 mg kg−1 for 28 days augmented oxidative damage in rat liver, kidney, and testis through its ability to increase the generation of free radicals, as well as through significant changes in endogenous antioxidant properties.4,5
OP is metabolized in the liver by CYP450, and disturbance of respiration in mitochondria during OP exposure leads to an increase in reactive oxygen species (ROS) formation.3 The testis is susceptible to ROS due to the high abundance of polyunsaturated fatty acids (PUFA) in this tissue, and to the prominent presence of ROS-generating systems such as mitochondria, xanthine-oxidases, NADPH-oxidases, and CYP450s.6 The accumulation of ROS in the testis causes an imbalance of homeostatic antioxidant defence systems, thus enhancing oxidative damage to lipids, proteins, and DNA.6 This damage may accelerate apoptosis in germ cells.7 In the mammalian testis, apoptosis is an active cellular process that plays an important role in controlling spermatogenic cell development.8 However, the number of apoptotic germ cells in rats can be increased after exposure to xenobiotic agents such as pesticides.8
As a response to stressful stimuli, cells have the ability to express the stress response, which is indicated by an increase in heat shock protein (HSP) expression.9 HSP is a group of stress proteins. A number of HSPs have been identified in the testis, and their roles in male reproduction are gaining more and more attention. The 70 kDa HSP (HSP70) acts as a molecular chaperone, assisting in the correct folding, assembly, and disassembly of protein complexes.10 Altered expression of HSP70 has been associated with pathogenesis leading to male infertility. The evaluation of this stress protein is therefore relevant to reproductive toxicology.9 The expression of HSP70 increases steadily in the testis after exposure to xenobiotic agents such as OP and organochlorine, and is a sign of oxidative stress.11 In addition, oxidative stress can affect a variety of physiological functions in the male reproductive tract such as spermatogenesis, which results in biochemical and morphological changes affecting male reproduction.1 Some biochemical parameters, such as total cholesterol, protein, and sialic acid, have been found to be altered in rats exposed to OPs, and this has been shown to cause defects in male reproductive functions.12–14
Various exogenous antioxidants, including vitamin E, have been employed in several clinical and experimental trials as possible treatment for OP-induced testicular damage.15,16 Vitamin E is a lipid-soluble antioxidant with two isomeric forms: tocopherol and tocotrienol. Alpha-tocopherol has shown potential in reducing OP-induced testicular damage, but whether this isomer of vitamin E can be used as a therapeutic tool for reducing testicular toxicity remains unclear.15,16 Compared with α-tocopherol, tocotrienol possesses superior antioxidant,17 neuroprotective18 and anti-hypercholesterolemic19 effects. Vitamin E derived naturally from palm oil mainly consists of tocotrienol. It is therefore also known as palm oil tocotrienol-rich fraction (TRF). Studies have shown that TRF acts as a potent antioxidant,17 anti-atherosclerotic,20 and anti-hypercholesterolemic19 under different pathological conditions. Furthermore, TRF at a dose of 200 mg kg−1 has shown protective effects in reducing oxidative damage to red blood cells and arteries in diabetic rats20,21 as well as kidneys and pancreas in OP-treated rats.22,23 In addition, TRF at this dose does not show any toxicity effects in normal rats.22,23 Thus, the present study was designed to investigate the potential effects of TRF at a dose of 200 mg kg−1 in reducing the oxidative damage, biochemical changes, and morphological alterations induced by FNT in the rat testis.
Materials and methods
Chemicals
FNT was purchased from SUPELCO Analytical, USA with lot number: LB75917; the purity of the chemicals used in this study was 99.9%. The palm oil TRF (Gold Tri.E 70), containing 171.1 mg of α-tocopherol, 190.4 mg of α-tocotrienol, 36.0 mg of β-tocotrienol, 211.2 mg of γ-tocotrienol, and 150.4 mg of δ-tocotrienol, was purchased from Sime-Darby, Malaysia. All other chemicals and reagents were purchased from Sigma-Aldrich, St. Louis, USA, except for 2,4-dinitrophenylhydrazine, which was purchased from Mallinckrodt Chemicals, Austria. Nitro blue tetrazolium and reduced glutathione were purchased from Merck, Darmstadt, Germany.
Animals and treatment
Male Sprague-Dawley rats (n = 40), weighing 230–250 g body weight, were obtained from the Laboratory Animal Resource Unit, Faculty of Medicine, Universiti Kebangsaan Malaysia, Kuala Lumpur, Malaysia. All rats were maintained in polypropylene cages at an ambient temperature of 27 ± 2 °C with 12-h-light/12-h-dark cycles. Rats were allowed free access to standard chow and water ad libitum during the study. The experiments were carried out in accordance with the regulations for the care and use of laboratory animals, and this study was approved by the Institutional Animal Ethics Committee with resolution number: FSKB/BIOMED/2010/BALKIS/14-JULY/311-AUGUST-2010-JULY-2011.
The rats were randomly divided into four groups, each comprised of ten rats. Group 1 (control): all rats received corn oil at a dose of 1 mg kg−1; Group 2 (TRF): all rats received palm oil TRF at a dose of 200 mg kg−1; Group 3 (FNT): all rats received FNT at a dose of 20 mg kg−1 (1/30 LD50); and Group 4 (TRF + FNT): all rats received TRF at a dose of 200 mg kg−1, 30 minutes prior to administration of 20 mg kg−1 FNT. The corn oil, TRF, and FNT were given orally by gavage for 28 days daily between 9:00 and 10:00 am. At the end of 28 days, the rats were anesthetized by inhalation of diethyl ether. Blood and testicular tissue samples were retrieved for oxidative stress and apoptotic markers, morphological evaluation, and a reproductive biochemical assay.
Determination of antioxidant status and oxidative stress markers
The obtained testis was homogenized in ice cold 50 mM phosphate buffer, pH 7.4, enriched with 0.25 M sucrose to obtain a 10% homogenate. The homogenized testis samples were assessed for activity of antioxidant enzymes such as superoxide dismutase (SOD), glutathione peroxidase (GPx), catalase (CAT), and glutathione s-transferase (GST), using the methods of Beyer and Fridovich,24 Lawrence and Burk,25 Aebi,26 and Habig and Jacoby,27 respectively. The levels of ferric-reducing antioxidant power (FRAP) and reduced glutathione (GSH) were assessed according to the method of Benzie and Strain28 and Ellman,29 respectively. Levels of malondialdehyde (MDA) were determined according to the method of Stocks and Dormandy30 while PC was measured as done by Levine et al.31 for lipid peroxidation and protein oxidation evaluation.
