DOI:
10.1039/C5SC00348B
(Edge Article)
Chem. Sci., 2015,
6, 4530-4536
A simple and effective “capping” approach to readily tune the fluorescence of near-infrared cyanines†
Received
29th January 2015
, Accepted 1st May 2015
First published on 5th May 2015
Abstract
Heptamethine cyanines are favorable for fluorescence imaging applications in biological systems owing to their near-infrared (NIR) absorption and emission. However, it is very difficult to quench the fluorescence of NIR dyes by the classic photoinduced electron transfer mechanism due to their relatively high-lying occupied molecular orbital energy levels. Herein, we present a simple and effective “capping” approach to readily tune the fluorescence of NIR cyanines. The resulting new functional NIR CyBX (X = O, N, or S) dyes not only retain the intact tricarbocyanine scaffold, but also have a built-in switch to regulate the fluorescence by spiro-cyclization. When compared to traditional cyanines, novel CyBX dyes have a superior character in that their NIR optical properties can be readily tuned by the intrinsic spiro-cyclization mechanism. We expect that this “capping” strategy can be extended across not only the visual spectrum but also to structurally distinct fluorophores.
Introduction
In 1856, Williams first discovered cyanine dyes.1 The desirable features of cyanine dyes include narrow absorption bands and high molar absorption coefficients. Members of the cyanines such as monomethine and trimethine cyanines (Cy3) generally display absorption and emission only in the visible region. The extension of the chromophore backbone of cyanine dyes by one vinylene moiety (CH
CH) may lead to a bathochromic shift of ∼100 nm.2 For instance, the maximal emission wavelengths of pentamethine (Cy5) and heptamethine cyanines (Cy7) can extend well into the near-infrared (NIR) region (>650 nm). However, increasing the length of the polymethine chain may elicit unwanted effects including low fluorescence quantum yields, poor photostability, and aggregation.3
With great efforts of the researchers in the field, these limitations have been addressed at least to a certain extent. For example, the introduction of a rigid chlorocyclohexenyl ring in the methine chain was employed to improve the stability and the fluorescence quantum yields of cyanine dyes.4 Maury et al. demonstrated that a small, hard anion could polarize the polymethine chain and therefore enhance the stability of cyanines.5 Blanchard's group reported that conjugation of the cyanine fluorophore Cy5 with a triplet-state quencher could significantly improve the photostability of the dye.6 Pham and co-workers described that tricarbocyanine modified with four water-soluble sulfonate groups could suppress aggregation.7
NIR tricarbocyanine dyes are the most useful members of the cyanine dyes and have been applied as functional fluorescent dyes in diverse fields such as monitoring disease biomarkers, detecting biomolecules, monitoring variations of physiological environments, sensing enzyme activity, studying protein–DNA interactions, and evaluating drug efficacy, all due to the pronounced advantages of the NIR properties.8 Up to date, photoinduced electron transfer (PET) is the main fluorescence mechanism employed for modulating fluorescence of NIR tricarbocyanines (Scheme 1A).8e,f,9 However, it is very difficult to quench the fluorescence of NIR dyes by the PET mechanism due to their relatively high-lying occupied molecular orbital (HOMO) energy levels.10
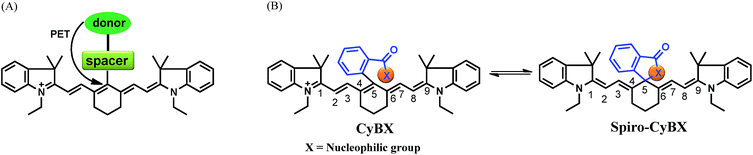 |
| Scheme 1 Design strategy for the new NIR functional CyBX (X = O, N, or S) dyes with an intrinsic spiro-cyclization based mechanism which modulates fluorescence On–Off. (A) The traditional PET mechanism for tuning fluorescence of heptamethine cyanines. (B) The spiro-cyclization based process of the novel functional NIR CyBX dyes described in this work. | |
This has, for a long time, constrained the full potential applications of this important class of NIR fluorescent dyes. Thus, it is crucial to introduce a new strategy to readily modulate the fluorescence of NIR cyanines.
Toward this end, in this contribution, we present a unique means to tune the fluorescence of NIR tricarbocyanines (Scheme 1B). Exemplified by heptamethine cyanine (Cy7), which is a widely used NIR dye, a carboxylic acid moiety (or its amide/thioic derivative) is strategically installed on the carbon 5-position (the central carbon) of the intact cyanine backbone to afford CyBX. We envisioned that CyBX could be transformed into Spiro-CyBX due to nucleophilic attack of heteroatom X on the electrophilic carbon 5. As CyBX essentially maintains the native scaffold of heptamethine cyanine, like heptamethine cyanine it should display absorption and emission in the NIR region. However, the typical “push–pull” characteristic of classic cyanines is disrupted in Spiro-CyBX, we thus anticipated that Spiro-CyBX may show almost no absorption and fluorescence in the NIR region. In other words, the carboxylic acid moiety (or its derivative) may function as a built-in mechanism to readily tune the fluorescence of heptamethine cyanine. Thereby, the transformation of non-fluorescent Spiro-CyBX into fluorescent CyBX could be exploited to design turn-on type NIR fluorescent probes for bioimaging applications in living systems.
