DOI:
10.1039/C5RA19255B
(Paper)
RSC Adv., 2015,
5, 90374-90385
Substituted furopyridinediones as novel inhibitors of α-glucosidase†
Received
22nd September 2015
, Accepted 6th October 2015
First published on 8th October 2015
Abstract
The global preponderance of diabetes mellitus has prompted the medical community to opt for various therapeutic solutions to curb this menace. One of the means involved controlling the post prandial hyperglycemia. α-Glucosidase inhibitors are known to be excellent agents for controlling postprandial hyperglycemia. Different classes of α-glucosidase inhibitors have been discovered. In this context, a diverse library of substituted furopyridinediones (12a–v) was designed as potential inhibitors of α-glucosidase, using an intuitive scaffold hopping approach (which was further rationalized by molecular docking). They were synthesized in one step via an aldol condensation reaction of the furopyridinedione scaffold and appropriate aldehydes. The compounds were screened against α-glucosidase using acarbose as the reference inhibitor. Among the screened compounds, 12p transpired to be the lead candidate with an IC50 of 0.24 μM. Lineweaver–Burke analysis of 12p indicated that it is a mixed inhibitor. X-ray crystallography of 12p further confirmed its structure. Molecular modelling studies and molecular dynamic simulation experiments were performed against a homology model of α-glucosidase to observe the binding interaction of 12p with the enzymes.
Introduction
Type I and type II diabetes or diabetes mellitus is one of the most widespread metabolic disorders in the world.1 To date, it has affected nearly 200 million of the global population. It is estimated that this number will swell to ∼400 million by 2030.2 The disease is delineated by hyperglycemia associated with various complications such as cardiac and vascular diseases, thrombosis, cerebral and renal disorders.3
The present therapeutic approaches to treat type II diabetes include antidiabetic oral medicines, viz., α-glucosidase inhibitors and glucosuric thiazolidiene diones.4 Peptide drugs, such as exenatide (glucagen like peptide-1) (GLP-1_agonists) and vildagliptan (dipeptidyl peptidase IV inhibitors), are few of the novel therapies in the market.5 Finally, cannabinoid receptor type I antagonists and bile acid sequestrants are part of emerging therapeutics.6
Controlling postprandial hyperglycemia by delaying the digestion of carbohydrates has been an effective therapeutic approach in type II diabetes.7 In this respect, inhibition of α-glucosidase enzyme slows down carbohydrate absorption. This stabilizes the blood glucose level among diabetic patients, therefore preventing hyperglycemia.
α-Glucosidase belongs to a class of glycoside hydrolase enzymes that release glucose by cleaving the glycosidic bond from the nonreducing terminal of their oligomeric substrate.8 In mammals, it degrades glycogen, assists in internal digestion of dietary carbohydrates and in glycoprotein folding and maturation. α-Glucosidase inhibitors (viz. acarbose) have been established as an effective medicine in type II diabetes.9 They inhibit the membrane bound α-glucosidase at the brush border of the small intestine that reduces digestion of starch and other dietary sugars, which in turn prevents hyperglycemia and maintains a normal blood sugar level.10
Apart from established therapies, a literature study suggested that diverse heterocycles have been discovered as potent α-glucosidase inhibitors. These include natural imino sugars, such as 1,4-dideoxy-1,4-imino-D-arabinitol (DAB) (1) (inhibits yeast α-glucosidase with an IC50 of 0.15 μM) and 2R,5R-dihydroxymethyl-3R,4R-dihydroxypyrrolidine (DMDP) (2) (containing an additional hydroxymethyl group and with a potency of 0.7 μM against yeast α-glucosidase); chalcone glucosides 3a–d, wherein the inhibitory activity against α-glucosidase reduces with the removal of the galloyl and caffeoyl substituents; flavonoids such as 4 (broussochalcone A, a prenylated compound that inhibits yeast α-glucosidase non-competitively with Ki = 5.3 μM); macrocyclic cyclitol 5 that exhibits potent α-glucosidase activity in rats (IC50 rat intestinal maltase = 0.23 μM); radicamin A/B 6a/b, which are alkaloids isolated from plants, also inhibit α-glucosidase in the range of 6.7–9.3 μM; highly active curcumins 7a–c that inhibit α-glucosidase in an IC50 range of 1.6–2.3 μM; and synthetic variants such as azasugar 8, oxindole derivatives 9a–c (inhibits yeast α-glucosidase with an IC50 of 2–14 μM), and terpenes 10 (Fig. 1).11–19
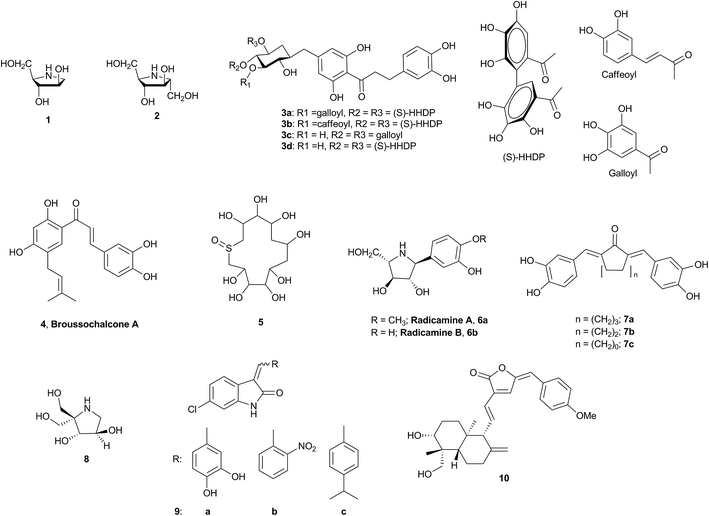 |
| Fig. 1 Representative inhibitors of α-glucosidase (natural and synthetic). | |
In a bid to identify a novel inhibitor of α-glucosidase, a close scrutiny of these natural products and their subsequent comparison with our in-house inventory of scaffolds prompted an intuitive and bioisosteric scaffold hopping exercise based on compounds 7a–c and 9a. It resulted in the selection of compound 12d containing furopyridinedione as the backbone. To rationalize our design, molecular docking revealed strong H-bonding and other nonbonding interactions of the linker and the backbone of 7a and 9a with α-glucosidase (Fig. 2 and Table 1 of ESI 2†) (a homology model was developed [ESI 2†] wherein the stereochemical quality of the model was validated by a Ramachandran plot and more than 99.3% of the residues were in the favored and allowed regions and only 0.7% [6 residues] were in the outlier region [Fig. 1, ESI 2†]). To our extreme gratification, compound 12d also unveiled a similar binding interaction with the protein (Fig. 2 and Table 1 of ESI 2†).
Therefore, with 12d as the reference, a molecular library, including 12a–v, was envisioned as the potential inhibitors of α-glucosidase. The general design of the compounds comprised furopyridinedione (13) attached to an aromatic or heteroaromatic moiety through a double bond (Fig. 2).
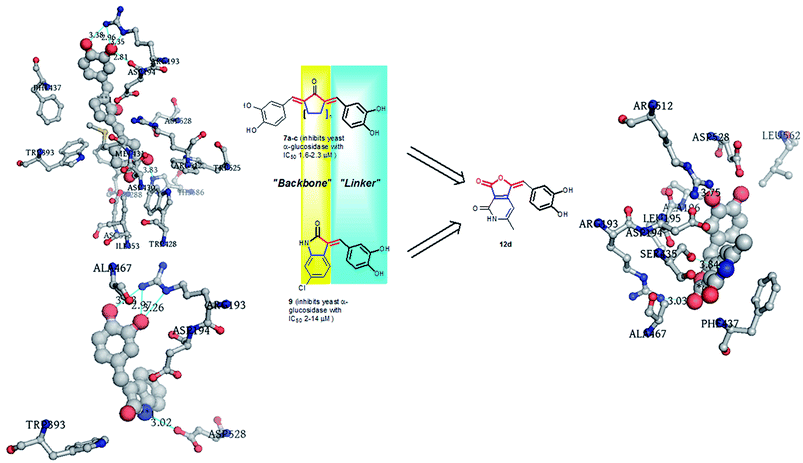 |
| Fig. 2 Design of the furopyridinedione molecules (12a–v) from scaffold hopping and bioisosteric substitution of 7a and 9a. | |
Results and discussion
Chemistry
The target library (12a–v) was synthesized as depicted in Scheme 1. The reaction involved aldol condensation of furopyridinedione 13 and appropriate aldehydes (14a–p and 15a–f) in the presence of piperidine as base and in ethanol under reflux (80–90 °C). The final compounds precipitated during the course of the reaction and they were filtered and washed with hexane for purification. They were characterized by routine 1H, 13C and LCMS. For the aromatic substituents, in general the yields of the final molecules with electron withdrawing functionalities (12c, h and l) were better (70–92%) compared to their electron donating counterpart, viz., 12a, b, d–f, g, and i–k (35–72%). In addition, the heteroaromatic aldehydes afforded the desired compounds (12l–p) in a moderate yield (62–75%). The 4-substituted thiophene analogs (12q–v) were synthesized from aldehydes 15a–f. Aldehydes 15a–e were synthesized by Suzuki coupling of 4-bromothiophene-2-carbaldehyde with phenyl, 3-pyridyl, 2-methoxy-5-pyridyl, 2-(N-boc-N-piperazinyl)-5-pyridyl, 4-fluorostyryl-boronic acids, catalytic palladium bis triphenyl phosphine chloride, sodium carbonate (as base) and dimethyl formamide (DMF) (as solvent) at 110 °C. Aromatic nucleophilic substitution (SNAr) of 4-bromothiophene-2-carbaldehyde with piperidine afforded 15f.