Western blot analysis of HSP70
The other testis sample was homogenized using a tissue
:
buffer ratio of 1
:
5 in ice cold 50 mM Tris, pH 7.4, containing 0.15 M NaCl, 10% glycerol (v/v), 1% NP-40 (v/v), 1 mM sodium fluoride, 1 mM sodium orthovanadate, 1 mM phenylmethylsulfonyl fluoride (PMSF), 1 mM EDTA, 150 mM bestatin, 1 mM leupeptin, and 1 mM aprotinin. The homogenate was centrifuged at 10
000g, 4 °C, for 10 minutes. The protein concentration was determined by the method of Lowry et al.32 Equal protein concentrations of the testis homogenate were separated by electrophoresis on a 12% sodium dodecyl sulphate polyacrylamide gel as described by Laemmli.37 The resolved proteins were transferred to a nitrocellulose membrane by electrophoresis at 100 kV, 400 mA, for 90 minutes. The membrane was incubated in blocking solution containing 5% skim milk in 1% TBST (100 mM Tris-base, 1500 mM NaCl, and 2% Tween) for 1 hour at room temperature, followed by overnight incubation with an HSP70 primary antibody (Sigma, USA) (1
:
10
000) at 4 °C, shaking. The following day, blots were washed 3 times in TBST buffer for 5 minutes each, and incubated in secondary antibody solution containing horseradish peroxidase-conjugated anti-mouse immunoglobulin (Promega, USA) (1
:
5000), for 1 hour. Immunodetection of the protein was visualized through an enhanced chemiluminescence (ECL) system (Vilber Lourmat – Fusion Fx, German). The bands were scanned and quantified by densitometry.
TUNEL assay of testis samples
The testis was assayed by TUNEL using a TACS® 2 TdT DAB Kit according to the manufacturer's instructions. Briefly, the testis was immediately fixed in 10% formalin solution and embedded with paraffin after one week, based on the kit procedure. A 3 μm section was cut and the slide was warmed at 57 °C for 5 minutes and then dewaxed. Then, the slides were dehydrated and digested with 20 μg ml−1 of proteinase-K for 15 minutes at 37 °C. Endogenous peroxide was quenched in 3% hydrogen peroxide in methanol for 5 minutes at room temperature. Apoptotic germ cells were labeled using terminal deoxynucleotidyl transferase (TdT) buffer in a moist chamber at 37 °C for 60 minutes. The sections were then rinsed and incubated with 50 μl of strep-HRP at 37 °C for 10 minutes. For color development, the slides were rinsed and immersed in DAB solution for 7 minutes at room temperature in the dark, rinsed and counterstained with 1% methyl green, dehydrated, and, finally, coverslips were mounted. To determine germ cell apoptosis, 100 seminiferous tubules were assessed under a light microscope.
Biochemical analysis
The obtained plasma and homogenized testis were further analysed for total protein, total cholesterol, and sialic acid. Total protein and cholesterol were evaluated based on the method of Lowry et al. (1951)32 and Franey and Amador (1968),33 respectively. Sialic acid was assessed using a QuantiChrom sialic acid assay kit (DSLA-100; BioAssay Systems, USA). The principle of the test is based on the method of Waren (1959).34
Histological analysis
The 10% formalin-fixed testis samples were further processed using a standard histological procedure. Embedded paraffin tissue blocks were cut to obtain 5 μm thicknesses and stained with hematoxylin and eosin (H&E). Morphological changes were observed under 40× magnification and verified by a histopathological expert.
For seminiferous tubule evaluation, PAS staining was done using the method of Ochei and Kolhatkar (2002).35 For each rat, 100 seminiferous tubules were evaluated for pathology and spermatogenesis status. For pathological evaluation, the seminiferous tubules were counted and categorised as normal, sloughing, atrophied, or with germ cell degeneration, based on the method of Sayim (2007).36 Seminiferous tubules with organised germ cells were categorised as normal; tubules with disorganised germ cells and germ cells in the lumen of the tubule were categorised as sloughing; tubules with a few or absent germ cells were categorised as atrophied, and the presence of round spermatids, vacuolated cells, and/or giant cells indicated degenerated germ cells. The index of spermatogenesis was calculated based on the spermatogenesis stage and Johnson's score.38
The other testis was cut into small pieces (1 mm3) and immersed immediately in 2.5% glutaraldehyde, 0.1 M PBS for one hour at room temperature. Samples were than post-fixed with osmium tetroxide for another hour, dehydrated four times in 70, 90, 100, and 100% acetone, followed by immersion in acetone
:
resin (1
:
1) for five minutes each. Samples were embedded in epoxy resin, cut into ultrathin slices (90 nm) and observed using a transmission electron microscope, Tecnai G2 (FEI, USA), at 100 kV.
Statistical analysis
The obtained data were expressed as mean ± SEM. All data were checked for normality and the normal data were further evaluated by one-way analysis of variance (ANOVA) followed by Tukey's post-hoc multiple comparison, using the Statistical Packages for the Social Sciences (SPSS) version 17.0. Results were considered statistically significant at p < 0.05.
Results
Antioxidant status and oxidative stress markers
Antioxidant status and oxidative stress markers for the four groups are shown in Table 1. Significantly reduced SOD, GPX, CAT, and GST were observed in the FNT-treated group compared with the control and TRF groups (p < 0.05). The activities of SOD, CAT, and GST were significantly increased in the TRF + FNT group compared with the FNT-only group (p < 0.05). Nevertheless, the same trend was also observed in the GPx activity of the TRF + FNT group when compared with the FNT-only group, although this difference did not reach significance. The levels of FRAP and GSH were significantly lower in the FNT-treated rats compared with the control and TRF-only groups (p < 0.05). Meanwhile, the co-administration of TRF in FNT-treated rats significantly increased FRAP and GSH levels compared with the FNT-only group (p < 0.05). Levels of both MDA and PC were significantly increased in the FNT group compared with the control and TRF groups (p < 0.05). Meanwhile, supplementation with TRF in FNT-treated rats significantly decreased MDA and PC levels compared with the FNT-only group (p < 0.05).
