DOI:
10.1039/C5RA20556E
(Paper)
RSC Adv., 2015,
5, 100750-100761
Biogenic nano-particulate iron-sulfide produced through sulfate and Fe(III)-(hydr)oxide reductions was enhanced by pyruvate as the electron donor†
Received
4th October 2015
, Accepted 18th November 2015
First published on 19th November 2015
Abstract
In nature, the formation of iron sulfide solids is mainly attributed to reductions of sulfate and ferric minerals by microorganisms such as Desulfovibrio vulgaris. In order to evaluate the impacts on microbial activity and optimize iron sulfide production for potential application in uranium remediation, we tested two types of electron donors (lactate and pyruvate) with three synthetic Fe(III) (hydr)oxides (goethite, hematite, and 2-line ferrihydrite). We monitored bacterial metabolism comprehensively, and characterized the biogenic solids using transmission electron microscopy equipped with energy-dispersive X-ray spectroscopy (TEM/EDX), X-ray photoelectron spectroscopy (XPS), X-ray diffraction (XRD), Raman spectroscopy, and mass distribution modeling. Despite similar amorphous FeS production when both e− donors were overdosed, D. vulgaris exhibited distinct patterns of metabolism and other solid production with the two electron donors. Once sulfate reduction was complete, further lactate fermentation was inhibited by accumulation of H2, and thus limited FeS production. In contrast, D. vulgaris utilized all pyruvate by diverting electrons from H2 to formate. In addition, the pH decrease due to the proton release during pyruvate utilization facilitated citrate-induced Fe(III) dissolution and consequently enhanced Fe(III) bioavailability. However, higher pH during lactate utilization and excess soluble Fe(II) during pyruvate utilization led to precipitation of Ca3(PO4)2 and Fe3(PO4)2, respectively. Together, these phenomena resulted in a substantial enhancement of Fe(III)-(hydr)oxide reduction and iron sulfide productivity with pyruvate, though the concentrations of calcium and phosphate need to be controlled to avoid precipitation of other minerals.
1. Introduction
Iron sulfides are naturally occurring minerals derived from either biological processes or hydrothermal activity.1 They can have various degrees of crystallinity and particle sizes, including small nano-crystallites of poorly ordered FeS, larger crystals of mackinawite, and bulk structure of greigite (Fe3S4) or pyrite (FeS2, or iron polysulfide).2–4 Research has revealed that iron sulfides are capable of reducing soluble uranyl ions (UO22+) to insoluble uraninite (UO2) solids,5,6 as well as preventing UO2 from being reoxidized by O2.7,8 This application of iron sulfide nanoparticles has increasingly drawn attention for long-term uranium remediation.7,9,10 Biogenic iron sulfide, with its nanocrystallite nature, is regarded of high redox activity due to the high specific surface area and high surface energy;6 under this scenario, microbial-driven production of iron sulfide could potentially be an effective, economic and sustainable approach for in long-term in situ uranium remediation of contaminated groundwater and sediments.
The source of the sulfide for biogenic iron sulfides typically is sulfate reduction by sulfate-reducing bacteria (SRB). Our previous laboratory-based study11 demonstrated successful production of biogenic iron sulfide nanoparticles through sulfate reduction by D. vulgaris and subsequent precipitation with soluble Fe2+ as the terminal iron source. In nature, to the contrary, the Fe2+ usually comes from bio-reduction of ferric irons carried out by iron-reducing bacteria, including some groups of SRB such as Desulfovibrio spp.12,13 Microbial Fe(III) reduction, especially of Fe(III) (hydr)oxide solids, such as goethite (α-FeOOH), hematite (α-Fe2O3), and ferrihydrite (Fe2O3·0.5H2O), significantly influences the availability of Fe(II) for the subsequent formation of FeS and may affect the characteristics of the FeS formed.14,15
Bio-reduction of Fe(III)-(hydr)oxides can occur by two mechanisms:16 direct enzymatic reductions by electrons derived from electron donors (eqn (1)–(3)) and indirect chemical reductions by biogenic sulfide (eqn (4)–(6)):
|
α-FeOOH + 3H+ + e− → Fe2+ + 2H2O
| (1) |
|
α-Fe2O3 + 6H+ + 2e− → 2Fe2+ + 3H2O
| (2) |
|
Fe2O3·0.5H2O + 6H+ + 2e− → 2Fe2+ + 3.5H2O
| (3) |
|
α-FeOOH + 0.5HS− + 2.5H+ → Fe2+ + 0.5S0 + 2H2O
| (4) |
|
α-Fe2O3 + HS− + 5H+ → 2Fe2+ + S0 + 3H2O
| (5) |
|
Fe2O3·0.5H2O + HS− + 5H+ → 2Fe2+ + S0 + 3.5H2O
| (6) |
The mechanisms and kinetics of enzymatic and chemical reductions of Fe(III) (hydr)oxides have been well studied. For enzymatic reductions, four possible mechanisms (direct contact, formation of electron shuttles, nanowires, and formation of soluble complex ligands) can explain how electrons transfer from the microbes to the solid surface.12,17–19 For chemical reductions by dissolved sulfide, an FeS-complex on the solid surface is rapidly formed prior to Fe(III) reduction coupled to sulfide oxidation, and the subsequent dissolution/detachment of Fe(II) has been identified as the rate-limiting step.20–22
Previous research provides some information on the concurrent reductions of sulfate and Fe(III) by SRB, as well as the solid products. Sani et al.15 demonstrated distinguishable patterns of Fe(III)-(hydr)oxide reduction by Desulfovibrio desulfuricans G-20 for lactate-limiting versus sulfate-limiting conditions. Li et al.23 reported only limited Fe(III) (hydr)oxide reductions by abiotic sulfide alone (5% of total iron for hematite and goethite) or enzymatically alone (less than 6% for all), but enhanced bio-reductions concomitant with abiotic reductions by biogenic sulfide from sulfate reduction by Desulfovibrio desulfuricans G-20 strain (64% for hematite, 74% for goethite, and nearly 100% for ferrihydrite). Herbert et al.24 and Gramp et al.25 found that the predominant biogenic FeS by SRB was mackinawite under most conditions, and its crystallinity was strongly affected by environmental conditions such as temperature, time course, and Fe
:
S ratio. However, little is known about the impacts of different electron donors on the bacteria activities, reduction and solid-production patterns, and the mechanisms behind the phenomena. Gaining understanding of these phenomena will be of especially high value for well-studied Desulfovibrio species, which feature diverse patterns of intracellular electron flow with different electron donors.
The pathways for lactate and pyruvate oxidations coupled with sulfate and Fe(III) reductions are well defined for Desulfovibrio species.26–30 When the starting donor is lactate (CH3CHOHCOO−), it is first partially oxidized to pyruvate (CH3COCOO−) in the periplasm, releasing protons and electrons. Pyruvate is then partially oxidized to acetate (CH3COO−) and CO2, also releasing protons and electrons. These electrons can be used directly for sulfate reduction via respiration, but they also can react with membrane-bound hydrogenases to form molecular hydrogen (H2) via fermentation when sulfate is absent. In addition, formate (CHOO−) can be an alternative to H2 as the fermentation product and electron carrier for periplasmic or extracellular reductions of other substrates, including Fe(III) (hydr)oxide solids.
