DOI:
10.1039/C5SC02428E
(Edge Article)
Chem. Sci., 2015,
6, 7336-7342
Domain-swapped cytochrome cb562 dimer and its nanocage encapsulating a Zn–SO4 cluster in the internal cavity†
Received
6th July 2015
, Accepted 22nd September 2015
First published on 22nd September 2015
Abstract
Protein nanostructures have been gaining in interest, along with developments in new methods for construction of novel nanostructures. We have previously shown that c-type cytochromes and myoglobin form oligomers by domain swapping. Herein, we show that a four-helix bundle protein cyt cb562, with the cyt b562 heme attached to the protein moiety by two Cys residues insertion, forms a domain-swapped dimer. Dimeric cyt cb562 did not dissociate to monomers at 4 °C, whereas dimeric cyt b562 dissociated under the same conditions, showing that heme attachment to the protein moiety stabilizes the domain-swapped structure. According to X-ray crystallographic analysis of dimeric cyt cb562, the two helices in the N-terminal region of one protomer interacted with the other two helices in the C-terminal region of the other protomer, where Lys51–Asp54 served as a hinge loop. The heme coordination structure of the dimer was similar to that of the monomer. In the crystal, three domain-swapped cyt cb562 dimers formed a unique cage structure with a Zn–SO4 cluster inside the cavity. The Zn–SO4 cluster consisted of fifteen Zn2+ and seven SO42− ions, whereas six additional Zn2+ ions were detected inside the cavity. The cage structure was stabilized by coordination of the amino acid side chains of the dimers to the Zn2+ ions and connection of two four-helix bundle units through the conformation-adjustable hinge loop. These results show that domain swapping can be applied in the construction of unique protein nanostructures.
Introduction
Protein nanostructures for development of artificial biomaterials have been constructed under various methods, such as chemical modification,1 disulfide bonding,2 cross-linking,3 computational design,4 carbon nanotube templating,5 metal coordination,6 protein–peptide tag interaction,7 and protein fusion.8 However, increase in protein nanostructure variety is desirable and therefore development of other methods such as domain swapping is necessary. Domain swapping has attracted attention as a mechanism of protein oligomerization, and reports on domain swapping have been increasing.9 In domain swapping, a secondary structural region or a domain of one protein molecule is replaced with the corresponding region or domain of another protein molecule.10 We have previously shown that small spherical heme proteins, c-type cytochrome (cyt) proteins and horse myoglobin (Mb), form oligomers by domain swapping.11 In the dimer and trimer of horse cyt c, the C-terminal α-helix domain swapped with the corresponding region of other molecules. In dimeric Pseudomonas aeruginosa (PA) cyt c551 and dimeric Hydrogenobacter thermophilus (HT) cyt c552, the region containing the N-terminal α-helix and heme was swapped.11b,d Previously, we proposed that the unstable loop region has a tendency to become a hinge loop for domain swapping in cyt c family proteins.11d In domain-swapped dimeric horse Mb, each active site consisted of two different protomers, and new long α-helices were formed by the E and F helices and the EF-loop of the original monomer.11c Although apoMb formed a dimer, a large amount of apoMb dissociated to monomers by incubation at 37 °C for 30 min, whereas holoMb oligomers did not dissociate under the same conditions.11c These results indicated that the Mb dimer was stabilized by heme binding to the protein.
Cyt b562 from E. coli is a relatively small (MW: ∼12
000) heme protein responsible for electron transfer in the periplasm.12 Owing to its simple four-helix bundle structure, cyt b562 is frequently used as a model for studying protein stability and folding.13 Cyt b562 from E. coli is composed of 106 amino acids, and four α-helices are arranged in an antiparallel orientation.14 Met7 and His102 are coordinated to the heme iron in cyt b562, where the heme may dissociate from the protein moiety. A c-type cyt b562 (cyt cb562) has been constructed by Barker and co-workers by replacing Arg98 and Tyr101 with Cys residues and introducing two covalent thioether bonds between the heme and protein moiety.15 Gray and co-workers have shown that the covalent attachment of the heme to the protein moiety in cyt cb562 increases its protein stability without perturbation in the structure and folding process from those of the wild-type protein (cyt b562).16
Tezcan and co-workers have constructed cyt cb562 nanostructures by introducing intermolecular interaction sites at the protein surface.6b,17 A cyt cb562 variant with two metal-chelating bis-His motifs at its surface has been shown to assemble into a tetramer, trimer and dimer through Zn2+, Ni2+ and Cu2+ coordination, respectively.17c Cage-like tetrahedral dodecamers have been constructed in the crystal lattice of another cyt cb562 variant.17f A cyt cb562 derivative with two additional Cys residues at its surface has been shown to assemble into a tetramer with a cryptand-like topology through selective formation of four interfacial disulfide bonds.17i A computationally designed dimer of cyt cb562 has been arranged by Zn2+ coordination into 1D helical nanotubes, and 2D or 3D crystalline arrays.6b
Recently, we have constructed a heterodimeric Mb with two different active sites by modifying the interface of two protomers in the domain-swapped wild-type dimer,18 demonstrating that domain swapping may be utilized to design artificial heme proteins. In this study, we obtained domain-swapped cyt cb562 oligomers, and found that the two helices (helices 1 and 2) in the N-terminal region of one protomer and the other two helices (helices 3 and 4) in the C-terminal region of the other protomer interact in the dimer. In the crystal, three cyt cb562 dimers formed a unique cage structure with a Zn–SO4 cluster inside the cavity, showing that domain-swapping may be utilized to construct unique nanostructures.