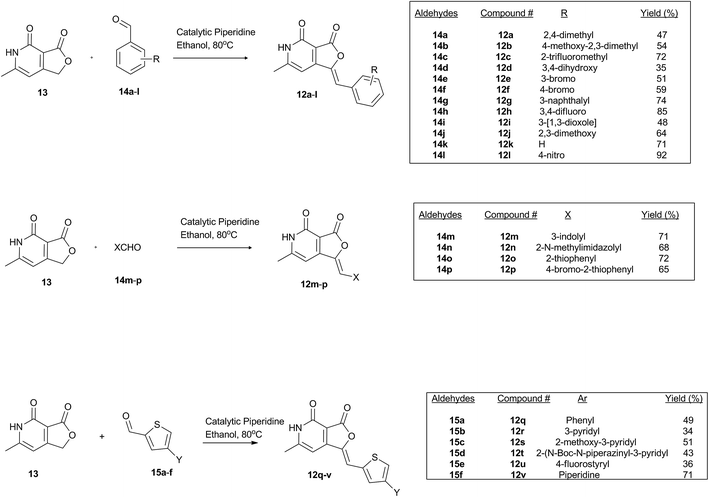 |
| Scheme 1 Synthesis of the library molecules 12a–v. | |
In general, the 1H-spectra of all the final compounds (12a–v) were characterized by a singlet at ∼2.3 ppm that corresponds to the methyl (CH3) on the pyridone moiety and have many signals in the aromatic region between 6.5 and 8.8 ppm. For compound 12t, the densely congregated peaks at the aliphatic region (3–4 ppm) can be attributed to the piperazinyl moiety. Similarly, the peaks at 1.7 and 4 ppm in 12v are because of the piperidine ring. In general, the 13C spectra of these compounds are characterized by the amide and lactone carbonyl at 165 and 160 ppm, respectively.
Pharmacological study
α-Glucosidase inhibition. The library of aryl and heteroaryl furopyridine diones was screened against α-glucosidase (yeast origin) with acarbose as a reference inhibitor. 100 μL of nitrophenol-D-glucopyranoside substrate (2 mM PNP-Glc dissolved in 2 mM phosphate buffer at pH of 7.2) (actual substrate concentration was 0.31 mg mL−1 to 0.93 mg mL−1) along with the test compounds at concentrations ranging from 0.1 to 1 μM and 10 to 50 μM were taken in 96-well plates. Furthermore, the final volume of the reaction mixture was made to 200 μL with 2 mM phosphate buffer (pH 7.2). The hydrolytic reaction commenced by the addition of α-glucosidase enzyme (0.5 mg mL−1) and the plates were incubated at 37 °C for 15 minutes. Finally, the reaction was terminated by adding 50 μL of 2 N Na2CO3. To know the potency of the test compounds, the percentage inhibitions observed were plotted against the concentration using a non-linear regression approach (data not shown), from which the IC50 concentrations were computed.
Structure activity relationship. Based on the results obtained from the α-glucosidase inhibitory activity of the molecules (12a–v), the library was segregated into three segments (Fig. 3). Segment 1 contained furopyridinedione attached to the diversely substituted aromatic groups, segment 2 contained various heteroaromatic groups and segment 3 contained substituted thiophenes. Assessment of these segments illustrated the evolution of the initial structure activity relationship studies directed towards a structure with improved inhibitory activity.
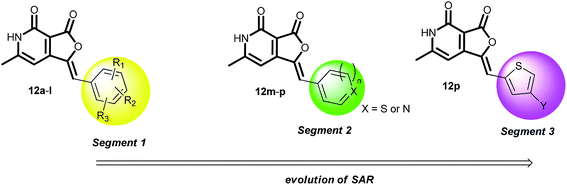 |
| Fig. 3 Structure activity relationship studies. | |
The activity results in Table 1 indicate that quite a few molecules in the library, such as 12d, f, h, l, m, n, p, and q, are more active than the reference molecule acarbose (17.4 μM). Interestingly, the substitution pattern in segment 1 significantly influenced the activity of the compound, and it was found that compounds with electron withdrawing substituents, such as 12c, f, h and l, are significantly more potent (IC50 range 1–21 μM) than their electron donating analogs (IC50 range of 40–54 μM). In general, the segment 2 compounds are more active than the segment 1 compounds. Among them, compound 12p is the leading candidate exhibiting inhibition with an IC50 of 0.24 μM. It contains a thiophene ring substituted with bromide at C4. The chemical reactivity of bromide in 12p is a concern; however, it is too early to comment on this. It is also worth considering that there is abundance of bromide containing drugs in the market such as vasodilator nicergoline, sedative brotizolam, anticancer agent pipobroman, and antiseptic merbromin.20 N-Methyl imidazole substituted 12n is another promising compound with an IC50 of 0.89 ± 0.007 μM. It can be noted that further modification of the C4 bromide of 12p with aryl, alkenyl, heteroaryl and 2°-amines (12q–v) could not improve the activity profile any further. This indicates a very tight SAR in this region, which requires subtle substitutions to improve the activity. In addition, screening of 12p against mammalian COS cell lines indicated that it is nontoxic (refer ESI†).