Herein, we describe the rational design, synthesis, and optical properties of a new class of cyanines, CyBX NIR functional dyes. We further conducted quantum chemical calculations to shed light on the unique CyBX NIR functional dyes. Finally, to demonstrate the potential use of these innovative types of cyanines, we created two turn-on NIR fluorescent probes based on the Spiro-CyBX platform for imaging pH changes in living cells and Hg2+ in living animals.
Results and discussion
Design and synthesis of CyBX NIR fluorescent dyes
Heteroatoms such as O, N, and S have distinct nucleophilic natures. Thus, their cyclization abilities may significantly vary. Based on the above strategy, we anticipated that CyBO, CyBN, and CyBS compounds (Scheme 2) may have different extents of spiro-cyclization, which could confer diverse optical properties on these compounds. Thus, we expected that these dyes may be used for different situations in living systems.
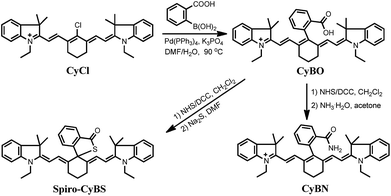 |
| Scheme 2 Synthesis of NIR functional CyBX dyes. | |
The synthesis of the compounds CyBO, CyBN, and CyBS is shown in Scheme 2. The starting compounds CyCl and 2-carboxyphenylboric acid in DMF/H2O were heated under reflux in the presence of Pd(PPh3)4 to afford CyBOvia the Suzuki–Miyaura method.11 This synthetic method is very simple with just one step. In addition, it is modular and versatile, as the carboxylic acid could be easily functionalized to afford its various derivatives. For instance, CyBO was firstly activated with a standard coupling reagent, N-hydroxysuccinimide (NHS), followed by reacting with ammonia or sodium sulfide to provide CyBN and CyBS, respectively. The structures of the CyBX products were fully characterized by 1H NMR, 13C NMR, MS-ESI, and HRMS (ESI†).
Optical properties of CyBX NIR fluorescent dyes
The absorption and emission profiles of CyBX (X = O, N, or S) compounds in different organic solvents (DMF, DMSO, CH3COCH3, CH2Cl2, MeOH, EtOH, and CH3CN) are shown in Fig. S1–S3 (ESI†), and the corresponding photophysical data in DMF are compiled in Table S1 (ESI†). CyBX dyes show maximal absorption and emission at around 770 and 800 nm, respectively, which are well located in the NIR region. Notably, as designed, the shapes of the absorption/emission spectra of CyBX dyes highly resemble those of CyCl (Fig. S4, ESI†), which is in good agreement with the fact that CyBX dyes keep the same intact cyanine backbone as CyCl (Scheme 2). In addition, the photostability of CyBX dyes in DMF was measured by continuous irradiation with a Xe lamp (150 W) with a 10 nm slit width at the maximal absorption wavelength of the CyBX dyes. The results demonstrate that less than 6.4% of the initial fluorescence intensity was decreased after 1 hour of irradiation (Fig. S5†), indicating that these NIR dyes have sufficient photostability for potential biological imaging applications.
We then examined the optical properties of the CyBX dyes in aqueous solution. As expected, they showed drastic differences in absorption intensity in PBS buffer (pH 7.4, 5% DMF) (Fig. 1A). The compound CyBO exhibits strong absorption at around 770 nm, while CyBN has a much smaller absorption. By sharp contrast, CyBS displays almost no absorption. The visual colors of the CyBX dyes (0.3 mM, pH = 7.4, PBS/DMF, v/v = 1
:
1) are consistent with the absorption properties. CyBO, CyBN, and CyBS exhibit green, light green, and pale yellow colours, respectively (Inset of Fig. 1A). CyBO is highly fluorescent, while CyBN displays good fluorescence, and CyBS is essentially non-fluorescent (Fig. 1B). Thus, the fluorescence intensity of these dyes is in the order of CyBO > CyBN > CyBS, in accordance with the trend observed in the absorption. These results may be ascribed to the fact that the nucleophilic abilities of heteroatoms are in the order of S > N > O. Thus, the data suggest that in aqueous buffer; for CyBO, the opened-ring form (CyBO) is predominated; for CyBN, the opened-ring form (CyBN) and the spiro form (Spiro-CyBN) are in equilibrium; for CyBS, the spiro form (Spiro-CyBS) is essentially predominated.