Results
Formation and stability of dimeric cyt b562 and dimeric cyt cb562
E. coli cyt b562 solution changed its colour from red to black by an addition of acetic acid up to 40% (v/v) (pH 1.9) to its neutral pH solution, although no precipitation was observed. Cyt b562 has been reported to denature at pH 2.3.19 Therefore, the colour change was ascribed to the heme dissociation from the cyt b562 protein moiety in the presence of 40% (v/v) acetic acid, causing change in the heme coordination. After lyophilization of the cyt b562 solution, the protein powder was dissolved in 50 mM potassium phosphate buffer, pH 7.0, and analysed by size exclusion chromatography. A small peak was observed at ∼11.1 mL in the elution curve, in addition to the monomer peak at ∼12.7 mL (Fig. S1a†). We attributed the ∼11.1 mL peak to the dimer. The amount of the dimer was estimated at ∼10% from the area in the chromatogram. Approximately 45% and 86% of the dimers dissociated to monomers by incubation at 4 °C for 2 and 12 h, respectively (Fig. S2A†), showing that dimeric cyt b562 is relatively unstable.
Attachment of the heme to the protein moiety in cyt b562 may stabilize the dimer, since the holoMb dimer was more stable than the apoMb dimer.11c To support this hypothesis, we used cyt cb562, in which the heme was covalently attached to the protein moiety through two thioether bonds by mutation of Arg98 and Tyr101 to Cys.15,16b,c A large amount of oligomers was obtained by an addition of acetic acid to the cyt cb562 solution up to 40% (v/v) and subsequent dissolution of the obtained precipitate with buffer (Fig. S1b†). In contrast to dimeric cyt b562, dimeric cyt cb562 did not dissociate to monomers by incubation at 4 °C for 12 h (Fig. S2B†), showing that dimeric cyt cb562 is more stable than dimeric cyt b562.
Optical absorption and circular dichroism measurements of dimeric cyt cb562
The peak wavelength and intensity of the Soret and Q bands of oxidized dimeric cyt cb562 were similar to those of the oxidized monomer, showing that the active site structure was similar between the monomer and dimer (Fig. 1A). Similar negative Cotton effects at 208 and 222 nm, characteristic of an α-helical structure, were observed in the circular dichroism (CD) spectra of oxidized monomeric and dimeric cyt cb562, indicating that the secondary structures also do not change significantly by the dimerization (Fig. 1B). It has been shown in other heme proteins, c-type cyts and Mb, that the secondary structures are similar between the monomer and domain-swapped dimer.11b–e
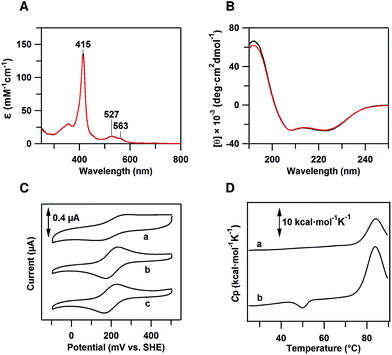 |
| Fig. 1 Characterization of dimeric cyt cb562. (A) Optical absorption spectra of oxidized monomeric (black) and dimeric (red) cyt cb562. (B) CD spectra of oxidized monomeric (black) and dimeric (red) cyt cb562. (C) Cyclic voltammograms of (a) monomeric E. coli cyt b562, (b) monomeric cyt cb562 and (c) dimeric cyt cb562. Scan rate was 50 mV s−1. (D) Differential scanning calorimetry thermograms of oxidized (a) monomeric and (b) dimeric cyt cb562. Scan rate was 1 °C min−1. Measurement conditions: sample concentration, (A) 10 μM, (B) 8 μM, (C) 200 μM and (D) 100 μM (heme unit); solvent, 50 mM potassium phosphate buffer; pH, 7.0. | |
Dimeric cyt cb562 redox potential
Cyclic voltammetry (CV) was performed to determine the midpoint redox potential (Em) of dimeric cyt cb562 (Fig. 1C). The Em value of the cyt cb562 monomer was obtained as 203 mV, similar to that reported previously (199–204 mV),20 and to that of cyt b562 (205 mV) (Fig. 1C). The Em value of the cyt cb562 dimer (198 mV) was similar to that of the cyt cb562 monomer. Dimeric cyt cb562 exhibited a similar high redox potential as the monomer, suggesting that the active site structure of the dimer is similar to that of the monomer.
Differential scanning calorimetry measurements of dimeric cyt cb562
Differential scanning calorimetry (DSC) measurements of oxidized monomeric and dimeric cyt cb562 were performed to investigate the thermodynamic properties of the dimer. A positive peak was observed at 83.9 °C in the DSC thermogram of the cyt cb562 monomer (Fig. 1D, a). We assigned this peak to denaturation of the cyt cb562 monomer, where the denaturation temperature of the cyt cb562 monomer was higher than that of the cyt b562 monomer (67.0 °C at pH 7.4; 73.8 °C at pH 5–6).13d However, an additional small negative peak was observed at 50.1 °C in the DSC thermogram of the cyt cb562 dimer (Fig. 1D, b). No peak was observed in the thermogram around 50 °C after dissociation of the cyt cb562 dimer to monomers by heating the dimer solution up to 70 °C (Fig. S3†). Therefore, we attributed the small negative peak to the dissociation of the cyt cb562 dimer to monomers. The area of this peak in the thermogram corresponds to the enthalpy change (ΔH) during the dissociation. The ΔH value for the dissociation of the cyt cb562 dimer to monomers was obtained as −13 ± 2 kcal mol−1 (per dimer), showing that the dimer is enthalpically disfavoured compared to the monomer.