Table 1 α-Glucosidase inhibition of 12a–va
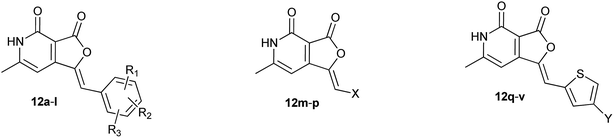
|
Entry |
R1 |
R2 |
R3 |
X |
Y |
α-Glucosidase IC50 (μM) |
(1) NA: no activity was observed; (2) IC50: concentration of inhibition required for half-maximal enzyme inhibition and is reported as means of two independent experiments; (3) the screening assays for finding these IC50 concentrations were performed at pH 7.0 and temperature 37 °C. |
12a |
2-Me |
5-Me |
— |
— |
— |
NA |
12b |
2-Me |
3-Me |
4-OMe |
— |
— |
NA |
12c |
2-CF3 |
— |
— |
— |
— |
23 ± 0.12 |
12d |
3-OH |
4-OH |
— |
— |
— |
2 ± 0.02 |
12e |
3-Br |
— |
— |
— |
— |
41 ± 0.21 |
12f |
4-Br |
— |
— |
— |
— |
10 ± 0.63 |
12g |
2-Napthalyl |
— |
— |
— |
— |
NA |
12h |
3-F |
4-F |
— |
— |
— |
1 ± 0.09 |
12i |
3,4-(O–CH2–O) |
|
|
|
NA |
12j |
2-OMe |
3-OMe |
— |
— |
— |
NA |
12k |
H |
— |
— |
— |
— |
53 ± 0.38 |
12l |
2-NO2 |
— |
— |
— |
— |
3.4 ± 0.55 |
12m |
— |
— |
— |
2-Indolyl |
— |
2 ± 0.15 |
12n |
— |
— |
— |
N-Me-2-imidazolyl |
|
0.9 ± 0.04 |
12o |
— |
— |
— |
2-Thiophenyl |
— |
10 ± 0.30 |
12p |
— |
— |
— |
4-Bromo-2-thiophenyl |
— |
0.24 ± 0.04 |
12q |
— |
— |
— |
— |
Phenyl |
8.3 ± 0.39 |
12r |
— |
— |
— |
— |
3-Pyridyl |
NA |
12s |
— |
— |
— |
— |
2-OMe-3-pyridyl |
30 ± 0.60 |
12t |
— |
— |
— |
— |
N-Boc-2-piperazinyl-3-pyridyl |
86 ± 0.62 |
12u |
— |
— |
— |
— |
4-Fluorostyryl |
NA |
12v |
— |
— |
— |
— |
N-Piperidinyl |
NA |
Acarbose |
— |
— |
— |
— |
— |
17 ± 0.7 |
Reaction kinetics study. Inhibitory kinetic assays were performed to identify the mode of inhibition of the highest effective molecule, 12p, over α-glucosidase. The study was executed by graphical means of employing primary (Lineweaver–Burk) and secondary plots.21 Fig. 4 illustrates the α-glucosidase inhibition kinetic plots and it can be observed that all the data lines on the Lineweaver–Burk plots of 12p intersected in the second quadrant, indicating a mixed type of inhibition over α-glucosidase enzyme. The inhibition mechanism, indeed, involved the binding of this inhibitor to both glucosidase and the glucosidase + substrate complex.22 There is a possibility that 12p binds to an allosteric site of the enzyme, α-glucosidase (yeast origin) and the substrate is bound to its active site. The mixed type of inhibition subsidizes two inhibition constants, viz., K1 and K2 (the observed Km for the employed substrate concentration was 0.53 mg mL−1). The constants were determined from the slope of 2° plots and Y-intercepts from a LB-plot against the concentration of each inhibitor (data not shown in this paper). Therefore, for 12p, the K1 and K2 constants with α-glucosidase were computed as 0.22 ± 0.008 μM and 0.25 ± 0.002 μM. The smaller dissociation constants indicated stronger inhibition by the enzymes.
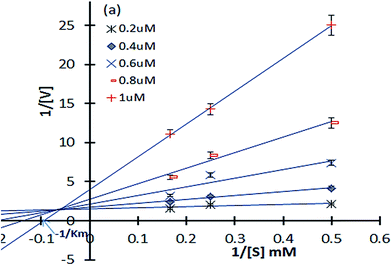 |
| Fig. 4 Double-reciprocal (Lineweaver–Burk) plots of α-glucosidase (yeast) inhibition kinetics exhibited by compound 12p; α-glucosidase (0.5 IU mL−1) was exposed to 12p at 37 °C for 10 min, followed by varying concentrations of 4-nitrophenyl α-D-glucopyranoside (PNPG). | |
X-ray crystallography. To confirm the conformation of compound 12p, we determined its three dimensional structure via a single-crystal X-ray diffraction experiment. The compound 12p was crystallized at room temperature by slow evaporation of its solution in N-methyl-2-pyrrolidone (NMP). Interestingly, the X-ray structure revealed that the compound 12p has co-crystallized with NMP, essentially due to the presence of a strong N–H⋯O hydrogen bond between the N–H of compound 12p and the O
C of NMP (Fig. 5). Both compound 12p and the solvent NMP have a planar geometry and they are found lying almost in the same plane. Both the molecules together pack in the crystal lattice in an anti-parallel fashion and form a zigzag pattern (Fig. 6). In the crystal structure, the strong intermolecular N–H⋯O hydrogen bond and π⋯π stacking with a short inter-planar distance of about 3.5 Å lead to the formation of a three dimensional framework. A crystallization experiment with other solvents and the search of a crystal structure with only the 12p molecule is in progress.
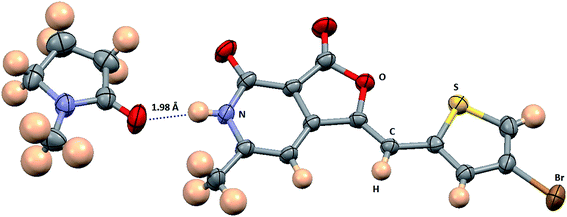 |
| Fig. 5 ORTEP diagram of compound 12p co-crystallized with N-methyl-2-pyrrolidone (NMP). Ellipsoids of non-hydrogen atoms are drawn at the 50% probability level. The color codes of different atom types are shown in the figure. The N–H⋯O hydrogen bond between 12p and NMP is shown as a blue dotted line. | |
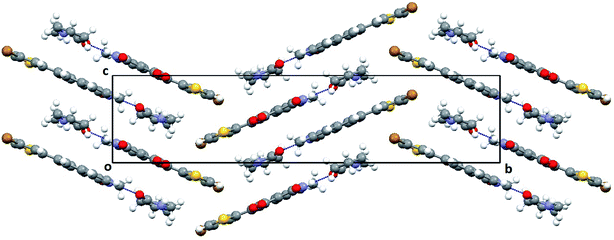 |
| Fig. 6 Packing diagram of 12p–NMP complex viewed down the a-axis showing the molecular arrangement in the crystal structure and the unique N–H⋯O hydrogen bond between 12p and NMP, shown as a blue dotted line. | |
Docking experiments of 12p
Molecular docking of 12p. To understand the binding interaction of 12p and acarbose with α-glucosidase, the molecular docking was conducted against the homology model of α-glucosidase. After docking, we received two alpha-glucosidase co12p and acarbose binding to the active site of modelled α-glucosidase protein by forming various bonded and non-bonded interactions. 12p is bonded to α-glucosidase with a predicted binding energy of −6.24 kcal mol−1, whereas acarbose binds to α-glucosidase with a predicted binding energy of −4.45 kcal mol−1.Our predicted binding model suggests that 12p formed two hydrogen bonds with ASP194 and SER588 residues via nitrogen of the pyridine moiety and sulphur of the thiophene moiety, respectively, while having a non-bonded interaction with TRP393, MET431, ASP528, PHE561, LEU562, LEU589, and LEU590 (Fig. 7a).
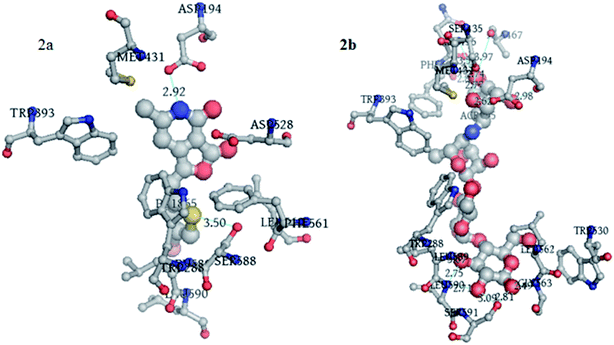 |
| Fig. 7 (a) 3D representation of interaction between 12p and alpha-glucosidase modelled protein. (b) 3D representation of interaction between acarbose and alpha-glucosidase modelled protein. | |
Acarbose formed hydrogen bonds with ASP194, SER 435, ASN436, ALA467, SER591, GLY563, LEU589 and LEU590 residues, while having a non-hydrogen bonded interaction with TRP288, TRP393, MET431, TRP530 and LEU562 (Fig. 7b).
Molecular dynamic simulation. MD simulations were performed for the alpha-glucosidase–12p complex by the Desmond 3.2 software, using the OPLS_2005 force field for a 47 ns simulation time. The protein–ligand complex was solvated in a 10 × 10 × 10 Å orthorhombic box with periodic boundary conditions by adding TIP3P water molecules. The whole system was neutralized by adding ions Na+ and Cl− so that the net charge of the system was 0. Herein, the default protocol of the Desmond software was used for relaxing the model. After default relaxation, which includes various restrained and without restrained minimization, the model was further relaxed using the NPT (number of atom, pressure, and temperature) ensemble class at 300 K temperature and 1.01325 bar pressure. Long-range electrostatic interactions were computed using the particle-mesh Ewald method and the van der Waals (VDW) cut-off was set to 9 Å. The SHAKE algorithm was used to satisfy the hydrogen bond geometry constraints during simulation.MD simulation helps in identifying the structural stability and flexibility present in the protein structure with time. It also helps in identifying the dynamic properties of the protein ligand complex. Simulation quality analysis plot shows that various parameters, such as total energy, potential energy, temperature, pressure and volume, were relatively stable throughout the MD simulation (Fig. 8a). RMSD plot for the protein backbone and heavy atoms shows initial rearrangement but attained stability after 10 ns of simulation (Fig. 8b). The same is observed in the case of the ligand RMSD (Fig. 9b). The backbone and heavy atoms of α-glucosidase showed a RMSD in the range of 1–2.6 Å and 1.2–3.2 Å, respectively. By analysing the root mean square fluctuation (RMSF) plot, it can be said that most of the residues were within the limit of 2.5 Å. Some residues, which were present in the loop of the protein, exceeded 2.5 Å (Fig. 8c). Protein secondary structure elements (SSE), such as alpha-helices and beta-strands, were monitored throughout the simulation and no major conformational changes in SSE were observed (Fig. 8d). The RMSD plot, RMSF plot, SSE plot and energy plot revealed that the α-glucosidase–12p complex was stable throughout the simulation.