Table 1 Effects of TRF on oxidative stress markers and antioxidant status in experimental rats
Parameters |
Control |
TRF |
FNT |
TRF + FNT |
Values are expressed as mean ± SEM. a Significantly different compared to the control group (p < 0.05); bsignificantly different compared to the TRF group (p < 0.05); csignificantly different compared to the FNT group (p < 0.05). |
SOD (unit min−1 per mg protein) |
0.78 ± 0.03 |
0.77 ± 0.04 |
0.53 ± 0.04a,b |
0.73 ± 0.06c |
CAT (nmol min−1 per mg protein) |
5.73 ± 0.38 |
5.34 ± 0.32 |
4.10 ± 0.14a,b |
5.05 ± 0.21c |
GPx (nmol min−1 per mg protein) |
3.12 ± 0.04 |
3.14 ± 0.12 |
2.52 ± 0.11a,b |
2.73 ± 0.14 |
GST (μmol min−1 per mg protein) |
6.92 ± 0.19 |
6.93 ± 0.30 |
5.20 ± 0.11a,b |
6.67 ± 0.35c |
GSH (mmol per mg protein) |
0.034 ± 0.001 |
0.035 ± 0.002 |
0.024 ± 0.002a,b |
0.034 ± 0.002c |
FRAP value (μM) |
1.07 ± 0.01 |
1.09 ± 0.01 |
0.97 ± 0.02a,b |
1.06 ± 0.02c |
MDA (μmol per mg protein) |
0.39 ± 0.02 |
0.39 ± 0.02 |
0.58 ± 0.03a,b |
0.44 ± 0.02c |
PC (nmol per mg protein) |
0.039 ± 0.005 |
0.038 ± 0.004 |
0.119 ± 0.011a,b |
0.058 ± 0.013c |
HSP70 levels
A significant increase in HSP70 levels was observed in FNT-treated rats compared with the control and TRF groups (p < 0.05). After treatment, TRF normalized the HSP70 levels in FNT + TRF rats compared with rats treated with FNT only (p < 0.05) (Fig. 1).
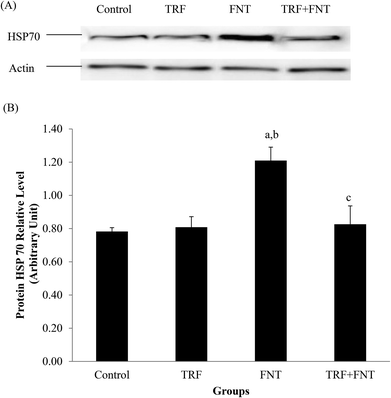 |
| Fig. 1 Immunoblot analysis of HSP70 expression in the rat testis. (A) Representative immunoblot showing an induction of HSP70 after FNT administration. The blot was probed with actin (42 kDa, bottom panel) and is representative of six replications. (B) Graph representing densitometrically scanned and quantified results corresponding to (A). (a) is significantly different from the control group at p < 0.05; (b) is significantly different from the TRF group at p < 0.05; (c) is significantly different from the FNT group at p < 0.05. | |
Germ cell apoptosis
A significant increase in the number of apoptotic germ cells was seen in FNT-treated rats compared with the control and TRF groups (p < 0.05) (Fig. 2). Meanwhile, the number of apoptotic germ cells decreased in the TRF + FNT group, compared with the FNT-only group (p < 0.05).
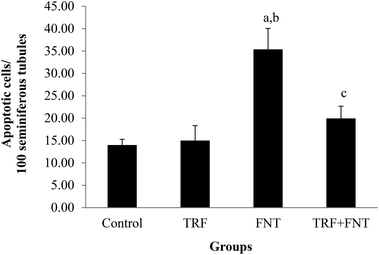 |
| Fig. 2 Apoptotic germ cells in rat testis. (a) is significantly different compared to the control group at p < 0.001, (b) is significantly different compared to the TRF group at p < 0.01, and (c) is significantly different compared to the FNT group at p < 0.01. | |
Biochemical evaluation
A significant reduction in sialic acid concentration was found in the plasma of the FNT-treated group compared with the control and TRF groups (p < 0.05). In addition, the sialic acid concentration in the testes of FNT-group rats was significantly increased compared with that in control- and TRF-group rats (p < 0.05). However, a significant decline in sialic acid concentration in the plasma of the TRF + FNT-group rats was found, compared with that in the FNT-only rats (p < 0.05) (Table 2).
Table 2 Biochemical analysis of plasma and testis in experimental rats
|
Plasma |
Testis |
Control |
TRF |
FNT |
TRF + FNT |
Control |
TRF |
FNT |
TRF + FNT |
Values are expressed as mean ± SEM. a Significantly different compared to the control group (p < 0.05); b significantly different compared to TRF group (p < 0.05); c significantly different compared to FNT group (p < 0.05). |
Sialic acid (μM) |
770.23 ± 35.48 |
769.64 ± 30.83 |
580.83 ± 63.67a,b |
755.28 ± 28.05c |
72.50 ± 1.83 |
74.43 ± 3.12 |
89.44 ± 3.05a,b |
81.07 ± 3.11 |
Cholesterol (mg ml−1) |
0.59 ± 0.03 |
0.61 ± 0.04 |
0.78 ± 0.02a,b |
0.67 ± 0.02 |
0.11 ± 0.01 |
0.12 ± 0.01 |
0.18 ± 0.01a,b |
0.14 ± 0.02c |
Protein (mg ml−1) |
59.18 ± 2.18 |
59.73 ± 1.77 |
69.97 ± 1.42a,b |
59.49 ± 2.34c |
14.65 ± 0.41 |
16.16 ± 0.45 |
19.12 ± 0.98a,b |
16.65 ± 0.82 |
The total cholesterol and total protein in the testis and plasma of FNT-treated rats were significantly increased compared with the control and TRF-treated rats (p < 0.05). However, compared with the FNT-only group, the TRF-supplemented group showed significantly increased total protein and total cholesterol in the plasma and testes, respectively (p < 0.05) (Table 2).