Dimeric cyt cb562 crystal structure
We performed X-ray crystallographic analysis to elucidate the detailed structure of dimeric cyt cb562. The 1.85 Å resolution structure of dimeric cyt cb562 exhibited a domain-swapped structure (PDB ID: 5AWI) (Fig. 2B). The two helices in the N-terminal region (helices 1 and 2) of a protomer and the other two helices in the C-terminal region (helices 3 and 4) of the other protomer interacted in the dimer. There were two independent cyt cb562 molecules forming a dimer in the asymmetric unit of the dimeric cyt cb562 crystal. The position of the backbone of the dimer overlapped well with that of the monomer (Fig. S4A†). We calculated the root-mean-square deviation (rmsd) for the Cα atoms between the structures of the monomer (Fig. 2A; K59W, R98C and Y101C mutant of cyt b562, PDB ID: 2BC5) and dimer. Residues in the N-terminal region (Ala1–Asp50) before a protomer hinge loop (Lys51–Asp54) and residues in the C-terminal region (Ser55–Arg106) after the hinge loop in the other protomer in the dimer were compared with the corresponding structural region of the monomer. The rmsd values were less than 0.36 Å, indicating that the corresponding structures of the monomer and dimer were similar. The heme orientation and the amino acid side chain positions at the active site of the dimer also overlapped well with those of the monomer (Fig. S4B†). Met7 and His102 were axially coordinated to the heme iron in the dimer as in the monomer (Fig. 2C), although Met7 originated from the other protomer to which the heme belonged (Fig. 2D).
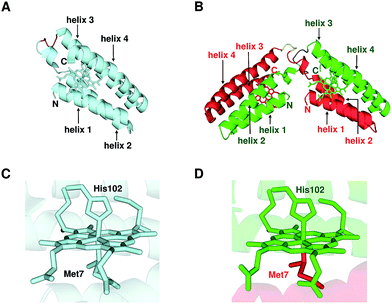 |
| Fig. 2 Crystal structures of oxidized monomeric and dimeric cyt cb562. (A) Protein structure of the monomer (K59W, R98C, and Y101C mutant of cyt b562; PDB ID: 2BC5). (B) Protein structure of the dimer solved in this study (PDB ID: 5AWI). (C) Active site structure of the monomer (PDB ID: 2BC5). (D) Active site structure of the dimer (PDB ID: 5AWI). The green and red regions in the dimer belong to different protomers. The hemes and the side chain atoms of heme-binding Cys residues (Cys98 and Cys101) and heme-coordinating residues (Met7 and His102) are shown as stick models. The N- and C-termini of the monomer and the protomers of the dimer are labelled as N and C, respectively. The α-helices are labelled helix 1, helix 2, helix 3 and helix 4 from the N-terminus. The hinge loop (Lys51–Asp54) is depicted in purple in the monomer, and blue and yellow in the dimer. | |
Three domain-swapped dimers of cyt cb562 formed a hollow cage-like structure in the crystal (Fig. 3A and B). The cage structure exhibited pseudo-D3 symmetry with one 3-fold and three pseudo 2-fold axes. The outer diameter of the cage was 55–60 Å (Fig. 3B). Interestingly, a cluster consisting of fifteen Zn2+ and seven sulfate (SO42−) ions was encapsulated in the cage (Fig. 3C, D and S5†). The Zn2+ ions were bridged by the SO42− ions, and the side chains of Asp2, Glu4, Asp5 and Glu8 coordinated to the Zn2+ ions (Fig. S6A–S6E†). Although it is rare to observe SO42− ion-bridged Zn2+ ions in inorganic complexes,21 the Zn2+–SO42− coordination was apparently stabilized in the cyt cb562 cage by fixing the ion positions (Fig. S6†). The Zn2+ ions of the cluster are classified into five coordination patterns (Zn1–Zn5, three Zn ions for each pattern) (Fig. S6A–S6E†). Six additional Zn2+ ions (three Zn6 and three Zn7) were also observed inside the cavity (Fig. S5, S6F and S6G†). However, there was no hydrogen bond or hydrophobic interaction at the interface of the dimers in the crystal structure; thus, evidently the coordination of the amino acid side chains to the Zn2+ ions stabilized the cage structure.
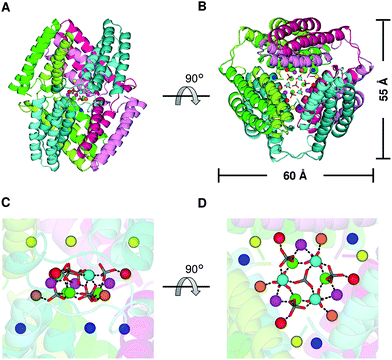 |
| Fig. 3 Cage structure constructed of three domain-swapped cyt cb562 dimers in the crystal (PDB ID: 5AWI): (A and B) overall structure of the cage. (B) is a 90°-rotated view of (A). The three dimers forming the cage are shown in combinations of green and light-green, blue-green and cyan, and red and pink, respectively. The horizontal scale bar (60 Å) corresponds to the distance between the α carbons (Cα) of the Pro56 residues in two protomers shown in green and pink. The vertical scale bar (55 Å) corresponds to the distance between the Cα of Pro53 in a protomer of one dimer (blue–green) and that of Thr96 in a protomer of another dimer (red). (C and D) Enlarged views of the Zn2+ and SO42− ions in the internal cavity. (D) is a 90°-rotated view of (C). The coordination bonds between Zn2+ and SO42− ions are shown as black dashed lines. The Zn2+ ions are shown as green, cyan, magenta, orange, red, yellow and blue spheres. The SO42− ions are shown as stick models, and their sulfur and oxygen atoms are coloured grey and red, respectively. | |
Interaction of dimeric cyt cb562 with Zn2+ ions in solution
To investigate the interaction of dimeric cyt cb562 with Zn2+ ions in solution, dynamic light scattering (DLS) measurements of oxidized dimeric cyt cb562 were performed with an addition of ZnSO4, ZnCl2 and Na2SO4 at pH 5.5, at which the crystal was obtained (Fig. 4A). The mean diameter of the particles became larger for dimeric cyt cb562 with an addition of ZnSO4 (Zn2+ and SO42− ions) (4.6 ± 0.3 nm) compared to that with an addition of ZnCl2 (Zn2+ and Cl− ions) (3.4 ± 0.4 nm) or Na2SO4 (Na+ and SO42− ions) (3.1 ± 0.6 nm), indicating that the dimers interacted among each other in solution in the presence of Zn2+ and SO42− ions, although a defined cage structure was not constructed.