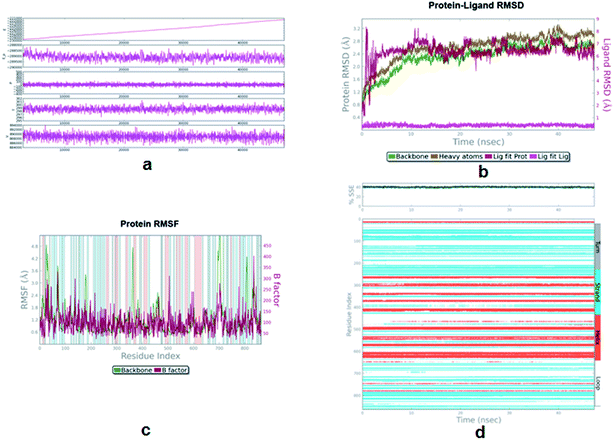 |
| Fig. 8 (a) Simulation quality plot of MD simulation of alpha-glucosidase–8 complex during a 47 ns simulation. This plot shows the total energy, potential energy, pressure, temperature and volume observed during the simulation period. (b) RMSD plot of the protein–ligand complex during simulation. (c) RMSF plot of protein–ligand complex during simulation. (d) Secondary Structure Elements (SSE) plot of the protein during simulation. | |
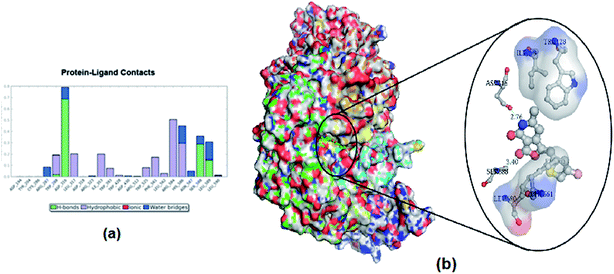 |
| Fig. 9 (a) Interaction plot of protein–ligand complex during simulation. (b) Interaction of 12p with α-glucosidase after 47 ns of simulation. | |
All the bonded and non-bonded interactions between 12p and α-glucosidase were monitored throughout the simulation. The overall interactions between 12p and α-glucosidase are shown in Fig. 9a. Simulation of the final structure of the α-glucosidase–12p complex along with their binding interactions after 47 ns is depicted in Fig. 9b. The major interaction between 12p and α-glucosidase from the simulation are H-bond interaction with ASP316, SER588 and LEU 589 residues, whereas hydrophobic interaction is with TRP 288, LEU 317, ILE353, PHE561, ARG584 and HIS586 residues. Water bridges were present with ARG287, HIS586, SER588, and LEU589.
Conclusion
Therefore, to generate a novel molecule against α-glucosidase, a small molecule library of 22 furopyridinediones was designed using an intuitive scaffold hopping and bioisosteric modification of a few existing α-glucosidase inhibitors. They were synthesized via an aldol condensation reaction, and the structures were confirmed based on NMR spectroscopy, mass analysis and single crystal X-ray crystallography of 12p. This library was further screened against α-glucosidase (yeast origin) with acarbose as the positive standard. Among the assayed compounds, 12p emerged as the most potent candidate showing inhibition with an IC50 value of 0.24 μM, which is 100-fold more active than the reference compound. This compound incorporated a bromide at the C4 position of a thiophene moiety, which in turn was connected to furopyridinedione at C2 via a methylene linkage. Reaction kinetics revealed it to be a mixed inhibitor. Molecular modelling and molecular dynamic simulation experiments revealed potential putative binding pockets of 12p along with the active region of α-glucosidase. This inhibitor can be considered as a favorable lead towards further investigation of an antidiabetic molecule.
Experimental
General
All reactions were carried out in flame-dried sealed tubes with magnetic stirring. Unless otherwise noted, all experiments were performed under an argon atmosphere. All reagents were purchased from Sigma Aldrich, Acros or Alfa Aesar. Solvents were treated with 4 Å molecular sieves or sodium and distilled prior to use. Purifications of reaction products were carried out by column chromatography using Chem Lab silica gel (230–400 mesh). Infrared spectra (IR) were obtained on a Thermoscientific Neoled IS5 FTIR spectrophotometer and are reported as wavenumber (cm−1). Infrared spectra were obtained by preparing a KBr pellet containing the title compound. 1H NMR and 13C NMR spectra were obtained with tetramethylsilane (TMS) as an internal standard at ambient temperature unless otherwise indicated on a Varian 300/400 and JEOL JNM-ECX500 MHz at 500 MHz for 1H NMR and 100 MHz for 13C NMR. Chemical shifts are reported in parts per million (ppm) and coupling constants are reported as Hertz (Hz). Splitting patterns are designated as singlet (s), broad singlet (bs), doublet (d), and triplet (t). Splitting patterns that could not be interpreted or easily visualized are designated as multiple (m). Mass spectrometry analysis was done on a 6540 UHD Accurate-Mass Q-TOF LC/MS system (Agilent Technologies) equipped with an Agilent 1290 LC system obtained by the Dept. of Chemistry, School of Natural Sciences, Shiv Nadar University, Uttar Pradesh 203207, India.
General procedure for the aldol condensation between 6-methylfuro[3,4-c]pyridine-3,4(1H,5H)-dione and appropriate aldehydes
To a stirred solution of 6-methylfuro[3,4-c]pyridine-3,4(1H,5H)-dione (300 mg, 1.8 mmol) in ethanol (10 mL), piperidine (15 mg, 0.18 mmol) was added, followed by the corresponding aldehyde (1.98 mmol) under a N2 atmosphere at room temperature, then the reaction mixture was stirred at 80 °C for 16 h, allowed to cool to room temperature and then diethyl ether (10 mL) was added. The resulting precipitate was collected by filtration, washed with diethyl ether (3 × 10 mL) and dried to give substituted 6-methylfuro[3,4-c]pyridine-3,4(1H,5H)-dione.
(Z)-1-(2,4-Dimethylbenzylidene)-6-methylfuro[3,4-c]pyridine-3,4(1H,5H)-dione (12a). Following the general protocol, 2,4-dimethylbenzaldehyde (267 mg, 1.98 mmol) afforded 12a in 238 mg (yield 47%). 1H NMR (300 MHz; DMSO-d6): δ 12.21 (br. s, 1H), 7.81 (m, 1H), 7.23–7.19 (m, 1H); 7.17–7.15 (m, 1H); 6.98–6.85 (m, 2H), 2.36 (s, 3H), 2.35–2.34 (m, 6H). 13C NMR (125 MHz; DMSO-d6): δ 164.84, 157.98, 156.65, 155.67, 143.16, 135.61, 135.39, 131.38, 130.95, 130.52, 109.28, 106.01, 97.79, 21.15, 19.92, 19.72. LCMS (ESI) m/z: [M + H]+ calculated for (C17H16NO3) 282.11, found 282.27.