Despite good knowledge of donor and acceptor catabolism in D. vulgaris, questions remain concerning the formation of biogenic FeS: (1) does supplying a different electron donor (e.g., lactate versus pyruvate) directly lead to different fermentative/oxidative patterns and consequently different patterns for sulfate and iron reductions? (2) Does the type of electron donor affect other culture characteristics and microbial activities indirectly? (3) Do the overall differences affect the chemical or physical characteristics of FeS solids that form? These are the questions we address here by our systematic study of the effects of electron donor (lactate versus pyruvate) coupled with Fe(III) (hydr)oxides as electron acceptors on the metabolism of D. vulgaris and the consequent formation of FeS solids. Our study documents the correlation between the biogenic iron sulfide quality and the electron donor chosen for biostimulation, fills critic gaps of the underlying mechanisms, and thus provides a baseline for the potential application of controllable biogenic FeS in U bioremediations.
2. Experimental
2.1. Strain, growth medium, and culturing conditions
Desulfovibrio vulgaris subsp. vulgaris Postgate and Campbell was purchased from the American Type Culture Collection (ATCC #29579) and grown in 160 mL serum bottles with 100 mL ATCC 1249 medium featuring key species of (in mM) lactate 31.2, sulfate 31.0, citrate 19.4, Fe(II) 3.5, Mg 16.6, Ca 7.3, and 2.9 phosphate. Details of the medium recipe and inoculation/transferring protocol are described in Zhou et al.11
2.2. Iron sources
We synthesized three Fe(III)-(hydr)oxide mineral sources – goethite (α-FeOOH), hematite (α-Fe2O3), and 2-line ferrihydrite (Fe2O3·0.5H2O; ferrihydrite for short) – as described by Schwertmann and Cornell.31 Fig. S1† presents and interprets transmission electron microscope (TEM) images of these three nanoparticulates.
2.3. Medium modification
In all experiments, we modified ATCC 1249 medium by (1) omitting yeast extract; (2) decreasing the sulfate concentration to ∼7.5 mM by replacing MgSO4 with MgCl2, and (3) replacing ferrous ammonium sulfate with a target iron source at the same molar concentration as sulfate. For all experiments, we provided lactate or pyruvate at a concentration greater than the electron equivalency needed for complete reductions of sulfate and Fe(III).
We deoxygenated each modified medium by bubbling N2 gas and then distributed the medium into two sterile 240 mL serum bottles (160 mL per bottle) as duplicates in an anaerobic glove box. The bottles were sealed with rubber stoppers and aluminum caps with a headspace of N2 gas, and then they were autoclaved for 15 minutes at 121 °C. All iron sources other than FeCl2 were added with other components at the beginning. The inocula were from fresh three-day batch cultures, with a dense biomass of 150–200 mg L−1 (in protein), determined by a UV-Visible spectrophotometer with Coomassie (Bradford) reagents. An initial protein concentration of ∼5 ± 1 mg L−1 for all experiments was obtained by injecting 3 mL of the inoculum into each bottle. The inoculated bottles were then transferred immediately to the shaker (200 rpm) and incubated at 30 °C.
2.4. Analytical methods
For routine analyses, we transferred the experimental bottles to the anaerobic glove box and collected 3 mL liquid samples using a sterile syringe. The pH was measured first with an Epoxy Semi-Micro Combination pH Electrode (Beckman Coulter BKA57187) and a pH Meter (Beckman Coulter BKA58734). Samples were then filtered through 0.20 μm membrane filters (Whatman Inc., Haverhill, MA) for other analyses. Concentrations of soluble Fe(III) and total soluble Fe [i.e., soluble Fe(II) + soluble Fe(III)] were analyzed by the colorimetric 5-sulfosalicylic acid (SSA) method32 using a UV-Visible spectrophotometer (Cary 50 Bio, Varian, Inc., Santa Clara, CA) at the wavelengths of 500 nm and 400 nm, respectively. Concentration of soluble Fe(II) was then calculated by subtracting soluble Fe(III) from total soluble Fe. The methods of measuring anions (including sulfate and phosphate) by ion exchange chromatography (IC) and volatile fatty acids (acetate, lactate, pyruvate, formate, and citrate) by high performance liquid chromatography (HPLC) are described in Zhou et al.11
We also measured soluble sulfide in the liquid phase, iron and elemental sulfur in the solid phase at the end of biotic goethite and hematite tests, as well as routinely during the biotic ferrihydrite test and the all abiotic tests. We analyzed the soluble sulfide using EPA Method 3762. We extracted total iron from the unfiltered samples (immediately after vigorous vortex) using 3% hydrochloric acid (HCl) for 24 hours and the measured the iron dissolved in HCl (representing total iron in the original sample) using the iron TNTplus kit (Hach, Loveland, CO). Concentration of total insoluble iron was then calculated by subtracting total soluble iron from total iron. Particularly for quantifying the colloidal Fe solids in the abiotic test, we first settled the bottles on the bench for 5 minutes to exclude the rapidly-precipitating aggregates and then extracted iron from the supernatant only; the turbidly colored supernatant revealed the existence of colloidal Fe(III). We extracted elemental sulfur from the liquid samples using tetrachloroethylene for 24 hours and analyzed the dissolved sulfur using ultra performance liquid chromatography (Waters, Milford, MA, USA) with an ACQUITY UPLC column of 2.1 × 50 mm, 1.7 μm BEH C18 (ref. 33) and an eluent of 95
:
5% methanol
:
water.34
We also measured H2 by collecting 100 μL headspace sample from each bottle with a 500 μL gas-tight syringe (Hamilton Company, Reno, NV), and injecting the gaseous sample into a gas chromatograph (Shimadzu GC-2010) equipped with a thermal conductivity detector (TCD). The standard deviation of the measurements for the H2 pressure was 0.008 atm (0.3 mM), and the detection limit was 0.02 atm (1.0 mM).
2.5. Separation of solids from culture medium
After observing complete lactate consumption and a constant soluble Fe concentration, we placed the serum bottles in the anaerobic glove box for separation and subsequent freeze drying of the solids. The detailed procedures are described in Zhou et al.11 The dry and powdered solids in the serum bottles under anoxic conditions were preserved at −20 °C for further analyses by transmission electron microscope equipped with energy-dispersive X-ray spectroscopy (TEM/EDX), X-ray photoelectron spectroscopy (XPS), X-ray diffraction (XRD), and Raman spectroscopy.
2.6. TEM/EDX analysis
Each powdered sample was loaded on a Lacey carbon 300-mesh copper TEM grid (Ted-Pella, Inc., Redding, CA, USA). TEM images were captured using a Philips CM200-FEG high resolution TEM/STEM (FEI Corp., Eindhoven, The Netherlands) operated at 200 kV, and elemental compositions at selected areas were identified using an EDX detector (EDAX Inc., Mahwah, NJ, USA).
2.7. XPS analysis
We performed XPS on a Vacuum Generators ESCALAB 220i-XL (Thermofisher, USA) with a monochromatic Al Kα source (hν = 1486.6 eV, line width = 0.7 eV for Ag 3d 5/2) at a base pressure of 7 × 10−10 mbar. To minimize the surface oxidation of FeS by oxygen in the atmosphere, the dried solids were sealed in the anoxic serum bottle with a rubber stopper before being transported from the glove box, rapidly wrapped up in the XPS room, and loaded to the XPS sample chamber for analysis under vacuum. We deconvoluted spectra with CasaXPS software.