Seminiferous tubule evaluation
With regard to pathology, significantly fewer normal seminiferous tubules were found in the FNT-treated group compared with the control and TRF groups (p < 0.05), and seminiferous tubules with sloughing, atrophy, and germ cell degeneration were significantly increased in the FNT-treated group compared with the control and TRF groups (p < 0.05). The TRF + FNT group, however, had a significantly greater normal seminiferous tubule count than the FNT-only group. Furthermore, atrophied seminiferous tubules and tubules with germ cell degeneration were significantly reduced in the TRF + FNT group compared with the FNT-only group (P < 0.05) (Table 3).
Table 3 Classification of seminiferous tubules in experimental groups
Classification of seminiferous tubules |
Control |
TRF |
FNT |
TRF + FNT |
Values are expressed as mean ± SEM. aSignificantly different compared to control group (p < 0.05); bsignificantly different compared to TRF group (p < 0.05); csignificantly different compared to FNT group (p < 0.05). |
Pathological condition (%)
|
Normal |
75.00 ± 3.40 |
73.00 ± 4.32 |
22.45 ± 6.42a,b |
57.03 ± 9.77c |
Sloughing |
24.00 ± 2.02 |
25.00 ± 3.97 |
33.37 ± 4.50 |
32.05 ± 7.57 |
Atrophy |
0.00 ± 0.00 |
0.00 ± 0.00 |
5.50 ± 1.18a,b |
0.19 ± 0.02c |
Germ cells degeneration |
1.00 ± 0.22 |
2.00 ± 0.89 |
38.91 ± 6.77a,b |
10.55 ± 2.81c |
Index of spermatogenesis
|
9.53 ± 0.04 |
9.56 ± 0.06 |
7.71 ± 0.47a,b |
9.08 ± 0.23c |
The index of spermatogenesis was also significantly reduced in FNT-treated rats compared with the control and TRF groups (p < 0.05). However, the index of spermatogenesis was significantly increased in the TRF + FNT group compared with the FNT-only group (p < 0.05) (Table 3).
Light microscopic examination
Control and TRF rats exhibited normal seminiferous tubule structure, i.e., with layers of connective tissue and myoid cells and lined with spermatogenic cells and Sertoli cells. Spermatogenic cells were arranged from the basal compartment to the lumen in the following order: spermatogonia, spermatocytes, spermatids, and spermatozoa. Sertoli cells were pyramidal in shape and were found in between the spermatogenic cells. Leydig cells were found in the interstitial space between the seminiferous tubules (Fig. 3a and 3b).
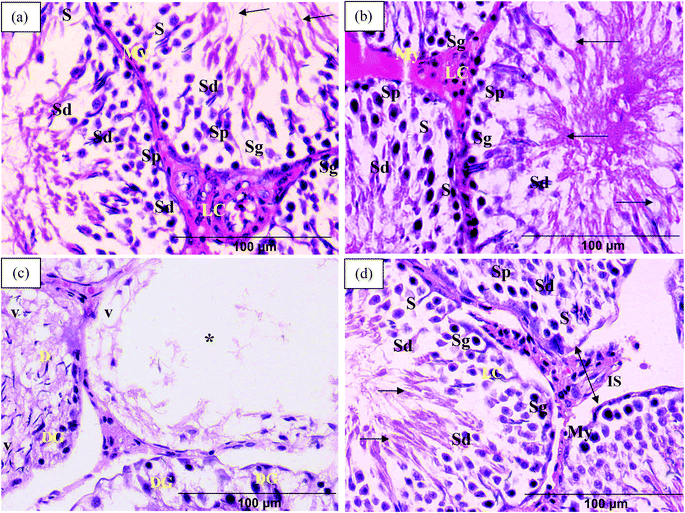 |
| Fig. 3 Light photomicrograph of the experimental rat testis (hematoxylin & eosin [H&E] stain, 40×). Sections of seminiferous tubules of control (a) and TRF (b) group rats in the presence of myoid cells (My) and connective tissue. Spermatogonia (Sg), spermatocyte (Sp), spermatid (Sd), Sertoli (S) cells and spermatozoa (black arrow) with heads attached to the germ cell lining and tails towards the lumen. Leydig cells (LC) dominate the interstitial space in between the seminiferous tubules. (c) Disorganisation (D) of seminiferous tubules in FNT-treated rats with marked vacuolations (V) and germ-cell degeneration (DG). The lumen, empty of germ cells, is clearly visible, as are massive vacuolations (*). (d) Sections of seminiferous tubules of TRF + FNT rats, showing attenuated severity, in the presence of normal germ cells. Widening of the interstitial space (↔) was noted. | |
Examination of seminiferous tubule sections from FNT-intoxicated rats revealed disorganisation of germ cells with marked vacuolations and germ cell degeneration. The vacuolations also served to separate the germ cells from the basal wall. Also found in FNT-treated rats were massive vacuolations that caused the germ cells to disappear into the lumen of the seminiferous tubules (Fig. 3c). TRF-supplemented rats, however, showed potential for amelioration of the histopathological changes induced by FNT, although the interstitial spaces between seminiferous tubules were still found to be increased within some tubules (Fig. 3d).
Electron microscopic examination
Electron microscopic examination of control and TRF rats showed normal seminiferous tubule wall structure (Fig. 4a and b). The seminiferous tubules were surrounded by smooth basal lamina with elongated myoid cells. Sertoli cells were identified with numerous organelles and large nuclei. Mitochondria, smooth endoplasmic reticulum, lysosomes, and lipid droplets were noted in the cytoplasm. Spermatogonia cells were also found attached to the basal lamina. Their nuclei included several nucleoli located adjacent to or associated with the nuclear membrane. Primary spermatocytes were detected above the spermatogonia cells. These cells had been actively dividing, as confirmed by the appearance of dense granules, indicating aggregation of chromosomal structure. Mitochondria were also found surrounding the cytoplasm area (Fig. 4a and b). The presence of complex tight junctions was also noted in the TRF group as a boundary between spermatogonia and spermatocytes (Fig. 4b).