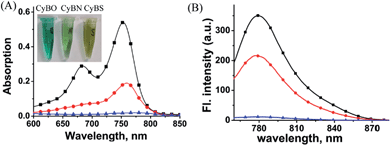 |
| Fig. 1 The absorption (A) and fluorescence (B) spectra of the compounds CyBO ( ), CyBN ( ), and CyBS ( ) (10 μM) in pH = 7.4, PBS/DMF = 95/5. The excitation wavelengths are at 746 nm for CyBO, 745 nm for CyBN, and 748 nm for CyBS, respectively. Inset: (A) the visual colors of the CyBX dyes (left to right: X = O, N, and S) in PBS aqueous solution. | |
To support the above hypothesis, we further investigated the fluorescence profiles of the new NIR dyes at different pH values. As shown in Fig. 2, CyBO shows strong fluorescence over a wide pH range of 4.0–11.0. It is worthy to note that, a gradual drop in the emission with increasing pH is consistent with the hypothesis that the spiro-cyclization ability of the carboxylic acid increases under basic conditions. In the situation of CyBN, a significant decrease in emission with increasing pH is observed. This can be explained by the equilibrium of the ring-opened form (CyBN) and the spiro form (Spiro-CyBN) shifting towards the spiro form (Spiro-CyBN) under basic conditions. In the case of CyBS, it essentially displays no fluorescence over a wide pH range of 4.0–11.0, implying that even under strong acidic conditions, the spiro form (Spiro-CyBS) is still predominated.
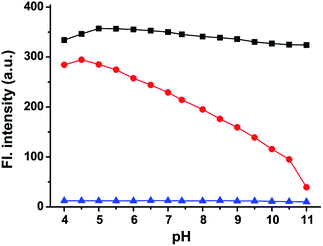 |
| Fig. 2 The maximum emission intensity of the compounds: CyBO ( ), CyBN ( ), and CyBS ( ) (10 μM, pH = 7.4, PBS/DMF = 95/5) at various pH values. The excitation wavelengths are at 746 nm for CyBO, 745 nm for CyBN, and 748 nm for CyBS, respectively. | |
To further confirm the design concept, we also compared both the 1H NMR and 13C NMR of CyBO and CyBS, as these two dyes have different predominant forms. In the case of CyBO, the ring-opened form (CyBO) is essentially predominated, while for CyBS, the spiro form (Spiro-CyBS) is predominated. Thus, we should observe a pronounced distinction between CyBO and CyBS in the NMR spectra. Indeed, the chemical shifts of the protons of CyBS exhibited a marked shift in respect to those of CyBO (Fig. 3A). This is consistent with the hypothesis that the spiro-cyclization of CyBS leads to the disappearance of the positive charge on the nitrogen, which should confer a strong effect on the chemical shifts of the protons. Importantly, the presence of CyBS in the spiro form and CyBO in the ring-opened form is clearly evident in 13C NMR spectra. As shown in Fig. 3B, the key 5-position carbon in CyBO displays a chemical shift at around 140 ppm, in good agreement with its sp2 character. By sharp contrast, the corresponding 5′-position carbon in CyBS has a chemical shift at around 73 ppm, in accordance with its sp3 character due to the spiro-cyclization.
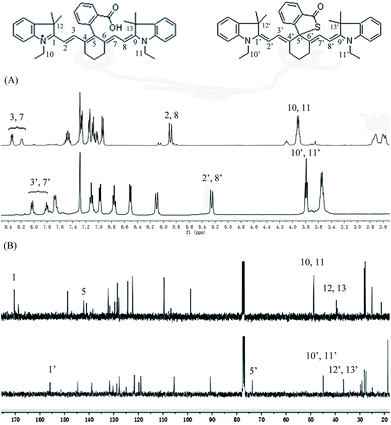 |
| Fig. 3 Comparison of partial 1H NMR (A) and 13C NMR (B) spectra of CyBO (top) and CyBS (bottom) in CDCl3. | |
Density functional theory (DFT) calculations
To shed light on the optical properties of the new functional NIR fluorescent CyBX dyes, DFT calculations with the B3LYP exchange functional employing 6-31G* basis sets using a suite of Gaussian 09 programs were conducted.12 The frontier molecular orbital plots of the CyBX dyes are shown in Fig. 4. The HOMO–LUMO energy gaps of the dyes are very close, consistent with the above findings that these dyes have similar maximal absorption wavelengths (Table S1, ESI†). Furthermore, the DFT optimized structures of the CyBX dyes reveal that the benzoic acid (benzamide, or benzothioic acid) moiety is nearly perpendicular to the heptamethine cyanine core (Fig. S6 and Table S2, ESI†), indicating that the benzoic acid (benzamide, or benzothioic acid) unit at the meso-position has a very minor contribution to the π–π conjugated backbone. This is in good agreement with the observation that the benzoic acid (benzamide or benzothioic acid) unit has almost no contribution to the HOMO–LUMO (Fig. 6). We then calculated the charge distribution of the optimized structures of the CyBX dyes by natural bond orbital (NBO) analysis at the B3LYP level (Fig. S7, ESI†). The representative atomic charges are displayed in Table S3 (ESI†). 1-, 9-position (C
N) and 5-position (central) carbons exhibit a positive charge, while all other carbons on the conjugated backbone show a negative charge. However, considering the three-dimensional structures of the CyBX dyes, only the central (5-position) carbon is likely to be attacked by the nucleophilic heteroatoms (O, N, or S) to form the five-membered spiro form (Spiro-CyBX).