(Z)-1-(4-Methoxy-2,3-dimethylbenzylidene)-6-methylfuro[3,4-c]pyridine-3,4(1H,5H)-dione (12b). Following the general protocol, 4-methoxy-2,3-dimethylbenzaldehyde (324 mg, 1.98 mmol) afforded 12b in 303 mg (yield 54%). 1H NMR (300 MHz; DMSO-d6): δ 12.15 (br. s, 1H), 7.85–7.83 (d, J = 6 Hz, 1H), 7.01–6.95 (m, 3H); 3.81 (s, 3H), 2.27–2.26 (d, J = 3 Hz, 6H), 2.18 (s, 3H). 13C NMR (125 MHz; DMSO-d6): δ 164.90, 158.29, 156.71, 155.11, 141.76, 138.47, 129.06, 124.98, 123.96, 110.47, 108.88, 105.45, 97.42, 56.04, 20.38, 16.39, 12.32. LCMS (ESI) m/z: [M + H]+ calculated for (C18H19NO4) 312.58, found 312.25.
(Z)-1-(2-Trifluoromethylbenzylidene)-6-methylfuro[3,4-c]pyridine-3,4(1H,5H)-dione (12c). Following the general protocol, 2-trifluoromethylbenzaldehyde (345 mg, 1.98 mmol) afforded 12c in 416 mg (yield 72%). 1H NMR (300 MHz; DMSO-d6): δ 11.99–11.80 (br. s, 1H), 8.20–8.18 (d, J = 6 Hz, 1H), 7.86–7.78 (m, 2H), 7.61–6.58 (m, 1H), 6.85 (s, 1H), 6.80 (s, 1H), 2.34 (s, 3H). 13C NMR (125 MHz; DMSO-d6): δ 164.19, 157.98, 157.02, 156.09, 145.45, 133.29, 132.57, 130.64, 129.69, 127.56, 127.31, 126.64, 106.59, 105.27, 97.52, 19.96. LCMS (ESI) m/z: [M + H]+ calculated for (C16H11F3NO3) 322.06, found 322.00.
(Z)-1-(3,4-Dihydroxybenzylidene)-6-methylfuro[3,4-c]pyridine-3,4(1H,5H)-dione (12d). Following the general protocol, 3,4-dihydroxybenzaldehyde (273 mg, 1.98 mmol) afforded 12d in 180 mg (yield 35%). 1H NMR (300 MHz; DMSO-d6): δ 7.39 (s, 1H), 7.16–7.05 (m, 1H), 6.80–6.75 (m, 2H), 6.72 (s, 1H), 2.28 (s, 3H). 13C NMR (125 MHz; DMSO-d6): δ 168.75, 167.43, 164.90, 158.35, 156.71, 155.05, 148.29, 146.05, 140.58, 124.67, 124.40, 117.66, 116.45, 113.39, 109.28, 105.14, 99.84, 97.03, 68.28, 20.00, 19.65. LCMS (ESI) m/z: [M + H]+ calculated for (C15H12NO5) 286.06, found 286.00.
(Z)-1-(3-Bromobenzylidene)-6-methylfuro[3,4-c]pyridine-3,4(1H,5H)-dione (12e). Following the general protocol, 3-bromobenzaldehyde (364 mg, 1.98 mmol) afforded 12e in 305 mg (yield 51%). 1H NMR (300 MHz; DMSO-d6): δ 12.41–12.21 (bs, 1H), 8.00 (s, 1H), 7.80–7.75 (d, J = 15 Hz, 1H), 7.61–7.56 (m, 1H), 7.48–7.40 (m, 1H), 6.99 (s, 1H), 6.68 (s, 1H), 2.31 (s, 3H). 13C NMR (125 MHz; DMSO-d6): δ 168.30, 158.04, 156.54, 156.27, 144.10, 135.53, 132.74, 132.23, 131.52, 129.71, 122.61, 109.86, 106.10, 97.35, 56.50, 20.10, 19.01. LCMS (ESI) m/z: [M + H]+ calculated for (C15H11BrNO3) 332.1488, found 332.0950.
(Z)-1-(4-Bromobenzylidene)-6-methylfuro[3,4-c]pyridine-3,4(1H,5H)-dione (12f). Following the general protocol, 4-bromobenzaldehyde (364 mg, 1.98 mmol) afforded 12f in 355 mg (yield 59%). 1H NMR (300 MHz; DMSO-d6): δ 12.35–12.25 (bs, 1H), 7.79–7.81 (m, 4H), 6.98 (s, 1H), 6.67 (s, 1H), 2.36 (s, 3H). 13C NMR (125 MHz; DMSO-d6): δ 164.37, 158.10, 156.61, 156.11, 143.67, 132.58, 132.49, 132.44, 123.18, 110.45, 106.02, 97.34, 20.09. LCMS (ESI) m/z: [M + H]+ calculated for (C15H11BrNO3) 332.1488, found 332.1330.
(Z)-6-Methyl-1-(naphthalen-2-ylmethylene)furo[3,4-c]pyridine-3,4(1H,5H)-dione (12g). Following the general protocol, 3-naphthaldehyde (311 mg, 1.98 mmol) afforded 12g in 404 mg (yield 74%). 1H NMR (300 MHz; DMSO-d6): δ 12.23 (bs, 1H), 8.59–8.42 (m, 1H), 8.25–8.19 (m, 1H), 8.01–7.95 (m, 2H), 7.65–7.52 (m, 4H), 7.19 (s, 1H), 2.36 (s, 3H). 13C NMR (125 MHz; DMSO-d6): δ 164.75, 158.31, 156.75, 155.92, 144.54, 133.84, 131.77, 130.29, 130.24, 129.77, 129.11, 127.42, 126.87, 126.17, 124.32, 107.50, 105.94, 97.93, 20.17. LCMS (ESI) m/z: [M + H]+ calculated for (C19H14NO3) 304.09, found 304.23.
(Z)-1-(3,4-Difluorobenzylidene)-6-methylfuro[3,4-c]pyridine-3,4(1H,5H)-dione (12h). Following the general protocol, 3,4-difluorobenzaldehyde (281 mg, 1.98 mmol) afforded 12h in 442 mg (yield 85%). 1H NMR (300 MHz; DMSO-d6): δ 12.25 (bs, 1H), 7.85–7.79 (m, 1H), 7.62–7.55 (m, 2H), 6.99 (s, 1H), 6.62 (s, 1H), 2.34 (s, 3H). 13C NMR (125 MHz; DMSO-d6): δ 164.21, 158.00, 156.47, 156.26, 143.69, 130.88, 128.11, 118.99, 118.85, 118.76, 118.62, 109.32, 106.00, 97.23, 20.07. LCMS (ESI) m/z: [M + H]+ calculated for (C15H10F2NO3) 290.05, found 290.17.
(Z)-1-(Benzo[d][1,3]dioxol-5-ylmethylene)-6-methylfuro[3,4-c]pyridine-3,4(1H,5H)-dione (12i). Following the general protocol, benzo[d][1,3]dioxole-5-carbaldehyde (300 mg, 1.98 mmol) afforded 12i in 256 mg (yield 48%). 1H NMR (300 MHz; DMSO-d6): δ 12.18 (bs, 1H), 7.40 (s, 1H), 7.35–7.33 (d, J = 6 Hz, 1H), 7.10–7.05 (m, 1H), 6.89 (s, 1H), 6.64–6.63 (d, J = 3 Hz, 1H), 6.05 (s, 2H), 2.34 (s, 3H). 13C NMR (125 MHz; DMSO-d6): δ 164.55, 158.19, 156.64, 155.50, 148.90, 148.33, 141.64, 127.38, 126.64, 112.13, 109.73, 109.39, 105.50, 102.18, 97.05, 20.02. LCMS (ESI) m/z: [M + H]+ calculated for (C16H12NO5) 298.06, found 298.18.
(Z)-1-(2,3-Dimethoxybenzylidene)-6-methylfuro[3,4-c]pyridine-3,4(1H,5H)-dione (12j). Following the general protocol, 2,3-dimethoxybenzaldehyde (328 mg, 1.98 mmol) afforded 12j in 362 mg (yield 64%). 1H NMR (300 MHz; DMSO-d6): δ 12.20 (bs, 1H), 7.40 (s, 1H), 7.64–7.62 (m, 1H), 7.21–7.15 (m, 2H), 6.95 (s, 1H), 6.88 (s, 1H) 3.80 (s, 6H), 2.31 (s, 1H). LCMS (ESI) m/z: [M + H]+ calculated for (C15H12NO5) 314.09, found 314.21.