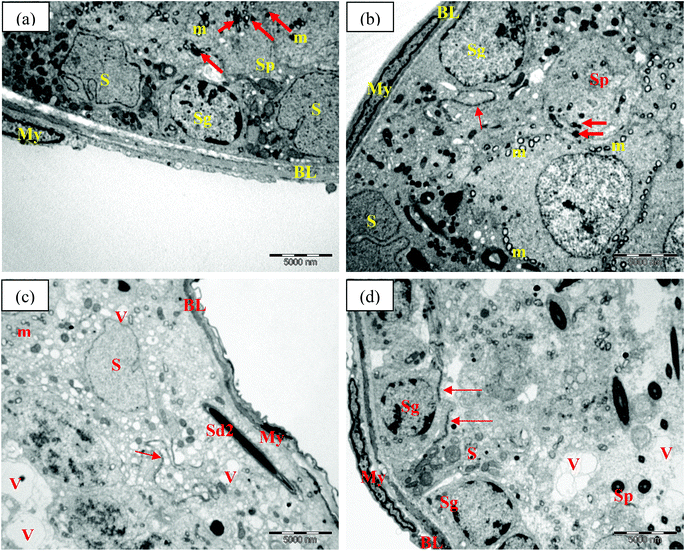 |
| Fig. 4 Electron micrograph of the walls of seminiferous tubules of experimental rats (scale ∼ 5000 nm), showing: (a) control and (b) TRF group rats, with myoid cells (My) at the basal lamina (BL), spermatogonia (Sg), spermatocytes (Sp), and Sertoli cells (S). Mitochondria (m), chromosomes with aggregates of dense granules (thick arrow), and tight junctions (thin arrow), which separate spermatogonia and spermatocytes, are also shown. (c) FNT group, with irregular basal lamina (BL) and shrunken myoid cells (My). Sertoli cells were separated by tight junctions with numerous vacuoles. Late spermatids (Sd2) near the basal lamina showing germ cell disorganisation are evident. (d) Protection of the walls of the seminiferous tubules from FNT-induced changes by TRF. Spermatogonia (Sg) attached to the basal lamina and myoid cells are visible, as are spermatocytes and Sertoli cells with tight junctions separating the spermatogonia from the other germ cells. | |
FNT-intoxicated rats exhibited alterations in the seminiferous tubule walls. These included irregularities in the basal lamina, shrunken myoid cells, and dense nuclei. Large vacuoles were scattered within the cytoplasm of Sertoli cells, with many vesicles. Late spermatids were also present near the basal lamina, which can explain the germ cell disorganisation seen in FNT-treated rats (Fig. 4c). TRF supplementation in FNT-treated rats preserved the ultrastructure of the seminiferous tubule wall, with normal basal lamina structure and myoid cells. However, some vacuoles were noted in the cytoplasma of Sertoli cells (Fig. 4d).
Fig. 5 shows the ultrastructure of spermatids at different stages of differentiation in the seminiferous tubules of the experimental rats. Control and TRF-treated rats exhibited normal structure in multiple early and late spermatids (Fig. 5a and b). The early spermatid nuclei were round in shape with uniformly scattered chromatin. The nuclear membrane was thickened at one side of the nucleus, and acrosomal granules and vesicles were present. Formation of acrosomal granules was detected near the nuclei of the spermatid cells (Fig. 5b). Mitochondria were located in line at the periphery of the spermatid cytoplasm (Fig. 5a and b). Rats exposed to FNT showed alterations in the ultrastructure of the spermatid, with more widely spaced mitochondria and aggregations of lipids and vacuoles in the cytoplasm (Fig. 5c). TRF supplementation in FNT-treated rats prevented these changes in the spermatid cells, indicated by the reduction in mitochondrial size. However, vacuoles were still noted in the cytoplasm of the cells (Fig. 5d).
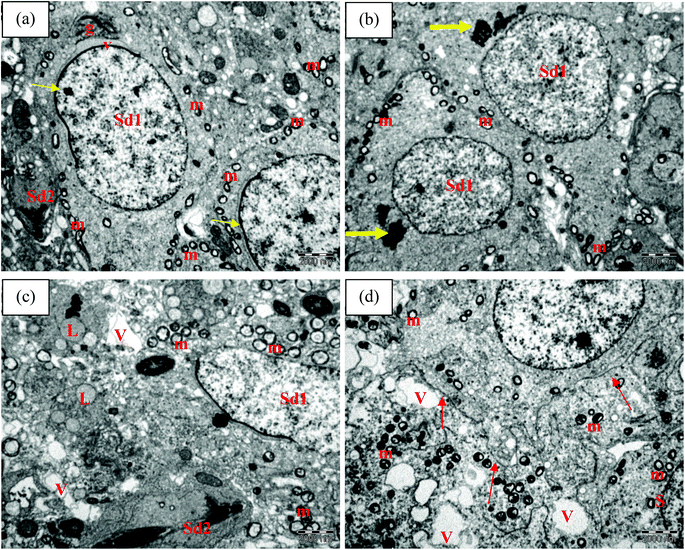 |
| Fig. 5 Electron micrograph of the testis of experimental rats (scale ∼ 2000 nm), showing multiple early spermatid cells (Sd1) and late spermatid cells (Sd2) of (a) control and (b) TRF groups, in the presence of acrosomal granules (g) and spreading acrosomal vesicles (v). The nuclear membrane is thickened at one side of the spermatid nucleus (thin arrow). Mitochondria (m) are visible at the periphery of the cytoplasm of the cells. The formation of acrosomal granules can be seen near the nuclei of the cells (thick arrow). (c) Widening space around mitochondria (m) and aggregation of lipids (L) and vacuoles (V) were noted in FNT-exposed rats. (d) Note the presence of tight junctions (red arrow) as a border surrounding germ cells in TRF + FNT rats. An abundance of vacuoles (V) and mitochondria (m) of smaller size were also found. | |
Ultrastructural features of the late spermatids in the experimental rats are shown in Fig. 6. The late spermatids in the control and TRF rats showed normal structure, with strongly elongated nuclei and condensed chromatin. The anterior of the nucleus was covered with an acrosomal cap (Fig. 6a and b). In the FNT-intoxicated rats, the late spermatids showed abnormal structure, with a lower density of chromatin. Vacuoles were also present, scattered in the cytoplasm of the cells (Fig. 6c). Meanwhile, rats that received FNT after TRF supplementation preserved normal late spermatid cell structure with strongly elongated nuclei and condensed chromatin, covered anteriorly by the acrosomal cap (Fig. 6d).