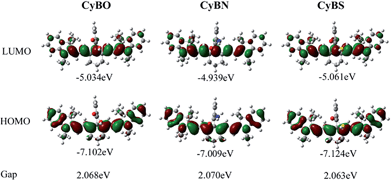 |
| Fig. 4 Molecular orbital plots (LUMO and HOMO) and HOMO–LUMO energy gaps of NIR CyBX (X = O, N, or S) dyes. | |
Development of an innovative NIR fluorescent pH probe for biological imaging
The above studies indicate that, like heptamethine cyanine, the new functional NIR CyBX dyes have both absorption and emission in the NIR region. Furthermore, importantly, CyBX dyes have an intrinsic fluorescence switch by spiro-cyclization. These highly favorable characters imply that CyBX dyes could act as robust platforms for design of NIR fluorescent probes. In addition, the standard MTT assays indicate that CyBX dyes have negligible cytotoxicity to living cells (Fig. S8, ESI†), suggesting that the new compounds are promising for applications in biological systems. In addition, to investigate the cellular uptake of CyBX dyes, we chose CyBO as a representative dye for the time-dependent confocal fluorescence imaging of living cells, up to 120 min (Fig. S9, ESI†). Efficient cellular uptake was observed in the first 30 min of incubation, while the fluorescence was not significantly different after incubating for 30, 60, and 120 min. These data suggest that the cellular uptake of the dye might be via endocytosis.13
Toward this end, we first tested the possibility of CyBN as a novel NIR fluorescent pH probe, as it is sensitive to pH variations as aforementioned (Fig. 2). Intracellular pH (pHi) plays an important role in many biological events including cell proliferation and apoptosis,14 enzymatic activity,15 and ion transport.16 In a typical mammalian cell, the pHi can vary from 4.7 in lysosomes to 8.0 in mitochondria.17 However, abnormal intracellular pH variations are associated with diseases such as Alzheimer's disease and cancer.18 Therefore, monitoring pH changes inside living cells is critical for investigating both physiological and pathological processes. So far, a diverse array of fluorescent pH probes have been developed.8e–g,19 However, only very few of them have both absorption and emission in the NIR region. In addition, most of the reported NIR fluorescent pH probes function by a PET mechanism. By contrast, herein we exploited CyBN as a candidate NIR fluorescent pH probe aiming to demonstrate that the internal fluorescence switch by spiro-cyclization in cyanines could effectively be employed for NIR pH probe development (Scheme 3).
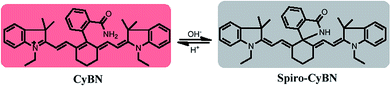 |
| Scheme 3 The ring-opened and spiro form of the NIR probe CyBN under acidic and basic conditions. | |
As shown in Fig. 5A, CyBN (10 μM, in PBS aqueous containing 5% DMF) displays strong fluorescence at pH 4.5. However, an increase in pH induces a gradual decrease of emission, in good agreement with the fluorescence images recorded by an in vivo imaging system (IVIS Lumina XR (IS1241N6071)) (Fig. 5B). The pH-dependence changes in the emission can be rationalized as the fluorescent ring-opened form of CyBN dominates under acidic conditions but the non-fluorescent spiro form dominates under basic conditions. The variations in the absorption profiles further support this explanation (Fig. S10, ESI†).
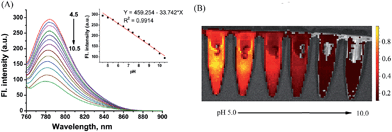 |
| Fig. 5 (A) pH-dependence of the fluorescence spectra of the NIR probe CyBN (10 μM, PBS/DMF: 95/5) upon excitation at 745 nm. Inset: plot of the fluorescence intensity versus pH. (B) The fluorescent images of CyBN (10 μM, DMF/PBS 5/95) at pH 5.0 to 10.0 using the IVIS Lumina XR (IS1241N6071) in vivo imaging system with an excitation filter of 675 nm and an emission range of 760–810 nm. | |
Encouraged by these results, we decided to employ CyBN to monitor real-time changes of pH in living cells. Toward this end, EC109 cells were incubated with the probe (5 μM) at 37 °C for 30 min, and then the cells were washed with pH 7.0 PBS medium (3 × 1 mL). Upon addition of NH4Cl, a weak basic reagent that can be used to increase intracellular pH,20 the variations of the fluorescence intensity of the cells were continuously recorded within 10 minutes (Fig. 6 and S11, ESI†). The results indicate that the dye-stained cells display a real-time drop in emission upon the addition of NH4Cl, which is in good agreement with the pH-dependent changes in the fluorescence spectra of CyBN in aqueous solution (Fig. 5A).