2.8. XRD analysis
We performed XRD using a Rigaku D/Max-IIB diffractometer with monochromated Cu Kα radiation. The instrument information, analysis procedure, and calculation of average mackinawite crystallite thicknesses were described in Zhou, et al.11 We used the crystallite thickness data to compare the mackinawite size by assuming that (1) the mackinawite crystallite was stoichiometric tetragonal FeS, and (2) polycrystalline particles and microstrain broadening were negligible.35,36
2.9. Raman analysis
We used a thermo scientific DXR Raman spectrometer fitted with a 532 nm laser for collecting the Raman spectra. The laser power was limited to 5 mW to reduce fast oxidation and damage to these iron-containing solid samples.
2.10. Mass-distribution calculations
Mass balances on S and Fe were developed on the basis of eqn (1)–(6) and by assuming that sulfate was reduced completely to sulfide by D. vulgaris and that iron precipitated with S was FeS. This leads to the following mass balances on Fe2+ (eqn (7)), total sulfur (eqn (8)), and sulfide-S (eqn (9)). |
CFe(III) (hydr)oxide,total = [Fe2+] + CFeS + CFe(III) (hydr)oxide,remaining + CFe3(PO4)2·8H2O (vivianite)
| (7) |
|
CS,total = [SO42−]initial = [SO42−]final + CS2−,total + CS0
| (8) |
|
CS2−,total = CS2−,free + CFeS
| (9) |
ESI† describes the step-by-step procedure for computing the distribution of Fe and S species by combining mass-balance eqn (10)–(12) with mass-action equilibrium for reactions in eqn (S1)–(S8).†
3. Results and discussion
3.1. Formate versus H2 as the e− carrier for Fe(III) (hydr)oxide reduction
Fig. 1 and 2 show the patterns of bacterial growth and substrate utilization by D. vulgaris when the electron donor was lactate or pyruvate, respectively, and Table 1 summarizes the environmental conditions measured during incubation. In lactate-stimulated bottles, lactate consumption and corresponding acetate accumulation occurred in parallel with sulfate reduction, and they halted once sulfate was completely reduced (Fig. 1). Consequently, a significant portion of lactate remained in the end. Sulfate reduction rapidly scavenged electrons produced during lactate oxidation; however, in the absence of sulfate, H2 produced during lactate fermentation began to accumulate. Previous studies with D. vulgaris revealed that even a small partial pressure of H2 in the headspace (as low as 0.003–0.015 atm) completely inhibited further fermentation by preventing the membrane-bound hydrogenase from re-oxidizing a quinone electron carrier,29,37–39 unless the presence of syntrophic H2 consumers (e.g., methanogens) to relieve the inhibition by making the fermentation conditions energetically favorable.40,41 This type of H2-based inhibition occurs commonly during fatty acid fermentations by many bacteria, including other Desulfovibrio spp.42,43 In our experiments, Fe(III) (hydr)oxide reductions were not able to scavenge H2 rapidly; as a result, D. vulgaris halted its utilization of lactate upon an accumulation of H2, less than 0.02 atm according to the GC detection limit, which corresponds to only 3.4% e− equivalent from consumed lactate after complete sulfate reduction. On the other hand, formate accumulated until sulfate reduction was complete and then was consumed over 1–2 days (Fig. 1). The maximum accumulating formate accounted for less than 12% of the e− equivalents from consumed lactate in all three bottles. This indicates that, during lactate fermentation in the absence of sulfate, D. vulgaris produced H2 as the primary electron carrier, but also diverted a small portion of electrons to formate.
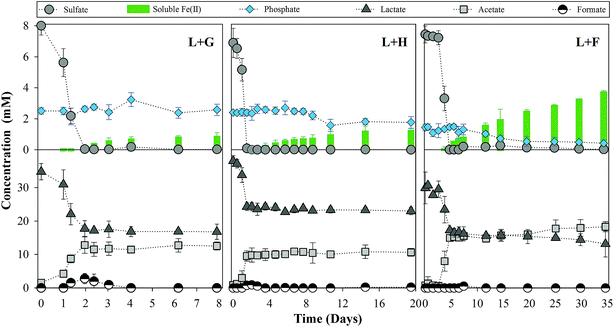 |
| Fig. 1 Concentrations of Fe(II), sulfate, phosphate, lactate, acetate, and formate during the growth of D. vulgaris with lactate as the electron donor and with goethite (“L+G”), hematite (“L+H”), or 2-line ferrihydrite (“L+F”) as the Fe(III) source. Error bars indicate standard deviations of duplicate experiments. | |
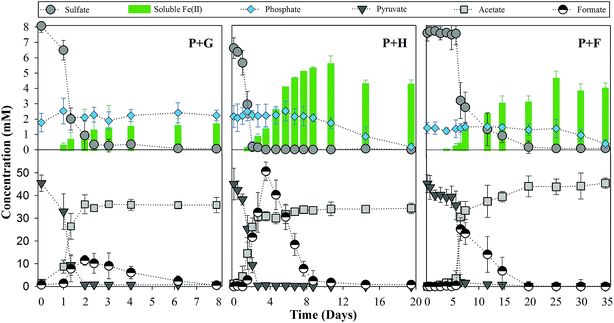 |
| Fig. 2 Concentrations of Fe(II), sulfate, phosphate, pyruvate, acetate, and formate during the growth of D. vulgaris with pyruvate as the electron donor with goethite (“P+G”), hematite (“P+H”), or 2-line ferrihydrite (“P+F”) as the Fe(III) source. Error bars indicate standard deviations of duplicate experiments. | |
Table 1 Measured concentrations of substrates, intermediates, and products; measured pH; experimental durations; and crystallite thicknesses of biogenic mackinawite for all iron-source conditions and when lactate or pyruvate was the electron donor
Iron source |
Electron donor |
Sulfate |
Fe2+ |
Sulfide |
S0 |
Formate |
pH |
Durationa (day) |
Mackinawite thickness (nm) |
Initial (mmol per bottle) |
Final (mmol per bottle) |
Final (mmol per bottle) |
Final (mmol per bottle) |
Final (mmol per bottle) |
Max. (mmol per bottle) |
Initial (s.u.) |
Final (s.u.) |
Counted from the day when the bottles were inoculated to the day when the bottles were opened for solid collection. Calculated on basis of data from TEM images. Calculated on basis of data from XRD spectra. |
Goethite |
Lactate |
1.4 |
0.0 |
0.1 |
0.0 |
0.5 |
0.6 |
7.2 |
7.4 |
6 |
— |
Pyruvate |
1.5 |
0.2 |
0.3 |
0.0 |
0.5 |
2.1 |
7.1 |
6.5 |
6 |
— |
Hematite |
Lactate |
1.2 |
0.0 |
0.2 |
0.0 |
0.0 |
0.2 |
6.9 |
7.6 |
20 |
4.8b |
Pyruvate |
1.2 |
0.0 |
0.8 |
0.0 |
0.0 |
9.1 |
7.0 |
6.6 |
20 |
— |
Ferrihydrite |
Lactate |
1.3 |
0.0 |
0.7 |
0.0 |
0.0 |
0.1 |
7.1 |
8.0 |
43 |
4.9c |
Pyruvate |
1.6 |
0.0 |
0.7 |
0.0 |
0.0 |
4.2 |
7.1 |
7.0 |
43 |
4.2c |
In contrast to the results with lactate, D. vulgaris rapidly consumed all pyruvate before sulfate reduction was completed (Fig. 2), resulting in more acetate accumulation than in the lactate-simulated bottles. The maximum formate concentrations were much higher than in lactate-stimulated bottles, especially in the hematite bottles (H-P; almost 100% of the e− equivalents from consumed pyruvate went to formate) and the ferrihydrite bottles (F-P; 64% to formate). In these two bottles, formate was the primary electron carrier according to the stoichiometry. This difference in formate generation indicates that D. vulgaris was capable of extensively altering the distribution of H2 versus formate production from exogenic pyruvate. By diverting electrons from H2 to formate, pyruvate fermentation avoided the inhibition present with lactate. The formate that accumulated in the liquid became an electron reservoir available for the slower electron-accepting processes associated with Fe(III) (hydr)oxides. The phenomenon was similar to the utilization of propionate as another source of electron reservoir for sulfate and Fe(III) oxide reductions recently observed in a mixed culture.44
3.2. Enzymatic versus nonenzymatic reductions of Fe(III) (hydr)oxide solids
Pyruvate was much more effective than lactate for Fe(III) (hydr)oxide reduction. According to the mass distribution calculations in Table 2 and Fig. 3, over 97% of all three Fe(III) (hydr)oxides were reduced when pyruvate was the electron donor, while lactate led to only ∼75% reductions of goethite and ferrihydrite and less than 33% hematite reduction.