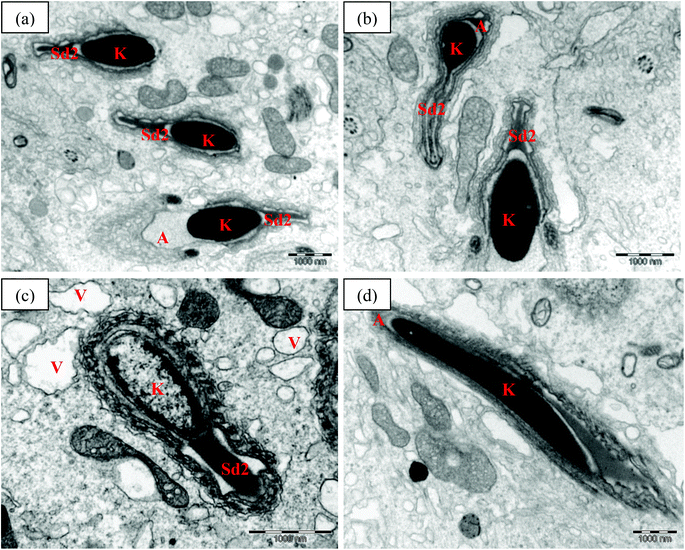 |
| Fig. 6 Electron micrograph of the testis in experimental rats (scale ∼ 1000 nm), showing late spermatid cells (Sd2) of (a) control and (b) TRF-exposed rats, with strongly elongated nuclei with condensed chromatin (K), covered anteriorly by the acrosomal cap (A). (c) Abnormal structure of late spermatid (Sd2) in FNT-exposed rats with lower-density chromatin (K). The presence of vacuoles (V) was also evident. (d) TRF + FNT rats showed normal structure of late spermatid cells (Sd2), with strongly elongated nuclei with condensed chromatin (K), covered anteriorly by the acrosomal cap (A). | |
Discussion
OP has the potential to induce oxidant injury in the male reproductive system due to the imbalance between ROS formation and antioxidant homeostasis.1 A biochemical defence mechanism in the form of antioxidants is required for preventing a progressive increase in ROS in the membranes and tissues and the resulting attack on biological molecules.39 Antioxidant supplements, such as vitamins and phenolic compounds, have been reported to improve and stabilize the activity and levels of endogenous antioxidants, thus reducing oxidative damage to susceptible cellular constituents.40 The present study found that TRF supplementation can increase the levels of enzymatic and non-enzymatic antioxidants, while reducing lipid peroxidation and protein oxidation. In line with the present results, exogenous antioxidants such as Zingiber officinale and vitamins E and C have been found to increase the activities of antioxidant enzymes, thereby inhibiting the propagation of lipid radicals in rats intoxicated with organophosphates. This mechanism might protect cellular constituents such as lipids, proteins, carbohydrates, and DNA from oxidative damage.41,42 Furthermore, supplemental TRF has been found to be fully utilized as an antioxidant scavenging free radical. The powerful antioxidant properties of TRF serve to maintain enzymatic and non-enzymatic antioxidant levels, thus protecting the cellular environment from oxidative stress.22,23
In the present study, TRF mainly consisted of tocotrienol and α-tocopherol at a ratio of 76
:
24. Both tocotrienol and tocopherol possess the same antioxidant mechanism, which works through the donation of a hydrogen (electron) to lipid radicals in the biological membrane, thus inhibiting the chain reaction in the lipid membrane.43 However, their side chains differ in their tail structure. The unsaturated isoprenoid side chain in tocotrienol influences the ability of this isomer to penetrate and easily integrate into the lipid membrane and distribute itself throughout the membrane. In addition, it serves to maintain the cell membrane, as well as effectively recycle its radicals formation (tocotrienoxyl).17 Due to these differences, tocotrienol is a more potent antioxidant than tocopherol,17 although tocopherol has a higher bioavailability than tocotrienol.44 Previous research has found that, even at small concentrations, tocotrienol shows powerful efficacy in combating free radicals.45 In the present study, tocopherol might be the first isomer to arrive at the testis, with its role in combating the free radicals followed by the superior antioxidant effects of tocotrienol, thus providing a two-pronged defence against the testicular toxicity induced by FNT.
Oxidative stress conditions may influence the expression of HSP70.11 The present study found that FNT causes oxidative stress in the rat testis, and this might increase the expression of HSP70. Upon repeated OP exposure, increased ROS formation leads to lipid peroxidation and protein oxidation, which causes changes in cellular function and protein denaturation. These mechanisms trigger the expression of HSP70 under many pathological conditions.46 Therefore, antioxidant application may reduce the expression of HSP70 through its ability to control oxidative stress. TRF showed potential in reducing HSP70 expression in FNT-treated rats, possibly due to its antioxidant properties. In agreement with the present study, Calabrese et al.47 found that vitamin E reduced HSP70 expression in skin exposed to H2O2, through its antioxidants properties. A combination of α-tocopherol, γ-tocopherol, and vitamin C was also capable of reducing HSP70 expression in skeletal muscle after exercise.48 Both α-tocopherol and vitamin C have been fully utilized as antioxidants, while γ-tocopherol has played a role in blocking HSP70 gene transcription.48 Since the supplemented TRF consists of α-tocopherol and α-, γ-, δ- and β-tocotrienol, the same mechanism might also be at work in the FNT rat testis supplemented with TRF.
Free radicals such as hydroxyl and peroxyl molecules may cause gene activation through covalent binding with DNA bases, deoxyribosyl, and DNA backbone. This gene activation might lead to accelerated germ cell apoptosis.7 FNT, which has been found to cause oxidative damage in rat testis, might also cause apoptosis of germ cells. However, in the present study, supplementation with TRF showed the potential to reduce apoptosis in the germ cells of FNT-exposed rats through a mechanism based on the protection of the cells from oxidative stress. Vitamins E and C have demonstrated free-radical scavenging ability that can protect cells from oxidative and apoptotic damage in rats exposed to chlorpyrifos.49 In addition, TRF also has the ability to increase the expression of genes involved in the synthesis of DNA repair enzymes.50 This might explain the reduction in apoptotic germ cell count in FNT-treated rats supplemented with TRF.