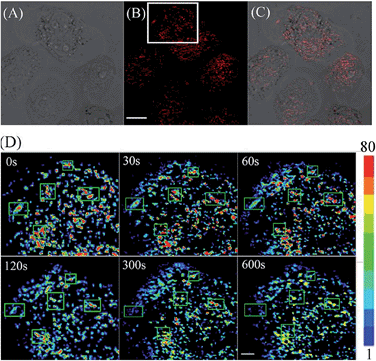 |
| Fig. 6 (A) Brightfield image of EC109 cells stained with CyBN (5 μM) in pH 7.0 PBS solution. (B) Confocal fluorescence image of (A). (C) Overlay of (A) and (B). (D) Followed by addition of 10 mM NH4Cl, and then image (B) was continuously imaged within 10 min. The white box in (B) was enlarged and has a pseudocolor showing the changes of pH with time. The changes of pH were highlighted by green boxes. All the images of fluorescence emission were collected between 770 to 810 nm upon excitation at 635 nm. Scale bar = 10 μm for (A–C) and 5 μm for (D). | |
We further investigated the possibility of CyBN in visualizing endogenous pH changes in an abdominal inflammation model induced by lipopolysaccharides (LPS).21 Two Kunming mice were intraperitoneally (i.p.) injected with LPS to produce an acute inflammatory response, and one of the mice was then injected with CyBN. As shown as in Fig. 7, the emission of the mouse loaded with both LPS and CyBN is stronger than that of the mouse incubated with only the probe CyBN. As a control, the mouse treated with only LPS exhibited almost no fluorescence (Fig. 7a). The fluorescence intensity from the abdominal area of the mice was quantified, and the inflamed mouse treated with CyBN exhibited a higher fluorescence intensity than the normal mouse (Fig. 7D), which suggests a decrease of the pH value in the inflammatory tissues, which is consistent with a previous report.22 Taken together, these results demonstrate that CyBN is useful for in vivo imaging and can detect the pH changes in small animals.
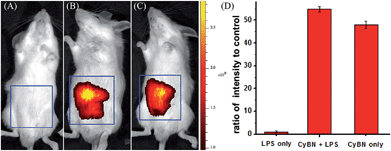 |
| Fig. 7 Representative fluorescence images (pseudocolor) of the mouse injected with CyBN during LPS-mediated inflammatory response in vivo. (A) Only LPS was injected as a control. (B) LPS was injected into the peritoneal cavity of the mice, followed by an injection with CyBN (50 nmol). (C) Only CyBN (50 nmol) was injected. (D) Quantification of the emission intensity from the abdominal area of the mice of the experimental groups relative to the control group. All mice were imaged using the IVIS Lumina XR (IS1241N6071) in vivo imaging system with an excitation filter of 745 nm and an emission range of 760–810 nm. | |
Development of a new NIR fluorescent turn-on Hg2+ probe for biological imaging in living animals
The thiol-affinity to Hg2+ has been exploited to construct fluorescent probes for mercury cations.23CyBS contains a strong nucleophilic benzothioate and shows almost no fluorescence as the spiro form (Spiro-CyBS) dominates. Thus, we envisioned that, upon reacting with Hg2+, the thiol moiety of non-fluorescent Spiro-CyBS may coordinate with Hg2+ resulting in the formation of highly fluorescent CyBS-Hg-CyBS (Scheme 4). In other words, CyBS may exhibit a fluorescence-enhanced signal in the presence of Hg2+.
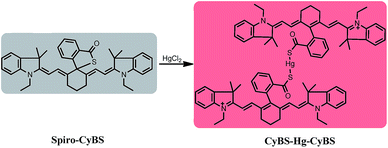 |
| Scheme 4 The likely sensing mechanism of CyBS with Hg2+. | |
As designed, the free probe CyBS is almost non-fluorescent in PBS solution (pH 7.4, 30% MeOH). However, addition of Hg2+ ions elicits a dramatic effect on both the fluorescence and absorption spectra (Fig. 8A and S12, ESI†). A significant fluorescence turn-on response is observed due to the Hg2+-mediated formation of the opened form, and the detection limit was calculated to be 7.27 × 10−7 M (S/N = 3) (Fig. S13, ESI†). Mass spectrometry analysis confirms that the probe coordinates with Hg2+ to form a fluorescent ring-opened form of CyBS-Hg-CyBS (Fig. S14, ESI†), which is consistent with the sensing mechanism reported for benzothioate-based fluorescent Hg2+ probes.23 Furthermore, as displayed in Fig. 8B, the probe is highly selective for Hg2+ over other various relevant metal ions (K+, Na+, Ca2+, Mg2+, Ag+, Al3+, Cr3+, Fe3+, Ni2+, Cu2+, Zn2+, and Cd2+) and reactive oxygen species (ROS) including HClO and H2O2.