Table 2 Mass distributions of iron, sulfur, and solid phases at the end of the experiments using goethite, hematite, or ferrihydrite as the iron-based electron acceptor and lactate or pyruvate as the electron donor
|
Iron source |
Goethite |
Hematite |
Ferrihydrite |
Electron donor |
Lactate |
Pyruvate |
Lactate |
Pyruvate |
Lactate |
Pyruvate |
The ratio for iron species refers to the percentage of the mole concentration of each iron-containing compound at the end of a test out of the total mole concentration of Fe added as Fe(III) (hydr)oxide at the beginning of the test. The ratio for sulfide species refers to the percentage of the mole concentration of each sulfur-containing compound at the end of a test out of the total mole concentration of S added as sulfate at the beginning of the test. The ratio for the final solids refers to the percentage of the mass of each type of minerals out of the total solid mass collected and dried at the end of each test. |
Iron species (mol mol−1 as %)a |
Fe(III) (hydr)oxide |
20 |
3 |
68 |
3 |
24 |
2 |
Soluble Fe(II) |
11 |
25 |
8 |
28 |
25 |
27 |
Soluble Fe(III) |
0 |
0 |
0 |
0 |
0 |
0 |
FeS(s) |
69 |
72 |
24 |
45 |
51 |
60 |
Vivianite [Fe3(PO4)2·8H2O(s)] |
0 |
0 |
0 |
24 |
0 |
11 |
Sulfur species (mol mol−1 as %)b |
Sulfate |
0 |
0 |
0 |
0 |
0 |
1 |
Soluble sulfide |
0 |
0 |
0 |
1 |
0 |
0 |
FeS(s) |
63 |
64 |
100 |
99 |
100 |
99 |
Elemental sulfur [S0(s)] |
37 |
36 |
0 |
0 |
0 |
0 |
Final solids (g g−1 as %)c |
Fe(III) (hydr)oxide(s) |
19 |
4 |
66 |
3 |
30 |
2 |
FeS(s) |
67 |
82 |
34 |
57 |
70 |
79 |
Elemental sulfur [S0(s)] |
14 |
14 |
0 |
0 |
0 |
0 |
Vivianite [Fe3(PO4)2·8H2O(s)] |
0 |
0 |
0 |
40 |
0 |
19 |
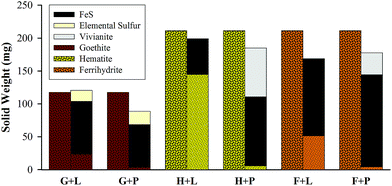 |
| Fig. 3 The mass of Fe(III) hydroxide solids initially added (bars on the left side) and the simulated final solid products (bars on the right side) separated from the experiments identified in the graph. | |
In all experiments, all 7.4 ± 0.5 mM sulfate was reduced to sulfide, with the potential to nonenzymatically reduce the same amount of each Fe(III) (hydr)oxide; thus, the discrepancy of reduced Fe from each Fe(III) (hydr)oxide can be attributed to enzymatic reactions. The considerably lower maximum concentrations of soluble Fe2+ in the lactate-stimulated bottles (Fig. 1) and mass distribution calculations (Table 2) reveal that enzymatic reduction of Fe(III) (hydr)oxides was much less when lactate was the electron donor, and one of the underlying causes was incomplete lactate fermentation, which resulted in less production of formate and H2.
Recent research45 discovered that a small portion (>10%) of thiosulfate, besides the dominant S0, were also produced through abiotic ferrihydrite reduction coupled with sulfide oxidation in presence of the sulfur-reducing bacterium Sulfurospirillum deleyianum, but the mechanisms remain unknown. In all our experiments, thiosulfate concentrations were below detection limit (>0.1% of total initial sulfate); the selection towards thiosulfate or S0 might be driven by distinct S-cycling metabolisms of different bacteria, and further insightful research is needed to address the phenomenon.
3.3. Competition between sulfate and Fe(III) reductions
When lactate was the electron donor, 99% sulfate reduction required less than 2 days in all three bottles. Although a slight amount of Fe(III) (hydr)oxides probably had been enzymatically reduced, soluble Fe2+ did not appear before sulfate reduction was complete. When pyruvate was the electron donor, the appearance of Fe2+ before completion of sulfate reduction documented Fe(III) (hydr)oxide reduction. Furthermore, sulfate reduction was significantly slowed when Fe(II) accumulated. As a result, D. vulgaris required a longer time to reach 99% sulfate reduction coupled with pyruvate than with lactate.
The distinct patterns for the two e− donors probably resulted from different e− transfer patterns between lactate and pyruvate. When sulfate was present, the electrons released from lactate fermentation to pyruvate were transferred for sulfate reduction exclusively, while the electrons from pyruvate fermentation to acetate were proportioned to cytoplasmic sulfate reduction and periplasmic H2/formate formation. With this scenario, sulfate reduction had higher priority than Fe(III) (hydr)oxide reduction when D. vulgaris utilized lactate. This scenario is consistent with the proposed model of electron flow proposed by Keller et al.26 for D. alaskensis G20.
3.4. Fe(III) (hydr)oxide dissolution by citrate
Citrate concentrations were stable during incubations for all the conditions in our D. vulgaris tests (Fig. S2†). This phenomenon, consistent with what earlier research observed,11,46–48 further confirmed that D. vulgaris is not able to utilize citrate as an electron donor and/or carbon source. Rather, citrate in the matrix functioned as (1) a pH buffer to maintain a favorable pH range (6–8) for D. vulgaris, and (2) an iron chelator to prevent iron from precipitation with anions other than sulfide, such as PO43−, OH−, and CO3.2–11,49 Furthermore, previous research reported the abiotic dissolution of Fe(III) (hydr)oxides by citrate, which enhanced subsequent microbial reduction.50–52 The proposed mechanism mainly includes (1) the adsorption of citrate on the solid surface through ligand exchange, which not only weakens the surface structure of the larger-sized aggregates, but also stabilization of smaller-sized colloids from further aggregation; and, (2) the fast and slow detachment/dissolution of Fe(III)-citrate from the large aggregates and small colloids, respectively.52,53 Overall, the dissolution of Fe(III) enhances its bioavailability towards microbial Fe(III) reduction.