Oxidative stress might also be responsible for adverse effects on male reproductive functions stemming from reduced spermatogenesis and biochemical alterations.51 In the present study, total cholesterol, total protein, and sialic acid in the plasma and testis were altered in FNT-intoxicated rats. Changes in sialic acid concentration and total cholesterol indicate altered androgen production and reduced spermatogenesis.52 In addition, the increase in total protein might be due to acute-phase protein formation, which can also be used as an indicator of early tissue reaction.53 In rats, TRF can ameliorate biochemical changes induced by FNT in both plasma and testicular tissue. The application of vitamin E has been found to reduce the biochemical changes in mercury chloride-intoxicated rats. Previous researchers have suggested that vitamin E works by scavenging free radicals, thereby preventing oxidative damage and maintaining the biochemical functions and structural integrity of germ cell membranes.54 This might explain the action of TRF in reducing the biochemical changes in FNT-treated rats through its antioxidant properties.
OP has been found to cause morphological alterations in the testis due to oxidative stress.55,56 In addition to oxidative stress, toxicant-induced Sertoli cell damage has been found to result in reduced spermatogenesis.57 Toxicants have the ability to pass through the blood–testis barrier and interfere with germ and Sertoli cell structure, thus inducing morphological alteration in the testis.14,54 Light and electron microscopic evaluations have shown that FNT induces various histopathological alterations and reduces spermatogenesis in the rat testis. Previous research has found that repeated exposure to FNT results in histological and ultrastructural alterations in the rat testis due to oxidative stress.58 The occurrence of lipid peroxidation, protein oxidation, and biochemical alterations, which can be observed early in the intoxication process, might explain the subsequent morphological alterations in the testis in FNT-treated rats. Supplementation with TRF has the potential to attenuate these morphological changes. Antioxidant vitamins such as vitamins C and E, as well as phenolic compounds such as green tea, have been found to reduce the morphological alteration in testis induced by OP.4,56 This would explain the present observation of mitigation of the effects of FNT by TRF; TRF could compensate for oxidative damage through its potent antioxidant ability, thus protecting against histopathological alterations in the rat testis induced by FNT.
In conclusion, TRF has the ability to protect cells from oxidative stress through its antioxidant properties. Through its control of oxidative stress, TRF can potentially reduce the expression of HSP70, thereby reducing apoptotic damage to germ cells, increasing testicular function, and attenuating morphological changes in FNT-treated rats. Considering this potential, further molecular investigation is needed into the apoptotic signaling pathway, as well as the Nrf2-ARE pathway, in order to gain a better understanding of TRF mechanisms responsible for the protection of germ cells from apoptosis and oxidative damage in FNT-exposed rats.
Acknowledgements
The authors would like to thank the Ministry of Higher Education and Universiti Kebangsaan Malaysia for funding this research (UKM-GUP-2011–126). We also extend our gratitude to the Director of the Institute for Medical Research (IMR), Malaysia, for permission to use the electron microscopy facilities. We would also like to express our gratitude to the staff of the Programme of Biomedical Science, School of Diagnostic and Applied Health Sciences, Faculty of Health Sciences, Universiti Kebangsaan Malaysia, and the Electron Microscopy Unit, IMR, Malaysia, for providing research facilities.
References
- A. Agarwal, K. P. Nallella, S. S. Allamaneni and T. M. Said, Reprod. Biomed. Online, 2004, 8(6), 616–627 CrossRef CAS.
-
WHO, Environmental Health Criteria, ed. World Health Organization, Geneva, 1992, vol. 133, pp. 75–81 Search PubMed.
- A. Lukaszewicz-Hussain, Pestic. Biochem. Physiol., 2010, 98, 145–150 CrossRef CAS PubMed.
- M. E. A. Elhalwagy, N. S. Darwish and E. M. Zaher, Pestic. Biochem. Physiol., 2008, 91, 81–89 CrossRef CAS PubMed.
- I. S. Taib, S. B. Budin, A. R. Ghazali, P. A. Jayusman, S. R. Louis and J. Mohamed, Clinics, 2013, 68(1), 93–100 CrossRef.
- R. J. Aitken and S. D. Roman, Oxid. Med. Cell. Longevity, 2008, 1(1), 15–24 CrossRef.
- M. Valko, C. Rhodes, J. Moncol, M. Izakovic and M. Mazur, Chem.-Biol. Interact., 2006, 160(1), 1–40 CrossRef CAS PubMed.
- A. M. Gawish, J. Aquacult. Res. Dev., 2010, 1, 101 Search PubMed.
- H. L. Feng, J. I. Sandlow and A. E. Sparks, Fertil. Steril., 2001, 76(6), 1136–1139 CrossRef CAS.
- D. J. Dix, J. W. Allen, B. W. Collins, P. Poorman-Allen, C. Mori, D. R. Blizard, P. R. Brown, E. H. Goulding, B. D. Strong and E. M. Eddy, Development, 1997, 124(22), 4595–4603 CAS.
- B. Saradha, S. Vaithinathan and P. P. Mathur, Toxicology, 2008, 243(1–2), 116–123 CrossRef CAS PubMed.
- S. C. Joshi, R. Mathur, A. Gajraj and T. Sharma, Environ. Toxicol. Pharmacol., 2003, 14(3), 91–98 CrossRef CAS.
- S. C. Joshi and B. Bansal, Int. J. Toxicol. Appl. Pharmacol., 2012, 2(1), 6–11 Search PubMed.
- S. C. Joshi, R. Mathur and N. Gulati, Toxicol. Ind. Health, 2007, 23(7), 439–444 CrossRef CAS PubMed.
- M. Uzunhisarcikli, Y. Kalender, K. Dirican, S. Kalender, A. Ogutcu and F. Buyukkomurcu, Pestic. Biochem. Physiol., 2007, 87, 115–122 CrossRef CAS PubMed.
- E. K. Dirican and Y. Kalender, Exp. Toxicol. Pathol., 2012, 64, 821–830 CrossRef CAS PubMed.
- L. Packer, S. U. Weber and G. Rimbach, J. Nutr., 2001, 131(2), 369S–373S CAS.
- S. Khanna, S. Roy, H. Ryu, P. Bahadduri, P. W. Swaan, R. R. Ratan and C. K. Sen, J. Biol. Chem., 2003, 278(44), 43508–43515 CrossRef CAS PubMed.
- A. A. Qureshi, N. Qureshi, J. Wright, Z. Shen, G. Kramer, A. Gapor, Y. Chong, G. DeWitt, A. Ong and D. Peterson, Am. J. Clin. Nutr., 1991, 53(4), 1021S–1026S CAS.