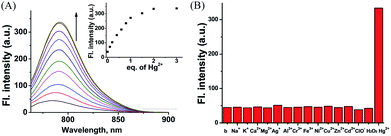 |
| Fig. 8 (A) Fluorescence spectra of CyBS (10 μM) in the presence of various concentrations of Hg2+ (0–30 μM) in phosphate buffer (pH 7.4, 30% MeOH) with excitation at 745 nm. Inset: fluorescence intensity of CyBS at 745 nm vs. Hg2+ concentration (0–3 equivalents). (B) Fluorescence intensity at 792 nm of CyBS (10 μM) with excitation at 745 nm in the presence of various species, such as 200 equivalents of K+, Na+, Ca2+, and Mg2+; 20 equivalents of Ag+, Al3+, Cr3+, Fe3+, Ni2+, Cu2+, Zn2+, and Cd2+; 2 equivalents of ClO−, H2O2, and Hg2+. | |
We proceeded to evaluate the ability of the probe to function in living cells. EC109 cells incubated with CyBS (5 μM) for 30 min at 37 °C provide almost no fluorescence (Fig. 9B). By contrast, the cells incubated with the probe and then Hg2+ had a strong fluorescence (Fig. 9D). These results demonstrate that CyBS is cell membrane permeable and can respond to Hg2+ with a turn-on signal in the living cells.
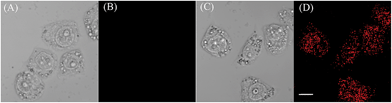 |
| Fig. 9 Images of EC109 cells treated with the probe CyBS in the absence or presence of Hg2+. (A) Brightfield image of EC109 cells incubated with only CyBS (5 μM) for 30 min; (B) fluorescence image of (A); (C) brightfield image of EC109 cells incubated with CyBS (5 μM) for 30 min and further treated with Hg2+ (5 μM) for another 5 min; and (D) fluorescence image of (C). Fluorescence images (B) and (D) were collected between 770 to 810 nm upon excitation at 635 nm. Scale bar = 10 μm. | |
To further demonstrate the potential of the probe for imaging applications in living animals owing to its advantageous NIR absorption and emission, the probe was given to mice in the absence or presence of Hg2+ ions. As shown in Fig. 10B, the control Kunming mouse i.p. injected with only CyBS (50 nmol) had very weak fluorescence. However, followed by i.p. injection with Hg2+ (100 nmol) at the same site, a significant increase of fluorescence intensity was noted (Fig. 10C and D). These results are in good agreement with the data aforementioned in aqueous solution (Fig. 8A) and living cells (Fig. 9). Taken together, these studies indicate that the new NIR probe CyBS can image Hg2+ not only in living cells but also in living animals, demonstrating its value.
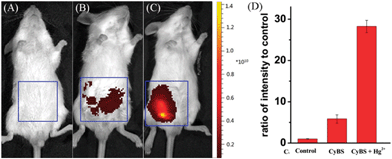 |
| Fig. 10 Representative fluorescence images (pseudocolor) of the mice. (A) Fluorescence image of the negative control, neither CyBS nor Hg2+ was injected; (B) fluorescence image of the mouse treated with only CyBS (50 nmol) for 10 min; (C) fluorescence image of the mouse injected with CyBS (50 nmol) for 10 min, followed by an i.p. injection of Hg2+ (100 nmol); (D) quantification of the emission intensity from the abdominal area of the mice of the experimental groups relative to the control group. All mice were imaged using the IVIS Lumina XR (IS1241N6071) in vivo imaging system with an excitation filter of 745 nm and an emission range of 760–810 nm. | |
Conclusions
In summary, we introduced a simple and effective capping approach to readily tune the fluorescence of NIR cyanines. The unique strategy is based on direct installation of a benzoic acid (benzamide, or benzothioic acid) moiety onto the intact cyanine backbone. The resulting new functional NIR CyBX (X = O, N, or S) dyes not only retain the intact tricarbocyanine scaffold, but also have a built-in switch to regulate fluorescence by spiro-cyclization. Thus, like traditional NIR cyanines, CyBX dyes exhibit both absorption and emission in the NIR region. Furthermore, importantly, CyBX dyes display a unique nature; their NIR optical properties can be readily tuned by the intrinsic spiro-cyclization mechanism. To our best knowledge, such types of NIR cyanines is unprecedented. We further showed that CyBN could be employed to monitor real-time pH changes in living systems and CyBS could be used to detect Hg2+ in both living cells and living animals with a turn-on signal, demonstrating the values of the NIR functional fluorescent CyBX dyes. We expect that the capping strategy can be extended across not only the visual spectrum and but also to structurally distinct fluorophore species. More broadly, the findings described herein suggest the possible development of a series of next-generation functional dyes with optical profiles that are readily tunable. A diverse array of optical probes with such exciting properties would likely find widespread application as powerful molecular tools in studies involving fluorescence microscopy.