In order to understand the contribution of 19.4 mM citrate in the matrix of all our biotic experiments, we conducted a series of abiotic tests featuring the same concentration of citrate for all three Fe(III) (hydr)oxides and both electron donors at three initial pHs: 6, 7, and 8. These tests followed the incubation procedure of the biotic tests inoculated with D. vulgaris. Fig. 4 shows the final iron speciation after the 30 day period. During the tests, we observed buildup of significant soluble Fe(III) (from 19% in L+G to 43% in 43% in L+H) only when the initial pH was 6. This observation is in accord with previous research reporting faster dissolution under acidic circumstances,50,52 probably due to that the reaction of citrate adsorption by substituting citrate ions for hydroxide ions was driven and controlled by the initial hydroxide concentration.
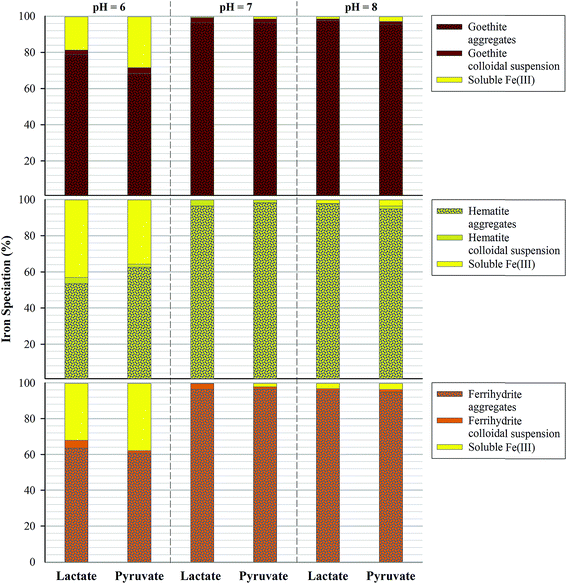 |
| Fig. 4 The final iron speciation after 30 day abiotic tests with citrate. | |
According to stoichiometry, lactate oxidation coupled with sulfate and Fe(III) reductions consumes protons, resulting in a pH increase, while pyruvate fermentation, in contrast, produces protons and thus decreases the pH. Consistently in our biotic tests, the pH was above 7 in all lactate-stimulated bottles, but was below 7 after a few days in all pyruvate-stimulated bottles, and even reached as low as 6.3 in the P+H bottles (Fig. S3†). Thus, in the pyruvate-stimulated bottles, citrate more effectively dissolved Fe(III), and the consequent enhancement of Fe(III) bioavailability, together with the complete utilization of pyruvate via formate, led to distinguishably better Fe(III) reduction (Table 2).
3.5. FeS production and characteristics
When lactate was the electron donor, D. vulgaris only reduced 32% of the hematite. As a result, FeS solids produced from hematite were least abundant (0.6 mmol per bottle, 24% of the total iron), compared to goethite (0.9 mmol per bottle, 69% of the total iron) and ferrihydrite (1.5 mmol per bottle, 51% of the total iron). EDX analyses (Fig. 5) further confirm this trend: solids from the H-L bottles had a lower S
:
Fe signal ratio than solids from other two bottles.
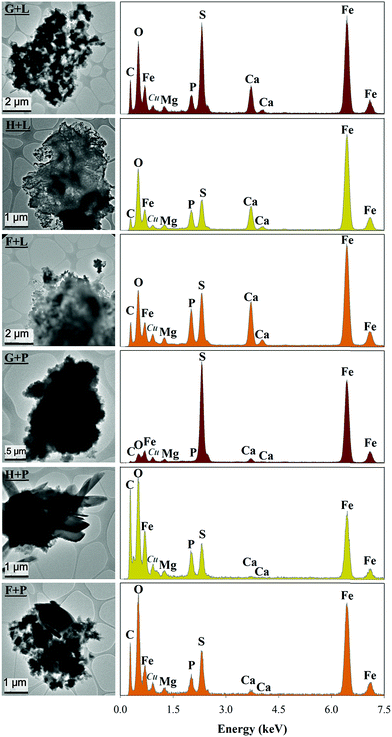 |
| Fig. 5 TEM images (left column) and EDX spectra (right column) of solids from the experiments identified in the TEM image. | |
When pyruvate was the electron donor, D. vulgaris reduced 97% of the Fe(III) (hydr)oxides and consequently produced more FeS than with lactate (Table 2; Fig. 3). This difference was clearly confirmed by the high S
:
Fe signal ratio in the EDX spectrum of the solids from the G+P bottles (Fig. 5). For the other two bottles, however, the S
:
Fe signal ratio was lower (Fig. 5) because more soluble Fe2+ precipitated as vivianite [Fe3(PO4)2·8(H2O)], which is discussed below.
Existence of FeS at the solid surface was confirmed in the XPS spectra by the special tiny humps on the Fe 2p 2/3 peak at 707.5–707.6 eV representing the Fe(II)–S bond,54,55 but only explicit in the samples from the pyruvate experiments (Fig. 6). The apparently higher FeS production with pyruvate probably can be attributed to the stronger XPS signals from the FeS produced with lactate.
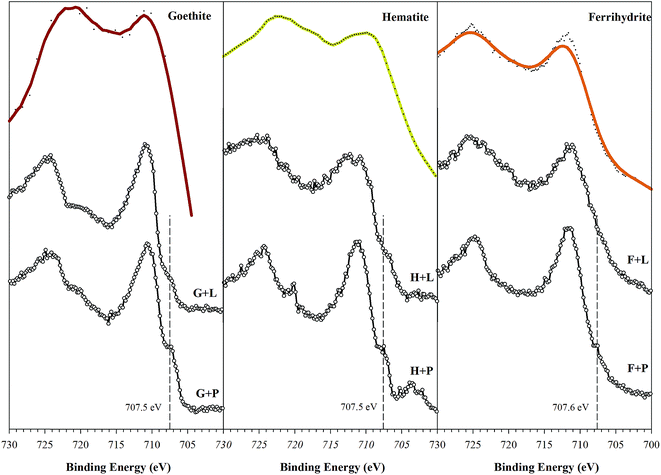 |
| Fig. 6 XPS spectra of synthetic Fe(III) (hydr)oxides and biogenic solids separated from the experiments, all identified in the graph. | |
Crystalline mackinawite was clearly present as a solid product from the two ferrihydrite-containing bottles (F+L and F+P), but was difficult to recognize in the solid products from other bottles by XRD (Fig. 7). TEM imaging did not allow us to examine lattice fringes for most of the solid samples on <20 nm length scales due to severe damage to sulfur and organic matter (Fig. S4†) due to the high energy of the electron beams for magnifications over 140
000×.56,57 We were able to observe lattice fringes only in a limited area of the solid sample from the H+L and G+P bottles (Fig. S5†).