- S. B. Budin, F. Othman, S. R. Louis, M. A. Bakar, S. Das and J. Mohamed, Clinics, 2009, 64(3), 235–244 CrossRef PubMed.
- F. A. Matough, S. B. Budin, Z. A. Hamid, S. R. Louis, N. Alwahaibi and J. Mohamed, Oxid. Antioxid. Med. Sci., 2012, 1(1), 59–68 Search PubMed.
- S. B. Budin, K. J. Han, P. A. Jayusman, I. S. Taib, A. R. Ghazali and J. Mohamed, J. Toxicol. Pathol., 2013, 26(2), 111 CrossRef PubMed.
- S. B. Budin, C. M. Han, P. A. Jayusman and I. S. Taib, Pak. J. Biol. Sci., 2012, 15(11), 517 CrossRef.
- W. Beyer and I. Fridovich, Anal. Biochem., 1987, 161, 559–566 CrossRef CAS.
- R. A. Lawrence and R. F. Burk, Biochem. Biophys. Res. Commun., 1976, 71(4), 952–958 CrossRef CAS.
- H. Aebi, Methods Enzymol., 1984, 105, 121–126 CAS.
- W. Habig and W. Jacoby, Methods Enzymol., 1981, 17, 398–405 Search PubMed.
- I. F. F. Benzie and J. J. Strain, Methods Enzymol., 1999, 299, 15–27 CAS.
- G. Ellman, Arch. Biochem. Biophys., 1959, 82(1), 70–77 CrossRef CAS.
- J. Stocks and T. L. Dormandy, J. Hematol., 1971, 20, 95–111 CAS.
- R. L. Levine, D. Garland, C. Oliver, A. Amici, I. Climent, A. Lenz, B. Ahn, S. Shaltiel and E. Stadtman, Methods Enzymol., 1990, 186, 464–478 CAS.
- O. Lowry, N. Rosebrough, A. Farr and R. Randall, J. Biol. Chem., 1951, 193, 265 CAS.
- R. Franey and E. Amador, Clin. Chim. Acta, 1968, 21, 255–263 CrossRef CAS.
- L. Warren, J. Biol. Chem., 1959, 234(8), 1971–1975 CAS.
-
J. Ochei and A. Kolhatkar, Medical Laboratory Science: Theory and Practice, Tata McGraw-Hill, New Delhi, 2002, pp. 173–174 Search PubMed.
- F. Sayim, Bull. Environ. Contam. Toxicol., 2007, 78(6), 479–484 CrossRef CAS PubMed.
- U. K. Laemmli, Nature, 1970, 227(5259), 680–685 CrossRef CAS.
- H. A. Johnson and E. P. Cronkite, Radiat. Res., 1959, 11(6), 825–831 CrossRef CAS.
- E. Cemeli, A. Baumgartner and D. Anderson, Mutat. Res./Rev. Mutat. Res., 2009, 681(1), 51–67 CrossRef CAS PubMed.
- A. Agarwal and L. H. Sekhon, Hum. Fertil., 2010, 13(4), 217–225 CrossRef PubMed.
- A. F. Ben, H. Fetoui, N. Zribi, F. Fakfakh and L. Ammar-Keskes, Andrologia, 2012, 1, 272–279 Search PubMed.
- B. Thongchuai, P. Narongchai, S. Narongchai, A. Panthong, N. Lertprasertsuk and C. Praputpittaya, Chiang Mai Med. J., 2010, 49(3), 81–88 Search PubMed.
- R. Brigelius-Flohe and M. G. Traber, FASEB J., 1999, 13(10), 1145–1155 CAS.
-
B. G. Sanders, K. Kline and W. Yu, Google Patents, WO 2001058889 A1, 2007 Search PubMed.
- S. Khanna, V. Patel, C. Rink, S. Roy and C. K. Sen, Free Radical Biol. Med., 2005, 39(10), 1310–1319 CrossRef CAS PubMed.
- S. C. Gupta, H. R. Siddique, N. Mathur, R. K. Mishra, D. K. Saxena and D. K. Chowdhuri, Toxicology, 2007, 238(1), 1–14 CrossRef CAS PubMed.
- V. Calabrese, G. Scapagnini, C. Catalano, T. E. Bates, D. Geraci, G. Pennisi and A. M. Giuffrida Stella, Int. J. Tissue React., 2001, 23(4), 127–135 CAS.
- C. P. Fischer, N. J. Hiscock, S. Basu, B. Vessby, A. Kallner, L.-B. Sjöberg, M. A. Febbraio and B. K. Pedersen, J. Appl. Physiol., 2006, 100(5), 1679–1687 CrossRef CAS PubMed.
- F. Yu, Z. Wang, B. Ju, Y. Wang, J. Wang and D. Bai, Exp. Toxicol. Pathol., 2008, 59, 415–423 CrossRef CAS PubMed.
- S.-F. Chin, N. A. A. Hamid, A. A. Latiff, Z. Zakaria, M. Mazlan, Y. A. M. Yusof, A. A. Karim, J. Ibahim, Z. Hamid and W. Z. W. Ngah, Nutrition, 2008, 24(1), 1–10 CrossRef CAS PubMed.
- N. Akhtar, M. Srivastava and R. Raizada, J. Environ. Biol., 2009, 30(6), 1047–1053 CAS.
- V. Dixit and R. Gupta, Planta Med., 1982, 46(12), 242–246 CrossRef CAS PubMed.
- F. H. Epstein, C. Gabay and I. Kushner, N. Engl. J. Med., 1999, 340(6), 448–454 CrossRef CAS PubMed.
- M. V. Rao and P. S. Sharma, Reprod. Toxicol., 2001, 15(6), 705–712 CrossRef CAS.
- L. Sarabia, I. Maurer and E. Bustos-Obregon, Ecotoxicol. Environ. Saf., 2009, 72, 663–668 CrossRef CAS PubMed.
- R. H. ElMazoudy, A. A. Attia and N. S. El-Shenawy, Pestic. Biochem. Physiol., 2011, 101, 175–181 CrossRef CAS PubMed.
- D. Creasy, Toxicol. Pathol., 2001, 29(1), 64–76 CrossRef CAS.
- M. O. Al-Jahdali and A. S. B. Bisher, J. Biol. Sci., 2007, 7(3), 520–525 CrossRef CAS.
|
This journal is © The Royal Society of Chemistry 2015 |