Ethical statement
Kunming mice were purchased from Experimental Animal Center of Xiangya School of Medicine Central South University (Changsha, China). All animal procedures for this study were approved by the Animal Ethical Experimentation Committee of Central South University according to the requirements of the National Act on the use of experimental animals (China).
Acknowledgements
Funding was partially provided by NSFC (21172063, 21472067) and the startup fund of University of Jinan.
Notes and references
- C. H. G. Williams, Trans. - R. Soc. Edinburgh, 1856, 21, 377 CrossRef.
- V. W. König, Angew. Chem., 1925, 38, 743 CrossRef PubMed.
-
R. P. Haughland, Molecular probes. Handbook of fluorescent probes and research chemicals, Molecular Probes Inc, Eugene, OR, 9th edn, 2002 Search PubMed.
-
L. Strekowski, Heterocyclic polymethine dyes: synthesis, properties and applications, Springer, Berlin/Heidelberg, 2008 Search PubMed.
- P.-A. Bouit, C. Aronica, L. Toupet, B. L. Guennic, C. Andraud and O. Maury, J. Am. Chem. Soc., 2010, 132, 4328 CrossRef CAS PubMed.
- R. B. Altman, D. S. Terry, Z. Zhou, Q. Zheng, P. Geggier, R. A. Kolster, Y. Zhao, J. A. Javitch, J. D. Warren and S. C. Blanchard, Nat. Methods, 2012, 9, 68 CrossRef CAS PubMed.
- W. Pham, L. Cassell, A. Gillman, D. Koktysh and J. C. Gore, Chem. Commun., 2008, 1895 RSC.
-
(a) W. M. Leevy, S. T. Gammon, H. Jiang, J. R. Johnson, D. J. Maxwell, E. N. Jackson, M. Marquez, D. Piwnica-Worms and B. D. Smith, J. Am. Chem. Soc., 2006, 128, 16476 CrossRef CAS PubMed;
(b) Y. Liu, M. Chen, T. Cao, Y. Sun, C. Li, Q. Liu, T. Yang, L. Yao, W. Feng and F. Li, J. Am. Chem. Soc., 2013, 135, 9869 CrossRef CAS PubMed;
(c) K. Pu, A. J. Shuhendler and J. Rao, Angew. Chem., Int. Ed., 2013, 52, 10325 CrossRef CAS PubMed;
(d) K. Kundu, S. F. Knight, N. Willett, S. Lee, W. R. Taylor and N. Murthy, Angew. Chem., Int. Ed., 2009, 48, 299 CrossRef CAS PubMed;
(e) B. Tang, F. Yu, P. Li, L. Tong, X. Duan, T. Xie and X. Wang, J. Am. Chem. Soc., 2009, 131, 3016 CrossRef CAS PubMed;
(f) R. Tang, H. Lee and S. Achilefu, J. Am. Chem. Soc., 2012, 134, 4545 CrossRef CAS PubMed;
(g) X. Peng, F. Song, E. Lu, Y. Wang, W. Zhou, J. Fan and Y. Gao, J. Am. Chem. Soc., 2005, 127, 4107 Search PubMed;
(h) S. A. Hilderbrand, K. A. Kelly, M. Niedre and R. Weissleder, Bioconjugate Chem., 2008, 19, 1635 CrossRef CAS PubMed;
(i) X. Li, W. Shi, S. Chen, J. Jia, H. Ma and O. S. Wolfbeis, Chem. Commun., 2010, 46, 2560 RSC;
(j) M. Whitney, E. N. Savariar, B. Friedman, R. A. Levin, J. L. Crisp, H. L. Glasgow, R. Lefkowitz, S. R. Adams, P. Steinbach, N. Nashi, Q. T. Nguyen and R. Y. Tsien, Angew. Chem., Int. Ed., 2013, 52, 325 CrossRef CAS PubMed;
(k) S. Zhang, V. Metelev, D. Tabatadze, P. C. Zamecnik and A. J. Bogdanov, Proc. Natl. Acad. Sci. U. S. A., 2008, 105, 4156 CrossRef CAS PubMed;
(l) Z. Yang, J. H. Lee, H. M. Jeon, J. H. Han, N. Park, Y. He, H. Lee, K. S. Hong, C. Kang and J. S. Kim, J. Am. Chem. Soc., 2013, 135, 11657 CrossRef CAS PubMed.
-
(a) X. Wang, L. Cui, N. Zhou, W. Zhu, R. Wang, X. Qian and Y. Xu, Chem. Sci., 2013, 4, 2936 RSC;
(b) S.-Y. Lim, K.-H. Hong, D. I. Kim, H. Kwon and H.-J. Kim, J. Am. Chem. Soc., 2014, 136, 7018 CrossRef CAS PubMed;
(c) K. Xu, M. Qiang, W. Gao, R. Su, N. Li, Y. Gao, Y. Xie, F. Kong and B. Tang, Chem. Sci., 2013, 4, 1079 RSC.