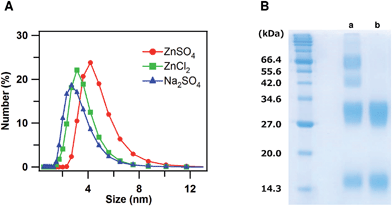 |
| Fig. 4 (A) Size distribution curves of oxidized dimeric cyt cb562 obtained from DLS measurements with an addition of ZnSO4 (circles, red), ZnCl2 (squares, green) or Na2SO4 (triangles, blue). Measurement conditions: sample concentration, 50 μM (heme unit); ZnSO4, ZnCl2, and Na2SO4 concentration, 300 μM; solvent, 15 mM MES buffer; pH 5.5; temperature, 25 °C. (B) SDS-PAGE analysis after the cross-linking reaction of the oxidized dimeric cyt cb562 solution with an addition of ZnSO4 (a) or Na2SO4 (b). Dimeric cyt cb562 (heme unit, 200 μM) was reacted with BS3 (25 mM) after an addition of ZnSO4 or Na2SO4 (1.2 mM) at pH 7.5. | |
We added bis-sulfosuccinimidyl suberate (BS3) to the oxidized dimeric cyt cb562 solution and cross-linked cyt cb562 to investigate the intermolecular interaction of cyt cb562 in solution in more detail. After the reaction of the cyt cb562 dimers with BS3 in the presence of Zn2+ and SO42− ions, the solution was analysed by SDS-PAGE (Fig. 4B, a). Four bands were detected in the SDS-PAGE gel at about 15, 30, 45 and 65 kDa, which corresponded well to the molecular weights of the monomer, dimer, trimer and tetramer, respectively. The dimers may have dissociated to monomers during the cross-linking reaction, resulting in formation of trimers. A broad band was also detected in the SDS-PAGE gel at a molecular weight higher than 100 kDa. When cross-linking the dimer in the presence of Na+ and SO42− ions, only two bands were detected in the SDS-PAGE gel at about 15 and 30 kDa, corresponding to the cyt cb562 monomer and dimer, respectively (Fig. 4B, b). These results showed that cyt cb562 forms oligomers larger than a tetramer in solution in the presence of Zn2+ and SO42− ions, but not in the absence of them.
Discussion
Domain swapping has been observed in various heme proteins.9a,b,f,h,11 In c-type cyts and Mb, the active sites of the domain-swapped oligomers are constructed with two protomers.11 The heme is coordinated with the amino acids from different protomers in dimeric c-type cyts,11a,b,d,e which may stabilize the domain-swapped structure. In cyt cb562, less domain-swapped dimers dissociated to monomers at 4 °C compared to cyt b562 (Fig. S2†). It has been reported that the folding free energy change of oxidized cyt cb562 (K59W, R98C and Y101C cyt b562 mutant) is −42 kJ mol−1, whereas that of oxidized cyt b562 is −30 kJ mol−1.16b The folding free energy change of oxidized HT cyt c552 exhibits a greater negative value than that of oxidized horse cyt c (HT cyt c552, −75 kJ mol−1; horse cyt c, −23 kJ mol−1 (at 25 °C, pH 7)),22 whereas the dissociation temperature of the domain-swapped HT cyt c552 dimer (92 °C)11b is higher than that of the horse cyt c dimer (58 °C).11a Since a domain-swapped dimer shares a similar three-dimensional structure with its monomer, excluding the hinge loop, the folding free energy change of the dimer may correlate with that of the monomer. In fact, dissociation of a domain-swapped dimer to monomers has been suggested to occur via significant unfolding of the polypeptide for many proteins, including RNase A, cyanovirin-N, Stefin A, MPro-C and p13suc1.23 Taking these results into consideration, stabilization of the domain-swapped dimer on dissociation to monomers may correspond to the negative value in the folding free energy change of its monomeric protein.
The ΔH value for the dissociation of the cyt cb562 dimer to monomers was obtained as −13 kcal mol−1 (per dimer) (Fig. 1D). The ΔH values for dimer dissociation varies from negative to positive values in c-type cyt proteins. The ΔH value of the horse cyt c dimer has been reported to be −40 kcal mol−1 (per dimer),11a whereas that of the PA cyt c551 dimer, HT cyt c552 dimer and Aquifex aeolicus cyt c555 dimer are ∼0, +14 and −14 kcal mol−1 (per dimer), respectively.11b,d,e Although many other factors including solvation may affect the ΔH value, the number of hydrogen bonds at the hinge loop decreased in cyt cb562 by the dimerization (dimer, three and six hydrogen bonds for each protomer; monomer, seven hydrogen bonds) (Fig. S7†).24 This decrease in the hydrogen bond number in cyt cb562 by the dimerization may contribute to the negative ΔH value.