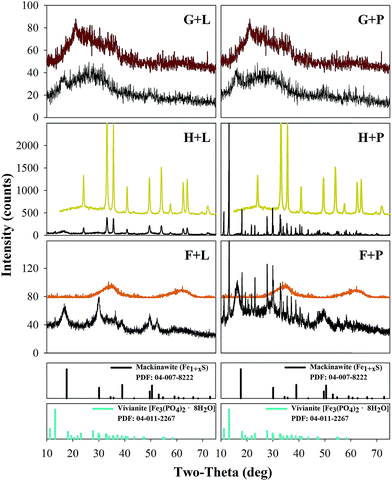 |
| Fig. 7 XRD spectra of synthetic goethite (top lines in G-L and G-P), hematite (top lines in H-L and H-P), 2-line ferrihydrite (top lines in F-L and F-P), and solids (bottom lines) separated from the experiments identified in the graph. | |
In the H+L samples, higher average spacing (5.3 ± 0.1 Å) corresponded to d-spacing of (001) planes of mackinawite, while smaller average spacing (3.3 ± 0.5 Å) corresponded to d-spacing of (101) planes of mackinawite.58–60 The thickness of individual crystals calculated by multiplying the number of (001) fringes by the average d-spacing was 4.8 nm. This value is very close to the thickness of mackinawite from the F+L bottles (4.9 nm; Table 1) calculated from eqn (2) on basis of the XRD data.
In the G+P samples, TEM detected lattice fringes at two nearby locations (Fig. S5†). In the brighter area on the left, the average d-spacing value of 3.0 ± 0.5 Å may indicate the presence of mackinawite. In the darker area on the right, the average d-spacing values (4.2 ± 0.2 Å and 2.7 ± 0.1 Å) were close to the d-spacing values of common goethite (4.18 Å and 2.69 Å).31
The detectable FeS solids were either in amorphous states or nano-crystalline mackinawite, suggesting a retarded crystallization process, probably due to depletion of sulfide, as reported by previous research.24,61,62
3.6. Presence of elemental sulfur
Elemental sulfur, produced via chemical Fe(III) reduction by sulfide, was expected to be present in the final solids from all the bottles. The chemical analyses (Table 1) and the mass-distribution calculations (Table 2) reveal that S0 accounted for a significant fraction of the original sulfate-S in the solids from the G+L and G+P bottles (37% and 36%, respectively), but were absent in other bottles. The routine analyses of sulfide and S0 in ferrihydrite bottles (Fig. S6†) revealed S0 accumulation followed by its consumption. This phenomenon is consistent with previous research63 that demonstrated slow S0 reduction by the pure cytochrome c3 extracted from Desulfovibrio species. In addition, although D. vulgaris did not grow with elemental sulfur as a respiratory electron acceptor, sulfide was formed from elemental sulfur to a limited extent.64,65 The limited and slow process allowed us to observe the presence of S0 in the solids from only the goethite test, which had a shorter duration than the hematite and ferrihydrite tests. Overall, the appearance of S0 further confirmed the chemical reduction of Fe(III) (hydr)oxides by sulfide.
Raman spectra of G+L and G+P solids (Fig. 8) show three prominent peaks at 154, 219, and 473 cm−1 corresponding to elemental sulfur (S08).66 XRD did not detect crystalline S0 in G+L and G+P solids, probably due to its presence in an amorphous form. Single S0 signal was not detected in any area by EDX either, due to its rapid melting and evaporation (Fig. S4†) caused by the high energy of the electron beams for higher magnifications.56,57
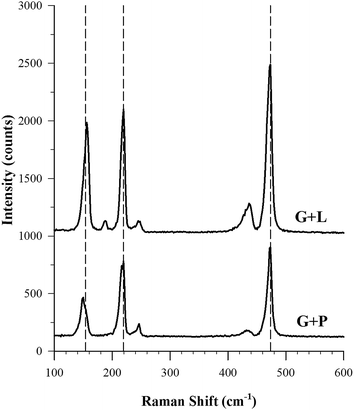 |
| Fig. 8 Raman spectra of the solids from the G+L and G+P experiments. | |
3.7. Calcium-phosphate precipitation
Phosphate uptake into the D. vulgaris biomass was minimal. According to stoichiometry,67 microbial growth through respiration of 7 mol sulfate and/or Fe(III) requires <0.1 mM phosphate; this small change was less than the detection limit of IC. Thus, detectable change of phosphate concentration in the liquid matrix mainly resulted from precipitation with metals.
In our tests, when lactate was the electron donor, the phosphate concentration in the medium was almost constant (2.6 ± 0.3 mM) through the incubation period in the G+L bottle, but gradually dropped by 0.9 ± 0.1 mM after day 8 and day 6 in the H+L and F+L bottles, respectively. Phosphate loss corresponded to pH higher than 7.4 (Fig. S3†). In addition, EDX detected strong signals of calcium (Ca) and phosphorus (P), as well as weaker signals of magnesium (Mg), in all three samples. The presence of P corresponded with phosphate loss in the H+L and F+L bottles. Further EDX scanning in selected areas (Fig. S7†) reveals that Ca, Mg, and P were mostly at the edges, but not in the center of the aggregate.
Previous research68 reported precipitation of Ca5(PO4)3OH at slightly alkaline pH values (normally higher than 7.4). The final pHs in all lactate-stimulated bottles were all above 7.4 (Table 1; Fig. S3†); this allowed calcium and phosphate precipitation, most likely in the form of Ca5(PO4)3OH. In contrast, the final weakly acidic or neutral pH conditions in all three pyruvate-stimulated bottles did not allow Ca5(PO4)3OH precipitation.
XRD scanning did not detect any Ca- or P-associated crystallites in lactate-stimulated bottles (Fig. 7), indicating that the precipitates were still amorphous, in line with the proposed inhibitive effect of Mg on Ca5(PO4)3OH crystallization by substituting in and disrupting the calcium-phosphate crystal lattice or by adsorbing onto the growing calcium phosphate crystals.69,70
Though Ca–Mg–P precipitation was an independent chemical process that did not affect Fe(III) and sulfate reduction, its aggregation on the surface of FeS solids may affect the reactivity of FeS.
3.8. Vivianite formation
When pyruvate was the electron donor, the greater accumulation of soluble Fe2+ from hematite and ferrihydrite was followed by major concentration decreases: 1.3 mM and 0.8 mM, concomitant with 2.0 mM (91%) and 1.0 mM (72%) phosphate loss, respectively. XRD analysis (Fig. 7) confirmed the formation of well-crystallized vivianite, and this explains the high O signals in the EDX spectra (Fig. 5). EDX scanning in selected areas revealed that vivianite had aggregated into large crystals taking a slab (Fig. S8†) or a bullet (Fig. S9†) shape from the H+P and F+P bottles, respectively.
Vivianite is rapidly formed when free ferrous and phosphate ions are present at near-neutral pH.71,72 Previous research73–75 revealed that free sulfide inhibited vivianite formation, but FeS precipitation and Fe(III) (hydr)oxide reductions scavenged sulfide and thus cleared the inhibitive effect in all our experiments. Completion of pyruvate fermentation led to a build-up of soluble Fe2+ high enough (5.5 and 4.6 mM in the H-P and F-P bottles, respectively) to exceed the equilibrium threshold determined by the vivianite solubility product and resulted in precipitation. This is confirmed by the modeling calculation presented in Fig. S10.†
4. Conclusion
In this study, we observed that the distinctly different patterns of electron donor utilization by D. vulgaris significantly affected the amount and type of FeS solids through Fe(III) and sulfate reduction, along with the generation of other solids. When lactate was the electron donor, H2 was the primary electron carrier available for respiring SO42− and Fe(III). Lactate fermentation was inhibited once sulfate reduction was completed, due to a small accumulation of H2. Lacking electrons for further enzymatic reductions of Fe(III) (hydr)oxide, D. vulgaris could not reduce all the Fe(III) (hydr)oxides and, thus, produced limited amounts of FeS when lactate was the fermentable substrate. In contrast, pyruvate enhanced the production of nano-particulate FeS due to (1) electron diversion from inhibitive H2 to non-inhibitive formate to realize its complete utilization, and (2) more proton release during its fermentation to facilitate Fe(III) (hydr)oxide dissolution by citrate as well as to prevent Ca-PO4 precipitation. The only drawback is accumulation of soluble Fe2+ from more hematite or ferrihydrite reduction – due to complete pyruvate fermentation – that led to precipitation of crystalline vivianite [Fe3(PO4)2·8(H2O)]. In summary, pyruvate is a better electron donor and carbon source than lactate for producing large amounts of biogenic mackinawite for potential applications in uranium remediation, but the concentrations of calcium and phosphate need to be controlled to avoid precipitation of other minerals.