-
(a) D. Rehm and A. Weller, Isr. J. Chem., 1970, 8, 259 CrossRef CAS PubMed;
(b) T. Egawa, K. Hanaoka, Y. Koide, S. Ujita, N. Takahashi, Y. Ikegaya, N. Matsuki, T. Terai, T. Ueno, T. Komatsu and T. Nagano, J. Am. Chem. Soc., 2011, 133, 14157 CrossRef CAS PubMed.
- H. Lee, J. C. Mason and S. Achilefu, J. Org. Chem., 2006, 71, 7862 CrossRef CAS PubMed.
-
M. J. Frisch, et al., GAUSSIAN 09 (Revision A.02), Gaussian, Inc., Pittsburgh, PA, 2009 Search PubMed.
- C. He, K. Lu and W. Lin, J. Am. Chem. Soc., 2015, 136, 12253 CrossRef PubMed.
-
(a) R. A. Gottlieb, H. A. Giesing, J. Y. Zhu, R. L. Engler and B. M. Babior, Proc. Natl. Acad. Sci. U. S. A., 1995, 92, 5965 CrossRef CAS;
(b) R. A. Gottlieb and A. Dosanjh, Proc. Natl. Acad. Sci. U. S. A., 1996, 93, 3587 CrossRef CAS.
- R. T. Kennedy, L. Huang and C. A. Aspinwall, J. Am. Chem. Soc., 1996, 118, 1795 CrossRef CAS.
-
(a) P. Donoso, M. Beltran and C. Hidalgo, Biochemistry, 1996, 35, 13419–13425 CrossRef CAS PubMed;
(b) R. G. W. Anderson and L. Orci, J. Cell Biol., 1988, 106, 539 CrossRef CAS.
- S. Chen, Y. Hong, Y. Liu, J. Liu, C. W. T. Leung, M. Li, R. T. K. Kwok, E. Zhao, J. W. Y. Lam, Y. Yu and B. Z. Tang, J. Am. Chem. Soc., 2013, 135, 4926 CrossRef CAS PubMed.
-
(a) H. Izumi, T. Torigoe, H. Ishiguchi, H. Uramoto, Y. Yoshida, M. Tanabe, T. Ise, T. Murakami, T. Yoshida, M. Nomoto and K. Kohno, Cancer Treat. Rev., 2003, 29, 541 CrossRef CAS;
(b) R. J. Gillies, N. Raghunand, M. L. Garcia-Martin and R. A. Gatenby, IEEE Eng. Med. Biol. Mag., 2004, 57 CrossRef.
-
(a) J. Han and K. Burgess, Chem. Rev., 2010, 110, 2709 CrossRef CAS PubMed;
(b) Q. Wan, S. Chen, W. Shi, L. Li and H. Ma, Angew. Chem., Int. Ed., 2014, 53, 10916 CrossRef CAS PubMed;
(c) J. Han, A. Loudet, R. Barhoumi, R. C. Burghardt and K. Burgess, J. Am. Chem. Soc., 2009, 131, 1642 CrossRef CAS PubMed;
(d) G. Li, D. Zhu, L. Xue and H. Jiang, Org. Lett., 2013, 15, 5020 CrossRef CAS PubMed;
(e) A. M. Dennis, W. J. Rhee, D. Sotto, S. N. Dublin and G. Bao, ACS Nano, 2012, 6, 2917 CrossRef CAS PubMed.
-
(a) A. Roos and W. F. Boron, Physiol. Rev., 1981, 61, 296 CAS;
(b) M. Tantama, Y. P. Hung and G. Yellen, J. Am. Chem. Soc., 2011, 133, 10034 CrossRef CAS PubMed.
-
(a) P. Li, H. Xiao, Y. Cheng, W. Zhang, F. Huang, W. Zhang, H. Wang and B. Tang, Chem. Commun., 2014, 50, 7184 RSC;
(b) M. S. Trent, C. M. Stead, A. X. Tran and J. A. Hankins, J. Endotoxin Res., 2006, 12, 205 CrossRef CAS PubMed.
-
(a) A. Lardner, J. Leukocyte Biol., 2001, 69, 522 CAS;
(b) F. Okajima, Cell. Signalling, 2013, 25, 2263 CrossRef CAS PubMed;
(c) S. Schreml, R. J. Meier, O. S. Wolfbeis, M. Landthaler, R.-M. Szeimies and P. Babilas, Proc. Natl. Acad. Sci. U. S. A., 2011, 108, 2432 CrossRef CAS PubMed.
-
(a) W. Shi and H. Ma, Chem. Commun., 2008, 1856 RSC;
(b) X.-Q. Zhan, Z.-H. Qian, H. Zheng, B.-Y. Su, Z. Lan and J.-G. Xu, Chem. Commun., 2008, 1859 RSC.
Footnote |
† Electronic supplementary information (ESI) available: Experimental procedures, characterization data, and additional spectra. See DOI: 10.1039/c5sc00348b |
|
This journal is © The Royal Society of Chemistry 2015 |
Click here to see how this site uses Cookies. View our privacy policy here.