The two helices in the N-terminal region (helices 1 and 2) of a protomer and the other two helices in the C-terminal region (helices 3 and 4) of the other protomer interacted between each other in domain-swapped dimeric cyt cb562 (Fig. 2B). Although the heme active site was constructed with two protomers and the axial ligands of the heme originated from different protomers in the dimer, the active site structures were similar between the monomer and dimer (Fig. 2C, D and S4B†). In agreement with the structural results, the absorption spectrum and redox potential were similar between the cyt cb562 monomer and its domain-swapped dimer (Fig. 1A and C). We have previously shown that domain-swapped oligomers of horse cyt c form through intermolecular hydrophobic interaction between the N- and C-terminal α-helices at the early stage of folding.25 Recently, it has been shown by folding simulation that apoMb adopts a similar domain swapping mechanism to that of horse cyt c, and the intermolecular contacts between the helices A–B region of one molecule and the helices G–H region of the another molecule at the early stage of folding result in formation of a domain-swapped dimer.26 In addition, it has been suggested that helices 2 and 3 form first at the initial stage of folding in wild-type apo cyt b562 and its mutant, in which the hydrophobic residues were replaced with Asp and Gly.13c,27 Cyt cb562 were precipitated by the addition of acetic acid (40% (v/v)), and presumably refolded by dissolution of the precipitate with buffer (Fig. S1b†). The present result, where the loop between helices 2 and 3 served as the hinge loop for domain swapping in cyt cb562, is consistent with the previously mentioned feature of helices 2 and 3 forming at the initial folding stage of cyt cb562 monomer. The domain-swapped dimer forms when helices 2 and 3 interact intermolecularly at the initial stage of folding; whereas, the intramolecular interaction of helices 2 and 3 results in formation of monomers (Fig. 5). However, the intermediate complex of cyt cb562 with intermolecular interaction between helices 2 and 3 is likely short-lived, since its folding has been reported to proceed without formation of stable intermediates.16c
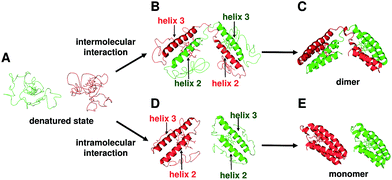 |
| Fig. 5 Schematic view of the dimerization process of cyt cb562 during folding. (A) Unfolded cyt cb562. (B) Dimer intermediate with intermolecular interaction between helices 2 and 3. (C) Dimeric cyt cb562 (PDB ID: 5AWI). (D) Monomer intermediates with intramolecular interaction between helices 2 and 3. (E) Monomeric cyt cb562 (PDB ID: 2BC5). | |
A cage structure of cyt cb562 was formed by three dimers in the crystal (Fig. 3A), although defined cages were not obtained in solution (Fig. 4B). However, the dimeric cyt cb562 crystal was not obtained without Zn2+ ions. The carboxylate groups of Asp (Asp2, Asp5, Asp12 and Asp39) and Glu (Glu4 and Glu8), together with the amino and carbonyl groups of Ala1, were coordinated to Zn2+ ions in the cavity of the domain-swapped cyt cb562 cage (Fig. S6†). The coordination of the amino acids to the Zn2+ ions guided the three cyt cb562 dimers into a cage structure, whereas no hydrogen bond or hydrophobic interaction was detected at the dimer interfaces. It has been reported that crystallization of a protein is promoted by increase in symmetry with the introduction of a Zn2+ coordination site, owing to the strict symmetry imposed on the protein molecule.28 The Zn2+ ions may have enhanced crystallization of the cyt cb562 dimer, where the cage structure of dimeric cyt cb562 exhibited a pseudo-D3 symmetry.
Although protein cages are potentially useful for many applications, such as drug and gene carriers,29 molecular flasks,30 nanomedicines,31 and nanodevices,32 successful protein cage assemblies are limited.4,8f,g Ni and Tezcan reported a cyt cb562 surface mutant crystalline cage, with cage cavity size around 35 Å in diameter, and success in encapsulating a heme peptide fragment inside the cage.17f We obtained a smaller cage constructed with three domain-swapped cyt cb562 dimers encapsulating a Zn–SO4 cluster (fifteen Zn2+ and seven SO42− ions). The cavity size of the domain-swapped cyt cb562 cage was calculated as 1860 Å3 by the program, VOIDOO,33 using a probe radius of 1.4 Å and excluding the Zn2+ and SO42− ions in the model; whereas, it was 32
740 Å3 for the cage structure of the cyt cb562 surface mutant. The interaction of the Zn2+ ions through the SO42− ions may be a factor in the small cavity size of the domain-swapped cyt cb562 cage.
Three Zn2+ ion binding sites have been detected in the cyt cb562 surface mutant cage.17f The Zn2+ ion coordinating non-modified residues (Ala1, Glu8, Asp12 and Asp39) in the surface mutant cage17f also coordinated to the Zn2+ ions in the domain-swapped dimer cage. Especially, the Zn7 site with Ala1, Asp12 and Asp39 of the domain-swapped dimer cage corresponded well to the Zn2+ ion binding site with Ala1 and Asp39 of the surface mutant cage (Fig. S8†). This indicates that N-terminal Ala1 and Asp39 have a tendency to interact together with Zn2+ ions.
Monomeric cyt cb562 did not crystalize under similar conditions that yielded a crystal for the dimer, although the secondary structures of the monomer and dimer were similar (Fig. S4A†). Therefore, the domain-swapped structure may have an important role in construction of the cage structure for dimeric cyt cb562. The amino acid residues in the dimer need to coordinate to the Zn2+ ions at appropriate positions to form the cage structure. Since the two four-helix bundle units were connected by a loop (hinge loop) in dimeric cyt cb562, the relative positions of the two units could be adjusted, whereas the entropy loss on cage formation by three domain-swapped dimers may be smaller than that by six monomers. These properties may have guided the dimers to form a cage structure.
Conclusions
Oligomers were obtained for cyt b562 and cyt cb562 by treatment with acetic acid. Dimeric cyt cb562 was more stable than dimeric cyt b562 at 4 °C, showing that the dimer stability increases by the heme attachment to the protein moiety. According to X-ray analysis, dimeric cyt cb562 exhibited a domain-swapped structure, where the two helices in the N-terminal region (helices 1 and 2) in a protomer and the other two helices in the C-terminal region (helices 3 and 4) of the other protomer interacted between each other. These results were in agreement with the previously mentioned proposal that helices 2 and 3 form at the initial cyt cb562 folding stage. Three dimeric cyt cb562 formed a cage structure containing a Zn–SO4 cluster inside the cavity in the crystal. Although no hydrogen bond or hydrophobic interaction existed at the interfaces of the dimers, coordination of the amino acids of cyt cb562 to Zn2+ ions directed formation of the cage structure. The cavity size of the domain-swapped cyt cb562 cage was smaller than that of the cyt cb562 surface mutant cage, where the interaction of the Zn2+ ions through the SO42− ions was important for maintaining the small domain-swapped cyt cb562 cage. The connection of two four-helix bundle units through the hinge loop in the domain-swapped dimer apparently stabilized the cage structure. These results show that domain swapping may be useful for designing artificial proteins with unique nanostructures, by connecting structural units with a conformation-adjustable loop.