Acknowledgements
We express gratitude to the Office of Science, U.S. Department of Energy, Grant No. DE-FG02-09ER64803, for supporting this research. We acknowledge Tara Clancy and Giridhar Upadhyaya at University of Michigan for assistance in establishing the solid washing protocol and growth media conditions for the biogenic production of mackinawite. We also acknowledge Yuqiang Bi at University of Michigan in analyzing a part of samples. We gratefully acknowledge the use of facilities supervised by Thomas Groy at the Department of Chemistry and biochemistry, and by Karl Weiss, Timothy Karcher, and Emmanuel Soignard in the LeRoy Eyring Center for Solid State Science, all at Arizona State University.
References
- V. K. Sharma, J. Filip, R. Zboril and R. S. Varma, Chem. Soc. Rev., 2015, 44(23), 8410–8423 RSC.
- M. A. A. Schoonen, in Sulfur Biogeochemistry: Past and Present, ed. J. P. Amend, K. J. Edwards and T. W. Lyons, Geological Society of America, Boulder, CO, USA, 2004, pp. 117–134 Search PubMed.
- D. Rickard and J. W. Morse, Mar. Chem., 2005, 97, 141–197 CrossRef CAS.
- M. Maurer and B. E. Rittmann, Biodegradation, 2004, 15, 405–417 CrossRef CAS PubMed.
- S. P. Hyun, J. A. Davis, K. Sun and K. F. Hayes, Environ. Sci. Technol., 2012, 46, 3369–3376 CrossRef CAS PubMed.
- H. Veeramani, A. C. Scheinost, N. Monsegue, N. P. Qafoku, R. Kukkadapu, M. Newville, A. Lanzirotti, A. Pruden, M. Murayama and M. F. Hochella, Environ. Sci. Technol., 2013, 47, 2361–2369 CrossRef CAS PubMed.
- A. Abdelouas, W. Lutze and H. E. Nuttall, J. Contam. Hydrol., 1999, 36, 353–375 CrossRef CAS.
- H. S. Moon, J. Komlos and P. R. Jaffe, J. Contam. Hydrol., 2009, 105, 18–27 CrossRef CAS PubMed.
- L. N. Moyes, R. H. Parkman, J. M. Charnock, D. J. Vaughan, F. R. Livens, C. R. Hughes and A. Braithwaite, Environ. Sci. Technol., 2000, 34, 1062–1068 CrossRef CAS.
- Y. Bi, S. Hyun, R. K. Kukkadapu and K. F. Hayes, Geochim. Cosmochim. Acta, 2013, 102, 175–190 CrossRef CAS.
- C. Zhou, R. Vannela, K. F. Hayes and B. E. Rittmann, J. Hazard. Mater., 2014, 272, 28–35 CrossRef CAS PubMed.
- K. A. Weber, L. A. Achenbach and J. D. Coates, Nat. Rev. Microbiol., 2006, 4, 752–764 CrossRef CAS PubMed.
- B. Yan, B. A. Wrenn, S. Basak, P. Biswas and D. E. Giammar, Environ. Sci. Technol., 2008, 42, 6526–6531 CrossRef CAS PubMed.
- J. M. Zachara, J. K. Fredrickson, S. C. Smith and P. L. Gassman, Geochim. Cosmochim. Acta, 2001, 65, 75–93 CrossRef CAS.
- R. K. Sani, B. M. Peyton, J. E. Amonette and G. G. Geesey, Geochim. Cosmochim. Acta, 2004, 68, 2639–2648 CrossRef CAS.
- T. M. Flynn, E. J. O'Loughlin, B. Mishra, T. J. DiChristina and K. M. Kemner, Science, 2014, 344, 1039–1042 CrossRef CAS PubMed.
- D. R. Lovley, J. D. Coates, E. L. BluntHarris, E. J. P. Phillips and J. C. Woodward, Nature, 1996, 382, 445–448 CrossRef CAS.
- G. Reguera, K. D. McCarthy, T. Mehta, J. S. Nicoll, M. T. Tuominen and D. R. Lovley, Nature, 2005, 435, 1098–1101 CrossRef CAS PubMed.
- C. E. Turick, L. S. Tisa and F. Caccavo, Appl. Environ. Microbiol., 2002, 68, 2436–2444 CrossRef CAS PubMed.
- M. D. Afonso and W. Stumm, Langmuir, 1992, 8, 1671–1675 CrossRef.
- S. W. Poulton, M. D. Krom and R. Raiswell, Geochim. Cosmochim. Acta, 2004, 68, 3703–3715 CrossRef CAS.
- S. W. Poulton, Chem. Geol., 2003, 202, 79–94 CrossRef CAS.
- Y. L. Li, H. Vali, J. Yang, T. J. Phelps and C. L. Zhang, Geomicrobiol. J., 2006, 23, 103–117 CrossRef CAS.
- R. B. Herbert, S. G. Benner, A. R. Pratt and D. W. Blowes, Chem. Geol., 1998, 144, 87–97 CrossRef CAS.
- J. P. Gramp, J. M. Bigham, F. S. Jones and O. H. Tuovinen, J. Hazard. Mater., 2010, 175, 1062–1067 CrossRef CAS PubMed.
- K. L. Keller, B. J. Rapp-Giles, E. S. Semkiw, I. Porat, S. D. Brown and J. D. Wall, Appl. Environ. Microbiol., 2014, 80, 855–868 CrossRef PubMed.
- F. O. Morais-Silva, C. I. Santos, R. Rodrigues, I. A. C. Pereira and C. Rodrigues-Pousada, J. Bacteriol., 2013, 195, 4753–4760 CrossRef CAS PubMed.
- J. F. Heidelberg, R. Seshadri, S. A. Haveman, C. L. Hemme, I. T. Paulsen, J. F. Kolonay, J. A. Eisen, N. Ward, B. Methe, L. M. Brinkac, S. C. Daugherty, R. T. Deboy, R. J. Dodson, A. S. Durkin, R. Madupu, W. C. Nelson, S. A. Sullivan, D. Fouts, D. H. Haft, J. Selengut, J. D. Peterson, T. M. Davidsen, N. Zafar, L. W. Zhou, D. Radune, G. Dimitrov, M. Hance, K. Tran, H. Khouri, J. Gill, T. R. Utterback, T. V. Feldblyum, J. D. Wall, G. Voordouw and C. M. Fraser, Nat. Biotechnol., 2004, 22, 554–559 CrossRef CAS PubMed.
- G. Voordouw, J. Bacteriol., 2002, 184, 5903–5911 CrossRef CAS PubMed.
- B.-E. Jugder, J. Welch, K.-F. Aguey-Zinsou and C. P. Marquis, RSC Adv., 2013, 3, 8142–8159 RSC.