(Z)-1-Enzylidene-6-methylfuro[3,4-c]pyridine-3,4(1H,5H)-dione (12k). Following the general protocol, benzaldehyde (210 mg, 1.98 mmol) afforded 12k in 321 mg (yield 74%). 1H NMR (500 MHz; DMSO-d6): δ 12.00 (br. s, 1H), 7.82–7.78 (m, 2H), 7.59–7.39 (m, 3H), 6.99 (s, 1H); 6.79 (s, 1H), 2.31 (s, 3H). 13C NMR (125 MHz; DMSO-d6): δ 164.59, 158.17, 156.78, 155.94, 143.19, 133.21, 130.86, 129.83, 129.49, 111.82, 105.95, 97.34, 20.20. LCMS (ESI) m/z: [M + H]+ calculated for (C15H12NO3) 254.07, found 254.14.
(Z)-6-Methyl-1-(4-nitrobenzylidene)furo[3,4-c]pyridine-3,4(1H,5H)-dione (12l). Following the general protocol, 4-nitrobenzaldehyde (300 mg, 1.98 mmol) afforded 12l in 493 mg (yield 48%). 1H NMR (500 MHz; DMSO-d6): δ 12.36 (br. s, 1H), 8.35 (d, 2H, J = 5 Hz), 8.015 (d, 2H, J = 5 Hz); 7.12 (s, 1H); 6.81 (s, 1H), 2.35 (s, 3H). 13C NMR (125 MHz; DMSO-d6): δ 166.60, 162.39, 161.43, 160.24, 146.85, 143.08, 130.95, 128.02, 128.02, 124.15, 124.15, 119.95, 22.55. HRMS (ESI-TOF) m/z: [M + H]+ calculated for (C15H11N2O5) 299.0662, found 299.0662.
(Z)-1-((1H-Indol-3-yl)methylene)-6-methylfuro[3,4-c]pyridine-3,4(1H,5H)-dione (12m). Following the general protocol, 1H-indole-3-carbaldehyde (290 mg, 1.98 mmol) afforded 12m in 374 mg (yield 71%). 1H NMR (500 MHz; DMSO-d6): δ 11.99 (br. s, 1H), 8.01 (m, 2H), 7.31–7.25 (m, 1H); 7.39 (s, 1H); 7.21–7.18 (m, 3H), 6.82 (s, 1H), 2.39 (s, 3H). 13C NMR (125 MHz; DMSO-d6): δ 168.77, 167.41, 164.88, 158.56, 158.38, 155.79, 155.05, 154.35, 139.38, 136.44, 130.69, 126.96, 123.02, 121.01, 119.26, 112.72, 109.59, 109.29, 106.85, 104.80, 99.84, 96.94, 68.29, 19.99, 19.65. LCMS (ESI) m/z: [M + H]+ calculated for (C17H13N2O3) 293.08, found 293.00.
(Z)-6-Methyl-1-((1-methyl-1H-imidazol-4-yl)methylene)furo[3,4-c]pyridine-3,4(1H,5H)-dione (2n). Following the general protocol, 1-methyl-1H-imidazole-4-carbaldehyde (218 mg, 1.98 mmol) afforded 12n in 315 mg (yield 68%). 1H NMR (500 MHz; DMSO-d6): δ 11.98 (br. s, 1H), 8.03 (m, 1H), 7.61–7.55 (m, 1H); 7.49 (s, 1H); 6.86 (s, 1H), 3.92 (s, 3H), 2.31 (s, 3H). 13C NMR (125 MHz; DMSO-d6): δ 164.36, 158.08, 155.29, 153.91, 138.86, 136.50, 133.84, 127.01, 122.71, 120.83, 118.90, 110.64, 108.20, 105.93, 96.48. LCMS (ESI) m/z: [M + H]+ calculated for (C13H12N3O3) 258.08, found 258.19.
(Z)-6-Methyl-1-(thiophen-2-ylmethylene)furo[3,4-c]pyridine-3,4(1H,5H)-dione (12o). Following the general protocol, thiophene-2-carbaldehyde (232 mg, 1.98 mmol) afforded 12o in 325 mg (yield 72%). 1H NMR (400 MHz; DMSO-d6): δ 12.17 (br. s, 1H), 7.86 (s, 1H), 7.47–7.19 (t, 3H); 6.71 (s, 1H); 2.32 (s, 3H). 13C NMR (125 MHz; DMSO-d6): δ 166.60, 163.68, 157.75, 155.30, 140.58, 135.63, 132.10, 132.02, 128.09, 106.01, 105.56, 96.68, 19.64. HRMS (ESI-TOF) m/z: [M + H]+ calculated for (C13H10NO3) 260.0303, found 260.0301.
(Z)-1-((4-Bromothiophen-2-yl)methylene)-6-methylfuro[3,4-c]pyridine-3,4(1H,5H)-dione (12p). Following the general protocol, 4-bromo-thiophene-2-carbaldehyde (380 mg, 1.98 mmol) afforded 12p in 395 mg (yield 65%). 1H NMR (400 MHz; DMSO-d6): δ 7.95 (s, 1H), 7.68 (s, 1H), 7.43 (s, 1H), 6.67 (s, 1H), 2.31 (s, 3H). 13C NMR (125 MHz; DMSO-d6): δ 163.79, 158.06, 156.27, 155.54, 142.34, 137.66, 133.12, 129.32, 110.43, 106.26, 104.66, 97.23, 20.10. HRMS (ESI-TOF) m/z: [M + H]+ calculated for (C13H9BrNO3S) 337.9481, found 337.9470.
(Z)-6-Methyl-1-((4-phenylthiophen-2-yl)methylene)furo[3,4-c]pyridine-3,4(1H,5H)-dione (12q). Following the general protocol, 4-phenyl-thiophene-2-carbaldehyde 15a (372 mg, 1.98 mmol) afforded 12q in 298 mg (yield 49%). 1H NMR (500 MHz; DMSO-d6): δ 12.20 (br. s, 1H), 8.17 (s, 1H) 7.83, (s, 1H), 7.73–7.72 (d, J = 6.85 Hz, 2H), 7.46–7.43 (t, J1 = 7.55 Hz, J2 = 15.1 Hz, 1H); 7.35–7.33 (d, J = 10.3 Hz, 2H), 6.72 (s, 1H), 5.13 (s, 1H), 2.34 (s, 3H). 13C NMR (125 MHz; DMSO-d6): δ 163.58, 157.70, 155.48, 155.19, 141.75, 141.08, 136.47, 134.28, 130.03, 129.05, 129.05, 127.61, 126.03, 126.03, 105.78, 105.62, 99.37, 96.72, 19.63. HRMS (ESI-TOF) m/z: [M + H]+ calculated for (C19H14NO3S) 336.0989, found 336.0710.
(Z)-6-Methyl-1-((4-(pyridin-3-yl)thiophen-2-yl)methylene)furo[3,4-c]pyridine-3,4(1H,5H)-dione (12r). Following the general protocol, pyridine-3-yl-thiophene-2-carbaldehyde 15b (374 mg, 1.98 mmol) afforded 12r in 207 mg (yield 34%). 1H NMR (400 MHz; DMSO-d6): δ 12.16 (br. s, 1H), 8.95 (s, 1H), 8.51–8.50 (d, J = 3.64 Hz, 1H), 8.30 (s, 1H), 8.11–8.09 (d, J = 7.76 Hz, 1H), 7.87 (s, 1H), 7.46–7.43 (m, 1H), 7.31 (s, 1H), 6.72 (s, 1H), 2.31 (s, 3H). 13C NMR (125 MHz; DMSO-d6): δ 169.11, 163.53, 157.69, 155.60, 155.16, 148.54, 147.13, 141.32, 138.49, 133.37, 130.06, 129.77, 128.11, 123.97, 105.63, 105.52, 96.75, 19.65. HRMS (ESI-TOF) m/z: [M + H]+ calculated for (C18H13N2O3S) 337.0641, found 337.0658.