Acknowledgements
We thank Dr Hironari Kamikubo, Nara Institute of Science and Technology, for the use of a DLS analyzer. We are also grateful to Mr Leigh McDowell, Nara Institute of Science and Technology, for his advice on manuscript preparation. The synchrotron radiation experiments were performed at the BL38B1 beamline of SPring-8 with the approval of JASRI (No. 2014A1184; Y. S.). This work was partially supported by Grants-in-Aid for Scientific Research (Category B No. 26288080; S. H.) and Challenging Exploratory Research (No. 15K13744; S. H.) from JSPS.
Notes and references
-
(a) M. J. Boerakker, J. M. Hannink, P. H. Bomans, P. M. Frederik, R. J. Nolte, E. M. Meijer and N. A. Sommerdijk, Angew. Chem., Int. Ed., 2002, 41, 4239 CrossRef CAS;
(b) P. Ringler and G. E. Schulz, Science, 2003, 302, 106 CrossRef CAS PubMed;
(c) H. Kitagishi, Y. Kakikura, H. Yamaguchi, K. Oohora, A. Harada and T. Hayashi, Angew. Chem., Int. Ed., 2009, 48, 1271 CrossRef CAS PubMed;
(d) M. M. C. Bastings, T. F. A. de Greef, J. L. J. van Dongen, M. Merkx and E. W. Meijer, Chem. Sci., 2010, 1, 79 RSC;
(e) K. Oohora, A. Onoda, H. Kitagishi, H. Yamaguchi, A. Harada and T. Hayashi, Chem. Sci., 2011, 2, 1033 RSC;
(f) K. Oohora, S. Burazerovic, A. Onoda, Y. M. Wilson, T. R. Ward and T. Hayashi, Angew. Chem., Int. Ed., 2012, 51, 3818 CrossRef CAS PubMed.
-
(a) E. R. Ballister, A. H. Lai, R. N. Zuckermann, Y. Cheng and J. D. Mougous, Proc. Natl. Acad. Sci. U. S. A., 2008, 105, 3733 CrossRef CAS PubMed;
(b) K. Usui, T. Maki, F. Ito, A. Suenaga, S. Kidoaki, M. Itoh, M. Taiji, T. Matsuda, Y. Hayashizaki and H. Suzuki, Protein Sci., 2009, 18, 960 CrossRef CAS PubMed.
- N. Dotan, D. Arad, F. Frolow and A. Freeman, Angew. Chem., Int. Ed., 1999, 38, 2363 CrossRef CAS PubMed.
-
(a) N. P. King, W. Sheffler, M. R. Sawaya, B. S. Vollmar, J. P. Sumida, I. André, T. Gonen, T. O. Yeates and D. Baker, Science, 2012, 336, 1171 CrossRef CAS PubMed;
(b) N. P. King, J. B. Bale, W. Sheffler, D. E. McNamara, S. Gonen, T. Gonen, T. O. Yeates and D. Baker, Nature, 2014, 510, 103 CrossRef PubMed.
- G. Grigoryan, Y. H. Kim, R. Acharya, K. Axelrod, R. M. Jain, L. Willis, M. Drndic, J. M. Kikkawa and W. F. DeGrado, Science, 2011, 332, 1071 CrossRef CAS PubMed.
-
(a) T. Ueno, T. Koshiyama, T. Tsuruga, T. Goto, S. Kanamaru, F. Arisaka and Y. Watanabe, Angew. Chem., Int. Ed., 2006, 45, 4508 CrossRef CAS PubMed;
(b) J. D. Brodin, X. I. Ambroggio, C. Tang, K. N. Parent, T. S. Baker and F. A. Tezcan, Nat. Chem., 2012, 4, 375 CrossRef CAS PubMed;
(c) Y. Bai, Q. Luo, W. Zhang, L. Miao, J. Xu, H. Li and J. Liu, J. Am. Chem. Soc., 2013, 135, 10966 CrossRef CAS PubMed;
(d) S. Biswas, K. Kinbara, T. Niwa, H. Taguchi, N. Ishii, S. Watanabe, K. Miyata, K. Kataoka and T. Aida, Nat. Chem., 2013, 5, 613 CrossRef CAS PubMed.
- W. B. Zhang, F. Sun, D. A. Tirrell and F. H. Arnold, J. Am. Chem. Soc., 2013, 135, 13988 CrossRef CAS PubMed.
-
(a) J. C. Carlson, S. S. Jena, M. Flenniken, T. F. Chou, R. A. Siegel and C. R. Wagner, J. Am. Chem. Soc., 2006, 128, 7630 CrossRef CAS PubMed;
(b) K. Sugimoto, S. Kanamaru, K. Iwasaki, F. Arisaka and I. Yamashita, Angew. Chem., Int. Ed., 2006, 45, 2725 CrossRef CAS PubMed;
(c) T. F. Chou, C. So, B. R. White, J. C. T. Carlson, M. Sarikaya and C. Wagner, ACS Nano, 2008, 2, 2519 CrossRef CAS PubMed;
(d) D. P. Patterson, A. M. Desai, M. M. B. Holl and E. N. G. Marsh, RSC Adv., 2011, 1, 1004 RSC;
(e) J. C. Sinclair, K. M. Davies, C. Vénien-Bryan and M. E. Noble, Nat. Nanotechnol., 2011, 6, 558 CrossRef CAS PubMed;
(f) Y. T. Lai, K. L. Tsai, M. R. Sawaya, F. J. Asturias and T. O. Yeates, J. Am. Chem. Soc., 2013, 135, 7738 CrossRef CAS PubMed;
(g) Y. L. Lai, E. Reading, G. L. Hura, K. L. Tsai, A. Laganowsky, F. J. Asturias, J. A. Tainer, C. V. Robinson and T. O. Yeates, Nat. Chem., 2014, 6, 1065 CrossRef CAS PubMed.