- U. Schwertmann and R. M. Cornell, Iron oxides in the laboratory, Verlagsgesellschaft, Weinheim, Germany, 1991 Search PubMed.
- D. G. Karamanev, L. N. Nikolov and V. Mamatarkova, Miner. Eng., 2002, 15, 341–346 CrossRef CAS.
- G. Z. Li, S. Park, D. W. Kang, R. Krajmalnik-Brown and B. E. Rittmann, Environ. Sci. Technol., 2011, 45, 8359–8367 CrossRef CAS PubMed.
- M. M. McGuire and R. J. Hamers, Environ. Sci. Technol., 2000, 34, 4651–4655 CrossRef CAS.
- H. Y. Jeong, J. H. Lee and K. F. Hayes, Geochim. Cosmochim. Acta, 2008, 72, 493–505 CrossRef CAS PubMed.
- A. R. Lennie, S. A. T. Redfern, P. F. Schofield and D. J. Vaughan, Mineral. Mag., 1995, 59, 677–683 CAS.
- I. P. Pankhania, A. M. Spormann, W. A. Hamilton and R. K. Thauer, Arch. Microbiol., 1988, 150, 26–31 CrossRef CAS.
- M. Martins and I. A. C. Pereira, Int. J. Hydrogen Energy, 2013, 38, 12294–12301 CrossRef CAS.
- D. R. Noguera, G. A. Brusseau, B. E. Rittmann and D. A. Stahl, Biotechnol. Bioeng., 1998, 59, 732–746 CrossRef CAS PubMed.
- V. J. Orphan, Curr. Opin. Microbiol., 2009, 12, 231–237 CrossRef CAS PubMed.
- C. B. Walker, Z. He, Z. K. Yang, J. A. Ringbauer, Q. He, J. Zhou, G. Voordouw, J. D. Wall, A. P. Arkin and T. C. Hazen, J. Bacteriol., 2009, 191, 5793–5801 CrossRef CAS PubMed.
- S. Fukuzaki, N. Nishio, M. Shobayashi and S. Nagai, Appl. Environ. Microbiol., 1990, 56, 719–723 CAS.
- S. M. da Silva, J. Voordouw, C. Leitão, M. Martins, G. Voordouw and I. A. C. Pereira, Microbiology, 2013, 159, 1760–1769 CrossRef CAS PubMed.
- M. J. Kwon, M. I. Boyanov, D. A. Antonopoulos, J. M. Brulc, E. R. Johnston, K. A. Skinner, K. M. Kemner and E. J. O'Loughlin, Geochim. Cosmochim. Acta, 2014, 129, 177–190 CrossRef CAS.
- R. Lohmayer, A. Kappler, T. Lösekann-Behrens and B. Planer-Friedrich, Appl. Environ. Microbiol., 2014, 80, 3141–3149 CrossRef PubMed.
- K. Kobayashi and G. W. Skyring, J. Gen. Appl. Microbiol., 1982, 28, 45–54 CrossRef CAS.
- G. Muyzer and A. J. Stams, Nat. Rev. Microbiol., 2008, 6, 441–454 CAS.
- D. Xu and T. Gu, Int. Biodeterior. Biodegrad., 2014, 91, 74–81 CrossRef CAS.
- S. P. Hyun and K. F. Hayes, J. Environ. Eng., 2009, 135, 1009–1014 CrossRef CAS.
- L. Y. Liang, A. Hofmann and B. H. Gu, Geochim. Cosmochim. Acta, 2000, 64, 2027–2037 CrossRef CAS.
- J. Bosch, K. Heister, T. Hofmann and R. U. Meckenstock, Appl. Environ. Microbiol., 2010, 76, 184–189 CrossRef CAS PubMed.
- J. Braunschweig, C. Klier, C. Schroder, M. Handel, J. Bosch, K. U. Totsche and R. U. Meckenstock, Geochim. Cosmochim. Acta, 2014, 139, 434–446 CrossRef CAS.
- G. Furrer and W. Stumm, Geochim. Cosmochim. Acta, 1986, 50, 1847–1860 CrossRef CAS.
- F. Basolo and R. G. Pearson, Mechanisms of inorganic reactions: a study of metal complexes in solution, Wiley, New York, USA, 2nd edn, 1967 Search PubMed.
- C. F. Petre and F. Larachi, AIChE J., 2007, 53, 2170–2187 CrossRef CAS.
- D. A. Young, in Phase diagrams of the elements, University of California Press, Berkeley, Los Angeles, CA, USA, 1991, ch. 10, pp. 129–132 Search PubMed.
- B. Meyer, Chem. Rev., 1976, 76, 367–388 CrossRef CAS.
- H. Ohfuji and D. Rickard, Earth Planet. Sci. Lett., 2006, 241, 227–233 CrossRef CAS.
- M. Wolthers, S. J. van der Gaast and D. Rickard, Am. Mineral., 2003, 88, 2007–2015 CrossRef CAS.
- A. R. Lennie, K. E. R. England and D. J. Vaughan, Am. Mineral., 1995, 80, 960–967 CAS.
- R. T. Wilkin and H. L. Barnes, Geochim. Cosmochim. Acta, 1996, 60, 4167–4179 CrossRef CAS.
- R. T. Wilkin and H. L. Barnes, Geochim. Cosmochim. Acta, 1997, 61, 323–339 CrossRef CAS.
- G. Fauque, D. Herve and J. Legall, Arch. Microbiol., 1979, 121, 261–264 CrossRef CAS PubMed.
- H. Biebl and N. Pfennig, Arch. Microbiol., 1977, 112, 115–117 CrossRef CAS PubMed.
- N. Pfennig and H. Biebl, in The prokaryotes, ed. M. P. Starr, H. Stolp, H. G. Truper, A. Balows and H. G. Schlegel, Springer, Berlin, Germany, 1981, vol. 1, pp. 941–947 Search PubMed.
- R. L. Aggarwal, L. W. Farrar and D. L. Polla, J. Raman Spectrosc., 2011, 42, 461–464 CrossRef CAS.
- B. E. Rittmann and P. L. McCarty, Environmental biotechnology: principles and applications, Tata McGraw-Hill Education, 2012 Search PubMed.
- J. F. Ferguson, D. Jenkins and J. Eastman, J. - Water Pollut.
Control Fed., 1973, 45, 620–631 CAS.
- J. F. Ferguson and P. L. Mccarty, Environ. Sci. Technol., 1971, 5, 534–540 CrossRef CAS.
- C. S. Martens and R. C. Harriss, Geochim. Cosmochim. Acta, 1970, 34, 621–625 CrossRef CAS.
- H. C. B. Hansen and I. F. Poulsen, Clays Clay Miner., 1999, 47, 312–318 Search PubMed.
- A. Zegeye, L. Huguet, M. Abdelmoula, C. Carteret, M. Mullet and F. Jorand, Geochim. Cosmochim. Acta, 2007, 71, 5450–5462 CrossRef CAS.
- J. O. Nriagu, Geochim. Cosmochim. Acta, 1972, 36, 459–470 CrossRef CAS.
- D. Postma, J. Sediment. Petrol., 1977, 47, 1089–1098 CAS.
- G. McGowan and J. Prangnell, Geoarchaeology, 2006, 21, 93–111 CrossRef.
Footnote |
† Electronic supplementary information (ESI) available. See DOI: 10.1039/c5ra20556e |
|
This journal is © The Royal Society of Chemistry 2015 |