(Z)-1-((4-(6-Methoxypyridin-3-yl)thiophen-2-yl)methylene)-6-methylfuro[3,4-c]pyridine-3,4(1H,5H)-dione (12s). Following the general protocol, 6-methoxypyridine-3-yl-thiophene-2-carbaldehyde 15c (433 mg, 1.98 mmol) afforded 12s in 336 mg (yield 51%). 1H NMR (500 MHz; DMSO-d6): δ 11.96 (bs, 1H); 8.43 (s, 1H); 8.15 (d, J = 4.2 Hz, 1H), 7.91 (s, 1H), 7.31 (s, 1H), 7.14 (s, 1H), 7.01 (d, J = 3.66 Hz, 1H), 6.72 (s, 1H), 3.89 (s, 3H) 2.29 (s, 3H). 13C NMR (125 MHz; DMSO-d6): 163.49, 159.56, 157.68, 155.59, 155.11, 153.98, 148.41, 142.66, 141.26, 140.02, 136.52, 130.03, 129.48, 110.67, 105.66, 103.67, 96.72, 79.03, 44.49, 28.08, 19.66. HRMS (ESI-TOF) m/z: [M + Na]+ calculated for (C19H14N20O2SNa) 389.0566, found 389.2034.
(Z)-tert-Butyl-4-(4-(5-((6-methyl-3,4-dioxo-4,5-dihydrofuro[3,4-c]pyridin-1(3H)-ylidene) methyl) thiophen-3-yl)pyridin-2-yl)piperazine-1-carboxylate (12t). Following the general protocol, tert-butyl 4-(5-(5-formylthiophen-3-yl)pyridin-2-yl)piperazine-1-carboxylate 15d (738 mg, 1.98 mmol) afforded 12t in 390 mg (yield 43%). 1H NMR (500 MHz; DMSO-d6): δ 11.93 (bs, 1H); 8.42 (s, 1H); 8.15 (d, J = 4.8 Hz 1H); 7.90 (s, 1H); 7.30 (s, 1H), 7.14 (s, 1H), 6.99 (d, J = 4.8 Hz, 1H), 6.71 (s, 1H), 2.35 (s, 3H), 3.44 (m, 4H), 3.34 (m, 4H), 1.42 (s, 9H). 13C NMR (125 MHz; DMSO-d6): 163.49, 159.56, 157.67, 155.59, 155.11, 153.97, 148.40, 142.65, 141.26, 140.02, 136.52, 130.02, 129.48, 110.67, 105.68, 105.53, 103.67, 96.72, 79.03, 44.48, 44.48, 28.08, 28.08, 28.08, 19.60. HRMS (ESI-TOF) m/z: [M + H]+ calculated for (C27H29N4O5S) 521.1853, found 521.1876.
(Z)-3-((4-((E)-4-Fluorostyryl)thiophen-2-yl)methylene)-4-methylfuro[3,4-c]pyridine-1,6(3H,5H)-dione (12u). Following the general protocol, (E)-4-(4-fluorostyryl)thiophene-2-carbaldehyde 15e (460 mg, 1.98 mmol) afforded 12u in 245 mg (yield 36%). 1H NMR (500 MHz; DMSO-d6): δ 12.21 (br. s, 1H), 7.87 (s, 1H), 7.72 (s, 1H); 7.64–7.61 (t, J1 = 7.55 Hz, J2 = 13.75 Hz, 1H); 7.30 (s, 1H), 7.24–7.21 (dd, J1 = 3.45 Hz, J2 = 3.4 Hz, 3H), 7.14 (s, 1H), 7.10 (s, 1H), 6.74 (s, 1H), 2.34 (s, 3H). 13C NMR (125 MHz; DMSO-d6): δ 163.49, 159.56, 157.67, 155.59, 155.11, 153.97, 148.40, 142.65, 141.26, 140.02, 136.52, 130.02, 130.02, 129.48, 110.67, 110.67, 105.68, 103.67, 96.72, 79.03, 19.66. HRMS (ESI-TOF) m/z: [M + H]+ calculated for (C21H15FNO3S) 380.0678, found 380.0779.
Synthesis of (Z)-6-methyl-1-((4-(piperidin-1-yl)thiophen-2-yl)methylene)furo[3,4-c]pyridine-3,4(1H,5H)-dione (12v). Following the general protocol, 4-(piperidin-1-yl)thiophene-2-carbaldehyde 15f (386 mg, 1.98 mmol) afforded 12v in 437 mg (yield 71%). 1H NMR (400 MHz; DMSO-d6): δ 10.69 (s, 1H), 7.92–7.89 (m, 2H), 7.26–7.25 (d, J = 4 Hz, 1H), 6.61 (s, 1H), 4.05 (s, 4H), 2.34 (s, 3H), 1.67 (s, 6H). 13C NMR (125 MHz; DMSO-d6): δ 167.31, 159.82, 158.82, 146.06, 143.57, 139.64, 132.09, 131.38, 130.38, 128.18, 95.65, 48.50, 48.50, 25.36, 25.36, 23.51, 18.99. HRMS (ESI-TOF) m/z: [M + H]+ calculated for (C18H19N2O3S) 343.1811, found 343.1851.
General procedure for the syntheses of 15a–e
To a stirred solution of 4-bromothiophene-2-carbaldehydes (1 eq.) in 1,4-dioxane (10 vol), the corresponding arylboronic acids (1.2 eq.) were added followed by aqueous (2 M) K2CO3 (3 eq.) purged with argon gas for 15 min and then bis(triphenylphosphine) palladium(II) dichloride (0.05 eq.) was added and the reaction mixture was heated at 100 °C for 14 h. Once TLC indicated complete consumption of the starting material, the reaction mixture was allowed to cool to room temperature then filtered through celite, quenched with 20 volumes of brine solution, extracted with ethyl acetate (2 × 10 vol), evaporated to dryness to give the crude substituted thiophene aldehydes (15a–e), which were used in the abovementioned reaction.
General procedure for the synthesis of 15f
To a stirred solution of 4-bromothiophene-2-carbaldehyde (200 mg, 1 mmol) in dimethyl formamide (10 mL), piperidine (90 mg, 1.1 mmol) and solid potassium carbonate (150 mg, 1.1 mmol) were added. The reaction mixture was heated at 110 °C for 6 h. Once TLC indicates complete consumption of the starting material, the reaction mixture was allowed to cool to room temperature then filtered through celite, quenched with 20 mL of water, extracted with ethyl acetate (2 × 10 mL) and then evaporated to dryness to give the crude 15f, which was used in the abovementioned reaction.
Pharmacological protocols
In vitro inhibition screening assay of α-glucosidase (yeast origin). The effectiveness of the compounds on inhibition of α-glucosidase (yeast origin) was assessed in 96-well plates using the respective substrates and buffering systems according to the procedure reported by Ferreres et al.23 Prior to assays, all the test compounds were dissolved in a suitable solvent, dimethylsulfoxide (DMSO) and eventually diluted to attain the desired concentration. The absorbance was measured spectrophotometrically at 400 nm (Epoch reader; version 2.00.18). The decrease in absorbance (ΔA) was compared with that of a control (buffer instead of test compound) to compute the inhibitory profile of the enzyme. The data used for the determination of IC50 concentrations were fitted by non-linear regression fitting and the variance analysis was carried out using MINITAB 15 software (trail version). The concentration of inhibition required for 50% of α-glucosidase activity under the assay conditions is defined as the IC50 value. The half maximal inhibitory (IC50) concentrations were determined from two independent assays performed in duplicate. Acarbose, an eminent α-glucosidase inhibitor, was employed as a positive control.
Inhibition (%) = (ΔAcontrol − ΔAsample)/ΔAcontrol × 100% |
One unit (IU) is defined as the amount of enzyme (α-glucosidase), which produces 1 μmol of PNP (p-nitro phenol) per min at 37 °C under the respective reaction conditions described below.
In brief, the assay condition was as follows:
α-Glucosidase inhibition (AGI). For AGI, 100 μL of p-nitrophenol-α-D-glucopyranoside substrate (2 mM, PNP-Glu was dissolved in 2 mM phosphate buffer at pH 7.2) and different concentrations of the test compounds were taken in 96-well plates. Then, the final volume of the reaction mixture was made up to 200 μL with 2 mM phosphate buffer (pH 7.2). The hydrolytic reaction commenced with the addition of α-glucosidase enzyme (0.5 IU mL−1) (obtained from Sigma Aldrich, Bangalore) and the plates were incubated at 37 °C for 15 min. The reaction was terminated by the addition of 50 μL of 2 N Na2CO3 solution.