-
(a) D. Nurizzo, M. C. Silvestrini, M. Mathieu, F. Cutruzzolà, D. Bourgeois, V. Fülöp, J. Hajdu, M. Brunori, M. Tegoni and C. Cambillau, Structure, 1997, 5, 1157 CrossRef CAS PubMed;
(b) B. R. Crane, R. J. Rosenfeld, A. S. Arvai, D. K. Ghosh, S. Ghosh, J. A. Tainer, D. J. Stuehr and E. D. Getzoff, EMBO J., 1999, 18, 6271 CrossRef CAS PubMed;
(c) M. E. Newcomer, Curr. Opin. Struct. Biol., 2002, 12, 48 CrossRef CAS PubMed;
(d) F. Rousseau, J. W. Schymkowitz and L. S. Itzhaki, Structure, 2003, 11, 243 CrossRef CAS PubMed;
(e) M. J. Bennett, M. R. Sawaya and D. Eisenberg, Structure, 2006, 14, 811 CrossRef CAS PubMed;
(f) M. Czjzek, S. Létoffé, C. Wandersman, M. Delepierre, A. Lecroisey and N. Izadi-Pruneyre, J. Mol. Biol., 2007, 365, 1176 CrossRef CAS PubMed;
(g) A. M. Gronenborn, Curr. Opin. Struct. Biol., 2009, 19, 39 CrossRef CAS PubMed;
(h) M. A. Silva, T. G. Lucas, C. A. Salgueiro and C. M. Gomes, PLoS One, 2012, 7, e46328 CrossRef CAS PubMed.
-
(a) M. J. Bennett, S. Choe and D. Eisenberg, Proc. Natl. Acad. Sci. U. S. A., 1994, 91, 3127 CrossRef CAS PubMed;
(b) M. J. Bennett, M. P. Schlunegger and D. Eisenberg, Protein Sci., 1995, 4, 2455 CrossRef CAS PubMed.
-
(a) S. Hirota, Y. Hattori, S. Nagao, M. Taketa, H. Komori, H. Kamikubo, Z. Wang, I. Takahashi, S. Negi, Y. Sugiura, M. Kataoka and Y. Higuchi, Proc. Natl. Acad. Sci. U. S. A., 2010, 107, 12854 CrossRef CAS PubMed;
(b) Y. Hayashi, S. Nagao, H. Osuka, H. Komori, Y. Higuchi and S. Hirota, Biochemistry, 2012, 51, 8608 CrossRef CAS PubMed;
(c) S. Nagao, H. Osuka, T. Yamada, T. Uni, Y. Shomura, K. Imai, Y. Higuchi and S. Hirota, Dalton Trans., 2012, 41, 11378 RSC;
(d) S. Nagao, M. Ueda, H. Osuka, H. Komori, H. Kamikubo, M. Kataoka, Y. Higuchi and S. Hirota, PLoS One, 2015, 10, e0123653 CrossRef PubMed;
(e) M. Yamanaka, S. Nagao, H. Komori, Y. Higuchi and S. Hirota, Protein Sci., 2015, 24, 366 CrossRef CAS PubMed.
- E. Itagaki and L. P. Hager, J. Biol. Chem., 1966, 241, 3687 CAS.
-
(a) M. T. Fisher, Biochemistry, 1991, 30, 10012 CrossRef CAS PubMed;
(b) C. R. Robinson, Y. Liu, J. A. Thomson, J. M. Sturtevant and S. G. Sligar, Biochemistry, 1997, 36, 16141 CrossRef CAS PubMed;
(c) E. J. Fuentes and A. J. Wand, Biochemistry, 1998, 37, 3687 CrossRef CAS PubMed;
(d) C. R. Robinson, Y. Liu, R. O'Brien, S. G. Sligar and J. M. Sturtevant, Protein Sci., 2008, 7, 961 CrossRef PubMed;
(e) P. Wittung-Stafshede, J. C. Lee, J. R. Winkler and H. B. Gray, Proc. Natl. Acad. Sci. U. S. A., 1999, 96, 6587 CrossRef CAS PubMed.
- F. Lederer, A. Glatigny, P. H. Bethge, H. D. Bellamy and F. S. Matthew, J. Mol. Biol., 1981, 148, 427 CrossRef CAS PubMed.
- P. D. Barker, E. P. Nerou, S. M. Freund and I. M. Fearnley, Biochemistry, 1995, 34, 15191 CrossRef CAS PubMed.
-
(a) J. Faraone-Mennella, H. B. Gray and J. R. Winkler, Proc. Natl. Acad. Sci. U. S. A., 2005, 102, 6315 CrossRef CAS PubMed;
(b) J. Faraone-Mennella, F. A. Tezcan, H. B. Gray and J. R. Winkler, Biochemistry, 2006, 45, 10504 CrossRef CAS PubMed;
(c) T. Kimura, J. C. Lee, H. B. Gray and J. R. Winkler, Proc. Natl. Acad. Sci. U. S. A., 2009, 106, 7834 CrossRef CAS PubMed.