Inhibition-kinetics. The kinetic mode of inhibition of the highest active compounds against α-glucosidase (yeast origin) was determined by preparing a series of test solutions in which the concentration of the respective substrate was varied in the presence of different concentrations of the inhibitors. The mode of inhibition (i.e. competitive, non-competitive, uncompetitive or mixed-type) of the screened compounds was determined on the basis of the inhibitory effects on Km (Michaelis constant: it is defined as the substrate concentration at half of the maximum velocity of the enzyme) and Vmax (maximum reaction velocity) of the enzyme.22 These studies were computed using the primary (Lineweaver–Burk plot) plots, which are the double reciprocal plots of enzyme reaction velocities (1/V) versus substrate concentrations (1/[S]). Analysis of the same data by secondary plots of slope versus [inhibitor] and Y-intercept versus [inhibitor] were also performed. The Lineweaver–Burk (LB) equation is as follows,
Molecular docking methodology
Homology modelling. The sequence of alpha-glucosidase was downloaded from Uniprot (ID: P10253). Blastp against protein data bank database was performed to identify the template for sequence alignment. Human maltase-glucoamylase (PDB ID: 2QLY, 3L4T) showed good similarity to our query sequence. Residues starting from 89 are aligned to these PDB showing a 44% identity. These two protein structures were chosen to model alpha-glucosidase using homology modelling. The homology model of alpha-glucosidase was built using Modeller v9.14. Three models were generated using Modeller v9.14 and the model having the best DOPE score was selected for further refinement. The modelled structure consists of certain loop structures that were further refined using ModLoop server. The energy of the refined model was minimized using Maestro v10 using Force Field OPLS2005. The stereochemical quality of this model was validated by a Ramachandran plot. More than 99.3% of the residues were in the favored and allowed regions and only 0.7% (6 residues) were in the outlier region (Fig. 1).
Preparation of modelled alpha glucosidase protein structure. Protein preparation was performed using protein preparation wizard of Maestro v10 in which assign bond orders, addition of hydrogens and di-sulfide bond forming were done. Furthermore, H-bond assignments were optimized using PROPKA at pH 7.0. Finally, the energy of the protein was minimized using the OPLS2005 force field.
Ligand preparation. The 2D structure of 12p and natural substrate of alpha glucosidase, i.e. acarbose were built using the MOE-Builder tool. Furthermore, various 3D conformations were searched using the conformational search tool in MOE. The conformations having the least energy were selected for docking studies.
X-ray crystal structure determination. X-ray diffraction data for 12p were collected at room temperature using Mo Kα (λ = 0.71073 Å) radiation on a Bruker D8 Venture diffractometer system equipped with dual micro focus sources and a Photon 100 CMOS detector. The data collection strategy employed for 12p was omega and phi scan. The crystal structure was solved by a direct method using the SHELXS program implemented in the SHELXTL package and refined by full-matrix least squares methods using SHELXL-2014.24 The non-hydrogen atoms were located in successive difference Fourier syntheses and refined with anisotropic thermal parameters. All the hydrogen atoms were placed at the calculated positions and refined using a riding model with appropriate HFIX commands. ESI.†
Acknowledgements
The authors would like to sincerely appreciate Shiv Nadar University for funding.
References
- Y. Shi and F. B. Hu, Lancet, 2014, 383, 1947–1948 CrossRef.
- S. Wild, G. Roglic, A. Green, R. Sicree and H. King, Diabetes Care, 2004, 27, 1047–1053 CrossRef.
- D. V. Nguyen, L. C. Shawand and M. B. Grant, Front. Endocrinol., 2012, 3, 1–7 Search PubMed.
- S. E. Nissen and K. Wolski, N. Engl. J. Med., 2007, 356, 2457–2471 CrossRef CAS PubMed.
-
(a) J. B. Buse, D. C. Klonoff and L. L. Nielsen, et al., Clin. Ther., 2007, 29, 139–153 CrossRef CAS PubMed;
(b) F. X. Pi-Sunyer, A. Schweizer and D. Mills, et al., Diabetes Res. Clin. Pract, 2007, 76, 132–138 CrossRef CAS PubMed.
- D. Cota, G. Marsicano and M. Tschop, et al., J. Clin. Invest., 2003, 112, 423–431 CrossRef CAS PubMed.
- K. C. Maki, M. L. Carson, M. P. Miller, M. Turowski, M. Bell, D. M. Wilder and M. S. Reeves, Diabetes Care, 2007, 30, 1039–1043 CrossRef CAS PubMed.
- H. A. Ernst, et al., J. Mol. Biol., 2006, 358, 1106–1124 CrossRef CAS PubMed.
-
(a) B. Henrissat, Biochem. J., 1991, 280, 309–316 CrossRef CAS;
(b) B. Henrissat and A. Bairoch, Biochem. J., 1993, 293, 781–788 CrossRef CAS.
-
(a) A. J. Krentz and C. J. Bailey, Drugs, 2005, 65, 385–411 CrossRef CAS;
(b) D. O. Schmidt, W. Frommer, L. Muller and E. Truscheit, Naturwissenschaften, 1979, 66, 584–585 CrossRef CAS;
(c) W. Puts, U. Keup, I. J. P. Krause, G. Thomas and F. HoO'meister, Naturwissenschaften, 1977, 64, 536–537 CrossRef.
- N. Asano, Curr. Top. Med. Chem., 2003, 3, 471–484 CrossRef CAS.
- T. Tanaka, R. Uehara, K. Nishida and I. Kouno, Phytochemistry, 2005, 66, 675–681 CrossRef CAS PubMed.
- T. K. Tabopda, J. Ngoupayo, P. K. Awoussong, A. Mitaine-Offer, M. S. Ali, B. T. Ngadjui and M. Lacaille-Dubois, J. Nat. Prod., 2008, 71, 2068–2072 CrossRef CAS PubMed.
- H. Oe and S. Ozaki, Biosci., Biotechnol., Biochem., 2008, 72, 1962–1964 CrossRef CAS PubMed.
- M. Shibano, D. Tsukamoto, A. Masuda, Y. Tanaka and G. Kusano, Chem. Pharm. Bull., 2001, 49, 1362–1365 CrossRef CAS.
- D. Zhang, M. Fu, S. Gao and J. Li, Evidence-based Complementary and Alternative Medicine, 2013, p. 16. Article ID 636053, DOI:10.1155/2013/636053.
- N. J. Pawar, V. S. Parihar, S. T. Chavan, R. Joshi, P. V. Joshi, S. G. Sabharwal, V. G. Puranik and D. D. Dhavale, J. Org. Chem., 2012, 77, 7873–7882 CrossRef CAS PubMed.
- M. Khan, M. Yousaf and A. Wadood, et al., Bioorg. Med. Chem., 2014, 22, 3441–3448 CrossRef CAS PubMed.
-
(a) T. Narender, et al., Eur. J. Med. Chem., 2013, 63, 162–169 CrossRef CAS PubMed;
(b) W. Hou, Y. Li, Q. Zhang, X. Wei, A. Peng, L. Chen and Y. Wei, Phytother. Res., 2009, 23, 614–618 CrossRef CAS PubMed.
-
(a) M. Fioravanti and L. Flicker, Cochrane Database of Systematic Reviews, 2001, 4, CD003159 Search PubMed;
(b) M. Fink and P. Irwin, Clin. Pharmacol. Ther., 1981, 30, 336–342 CrossRef CAS PubMed;
(c) F. Passamonti and M. Lazzarino, Leuk. Lymphoma, 2003, 44, 1483–1488 CrossRef CAS PubMed;
(d) P. N. Mohite and A. M. Bhatnagar, The Internet Journal of Surgery, 2009 vol. 21, ISSN 1528-8242 Search PubMed.
- H. Lineweaver and D. Burk, J. Am. Chem. Soc., 1934, 56, 658–666 CrossRef CAS.
- B. T. Burlingham and T. S. Widlanski, J. Chem. Educ., 2003, 80, 214–218 CrossRef CAS.
- F. Ferreres, A. Gil-Izquierdo, J. Vinholes, S. T. Silva, P. Valentao and P. B. Andrade, Food Chem., 2012, 134, 894–896 CrossRef CAS PubMed.
- G. M. Sheldrick, SHELXTL Version 2014/7, http://shelx.uni-ac.gwdg.de/SHELX/index.php Search PubMed.
Footnotes |
† Electronic supplementary information (ESI) available. CCDC 1401263 (12p). For ESI and crystallographic data in CIF or other electronic format see DOI: 10.1039/c5ra19255b |
‡ Equal contributor. |
|
This journal is © The Royal Society of Chemistry 2015 |