-
(a) E. N. Salgado, J. Faraone-Mennella and F. A. Tezcan, J. Am. Chem. Soc., 2007, 129, 13374 CrossRef CAS PubMed;
(b) E. N. Salgado, R. A. Lewis, J. Faraone-Mennella and F. A. Tezcan, J. Am. Chem. Soc., 2008, 130, 6082 CrossRef CAS PubMed;
(c) E. N. Salgado, R. A. Lewis, S. Mossin, A. L. Rheingold and F. A. Tezcan, Inorg. Chem., 2009, 48, 2726 CrossRef CAS PubMed;
(d) R. J. Radford and F. A. Tezcan, J. Am. Chem. Soc., 2009, 131, 9136 CrossRef CAS PubMed;
(e) J. D. Brodin, A. Medina-Morales, T. Ni, E. N. Salgado, X. I. Ambroggio and F. A. Tezcan, J. Am. Chem. Soc., 2010, 132, 8610 CrossRef CAS PubMed;
(f) T. W. Ni and F. A. Tezcan, Angew. Chem., Int. Ed., 2010, 49, 7014 CrossRef CAS PubMed;
(g) R. J. Radford, P. C. Nguyen, T. B. Ditri, J. S. Figueroa and F. A. Tezcan, Inorg. Chem., 2010, 49, 4362 CrossRef CAS PubMed;
(h) E. N. Salgado, X. I. Ambroggio, J. D. Brodin, R. A. Lewis, B. Kuhlman and F. A. Tezcan, Proc. Natl. Acad. Sci. U. S. A., 2010, 107, 1827 CrossRef CAS PubMed;
(i) A. Medina-Morales, A. Perez, J. D. Brodin and F. A. Tezcan, J. Am. Chem. Soc., 2013, 135, 12013 CrossRef CAS PubMed;
(j) W. J. Song and F. A. Tezcan, Science, 2014, 346, 1525 CrossRef CAS PubMed.
- Y. W. Lin, S. Nagao, M. Zhang, Y. Shomura, Y. Higuchi and S. Hirota, Angew. Chem., Int.
Ed., 2015, 54, 511 CAS.
- Y. Q. Feng and S. G. Sligar, Biochemistry, 1991, 30, 10150 CrossRef CAS PubMed.
-
(a) P. D. Barker, J. L. Butler, P. de Oliveira, H. A. O. Hill and N. I. Hunt, Inorg. Chim. Acta, 1996, 252, 71 CrossRef CAS;
(b) Y. Mie, F. Mizutani, T. Uno, C. Yamada, K. Nishiyama and I. Taniguchi, J. Inorg. Biochem., 2005, 99, 1245 CrossRef CAS PubMed.
-
(a) B. Ali, I. G. Dance, D. C. Craig and M. L. Scudder, J. Chem. Soc., Dalton Trans., 1998, 1661 RSC;
(b) Z. Zhang, J.-F. Ma, Y.-Y. Liu, W.-Q. Kan and J. Yang, CrystEngComm, 2013, 15, 2009 RSC;
(c) M. M. Zhang, Y. Gong, P. Zhang, H. F. Shi and J. H. Lin, Dalton Trans., 2014, 43, 17129 RSC.
-
(a) J. Bágel'ová, M. Antalík and Z. Tomori, IUBMB Life, 1997, 43, 891 CrossRef;
(b) S. Uchiyama, A. Ohshima, S. Nakamura, J. Hasegawa, N. Terui, S. J. Takayama, Y. Yamamoto, Y. Sambongi and Y. Kobayashi, J. Am. Chem. Soc., 2004, 126, 14684 CrossRef PubMed.
-
(a) R. Jerala and E. Žerovnik, J. Mol. Biol., 1999, 291, 1079 CrossRef CAS PubMed;
(b) F. Rousseau, J. W. Schymkowitz, H. R. Wilkinson and L. S. Itzhaki, Proc. Natl. Acad. Sci. U. S. A., 2001, 98, 5596 CrossRef CAS PubMed;
(c) E. Bucci, L. Vitagliano, R. Barone, S. Sorrentino, G. D'Alessio and G. Graziano, Biophys. Chem., 2005, 116, 89 CrossRef CAS PubMed;
(d) X. Kang, N. Zhong, P. Zou, S. Zhang, C. Jin and B. Xia, Proc. Natl. Acad. Sci. U. S. A., 2012, 109, 14900 CrossRef CAS PubMed;
(e) L. Liu, I. J. Byeon, I. Bahar and A. M. Gronenborn, J. Am. Chem. Soc., 2012, 134, 4229 CrossRef CAS PubMed.
- The cyt b562 monomer was used for the comparison, since the W59 residue of the K59W mutant cyt cb562 interacted with the hinge loop.
- P. P. Parui, M. S. Deshpande, S. Nagao, H. Kamikubo, H. Komori, Y. Higuchi, M. Kataoka and S. Hirota, Biochemistry, 2013, 52, 8732 CrossRef CAS PubMed.
- K. Ono, M. Ito, S. Hirota and S. Takada, Phys. Chem. Chem. Phys., 2015, 17, 5006 RSC.
- H. Feng, Z. Zhou and Y. Bai, Proc. Natl. Acad. Sci. U. S. A., 2005, 102, 5026 CrossRef CAS PubMed.
- A. Laganowsky, M. Zhao, A. B. Soriaga, M. R. Sawaya, D. Cascio and T. O. Yeates, Protein Sci., 2011, 20, 1876 CrossRef CAS PubMed.
- N. M. Molino and S. W. Wang, Curr. Opin. Biotechnol., 2014, 28, 75 CrossRef CAS PubMed.
- T. Ueno, Chem.–Eur. J., 2013, 19, 9096 CrossRef CAS PubMed.
- D. Peer, J. M. Karp, S. Hong, O. C. Farokhzad, R. Margalit and R. Langer, Nat. Nanotechnol., 2007, 2, 751 CrossRef CAS PubMed.
- I. Yamashita, K. Iwahori and S. Kumagai, Biochim. Biophys. Acta, Gen. Subj., 2010, 1800, 846 CrossRef CAS PubMed.
- G. J. Kleywegt and T. A. Jones, Acta Crystallogr., Sect. D: Biol. Crystallogr., 1994, 50, 178 CrossRef CAS PubMed.
Footnote |
† Electronic supplementary information (ESI) available: Experimental procedure, size exclusion chromatogram, DSC thermogram, protein structure and statistics of data collection. See DOI: 10.1039/c5sc02428e |
|
This journal is © The Royal Society of Chemistry 2015 |
Click here to see how this site uses Cookies. View our privacy policy here.