DOI:
10.1039/C5RA13642C
(Paper)
RSC Adv., 2015,
5, 90386-90395
Nanostructured lipid carriers modified with PEGylated carboxymethylcellulose polymers for effective delivery of docetaxel
Received
12th July 2015
, Accepted 16th October 2015
First published on 16th October 2015
Abstract
An amphiphilic carboxymethylcellulose-graft-histidine/methoxypolyethylene glycol (CMP) copolymer was firstly synthesized to modify nanostructured lipid carriers (NLCs), and the impact of this CMP modification on in vitro and in vivo behaviors of NLCs was investigated in detail. An obvious core–shell structure of CMP-coated nanostructured lipid carriers (CNLCs) was observed by a transmission electron microscope (TEM). Docetaxel (DTX) efficiently encapsulated in CNLCs formed 88.7 ± 1.11 nm particles with a negative zeta potential of −20.9 ± 1.11 mV. In vitro release studies indicated that the CMP coating significantly reduced the burst drug release at pH 7.4, whereas DTX-loaded CNLCs (DTX-CNLCs) could release the drug as quickly as DTX-loaded NLCs (DTX-NLCs) at pH 5.0. The pharmacokinetic performances proved that DTX-CNLCs had longer retention times in blood and higher area under the concentration–time curve (AUC) in rats than DTX-NLCs (p < 0.05). Furthermore, cell uptake, cytotoxicity, and cell apoptosis assays in MCF-7 cells consistently demonstrated that no obvious difference was found between DTX-CNLCs and DTX-NLCs (p > 0.05), indicating that this modification did not disturb effective killing of the cancer cells. As expected, DTX-CNLCs exhibited better antitumor effects than DTX-NLCs in the tumor-bearing nude mice (p < 0.05), due to their long circulation effect. Consequently, CNLCs hold great potential for effective delivery of anticancer drugs.
1. Introduction
Nanostructured lipid carriers (NLCs) composed of a solid lipid matrix with a certain content of liquid lipid are the second generation of lipid nanoparticles (NPs), which possess many merits as anticancer drug vehicles such as good biocompatibility, high drug entrapment efficiency (EE), small particle size, and passive targeting via the enhanced permeability and retention (EPR) effect.1,2 Furthermore, it was reported that drugs encapsulated in this delivery system could obtain specific targeting effect and enhanced antitumor efficacy. For example, baicalein-loaded tocol NLCs had the tendency of specific brain targeting.3 Docetaxel (DTX) modified with NLCs exhibited an improved cytotoxicity against A549 cells and superior treatment of malignant melanoma.4 Unfortunately, plain NLCs are often confronted with burst release behaviors5 and rapid uptake by the mononuclear phagocyte system (MPS),6 which could affect the efficacy of anticancer drugs and even cause adverse effects in vivo. Surface modification is an effective approach to modulate drug release profile, prolong blood circulation of NPs, and increase antitumor effect. It is generally known that polyethylene glycol (PEG) forming a hydrophilic shield on the surface of particles can reduce the rate of MPS uptake, and then prolong drug circulation time in blood.7–9 Oridonin-loaded long circulating NLCs showed significantly higher area under the concentration–time curve (AUC) and longer mean residence time (MRT) than unmodified NLCs (p < 0.05), due to the presence of PEG coating.10 In previous studies, PEG molecules were usually conjugated with stearic acid (SA) to generate an amphiphilic polymer for lipid nanoparticle modification. SA was incorporated into the lipid core, while the hydrophilic PEG chain was on the surface of colloidal carriers.11,12 However, it should be noted that incorporating PEG-SA into the lipid matrix might reduce drug encapsulation and accelerate drug release at pH 7.4.8,10 These problems should be taken into consideration regarding surface modification and its applications in drug delivery. Interestingly, polysaccharides show great potential in surface modification of lipid NPs. Besides the high stability and good safety profile,13 polysaccharides absorbed on the surface of lipid NPs had the ability to slow drug release rate.14 In addition, Yang et al. reported that the MRT of hyaluronic acid-coated NLCs (HA-NLC) was increased by approximately 1.6 times as compared to that of taxol.15
The above background information has recently encouraged us to design a PEGylated carboxymethylcellulose polymer to modify NLCs. Carboxymethylcellulose (CMC) is an important excipient in pharmaceutical products due to its abundant source, high safety, and good biocompatibility (FDA inactive ingredients database). CMC-based nanocarriers show promising applications in drug delivery fields.16,17 Of what interest is the PEGylated carboxymethylcellulose conjugate of docetaxel developed by Ernsting et al. First, the conjugate could be effectively internalized into a panel of tumor cells,18 indicating its good interaction with tumor cells. Moreover, it could obviously improve the pharmacokinetics, biodistribution, and anticancer efficacy of DTX compared to free DTX, partly as a result of the protection of the PEGylated carboxymethylcellulose polymer.19,20 With pH-sensitivity and much perfect biocompatibility, histidine served as side-chain pendants to design amphiphilic copolymers has attracted substantial interest.21,22 The “proton sponge effect” caused by the protonation of imidazole group could disrupt the lysosomal membrane and then facilitate the rapid anticancer drug release into the cytoplasm.23 In our previous publication, the “smart” carboxymethylcellulose-graft-histidine copolymer showed great potential in drug delivery.24 Therefore, the superiorities of these well-selected materials are combined to develop an amphiphilic carboxymethylcellulose-graft-histidine/methoxypolyethylene glycol (CMP) copolymer.
DTX belonging to the taxane family of antimitotic agents was used as a model drug. It is commonly used in the treatment of various types of solid tumors including ovarian, non-small cell lung, breast, prostate, and head and neck cancers.25 Because of poor aqueous solubility, the commercial formulation Taxotere® is prepared with high concentration of Tween 80, which has been reported to cause severe allergic reactions and peripheral neuropathology.26 In this study, NLCs were modified with CMP for effective delivery of DTX. We compared its behavior with the behavior of unmodified NLCs in terms of their size, zeta potential, drug encapsulation, drug release, and pharmacokinetics. Furthermore, in vitro cellular uptake, cytotoxicity, and cell apoptosis were performed in MCF-7 cells to assess whether the CMP coating could affect the ability of NLCs to kill cancer cells. Finally, in vivo antitumor efficacy was studied in the BALB/c nude mice model bearing A549 tumor nodule to compare the antitumor effect of NLCs with or without CMP coating.
2. Materials and methods
2.1. Materials
Carboxymethylcellulose (CMC, degree of substitution (DS) = 0.81, low viscosity) was kindly provided by Sunhere Pharmaceutical Excipients Co., Ltd (Anhui, China). L-Histidine was obtained from Kyowa Amino Acid Co., Ltd (Shanghai, China). Methoxypolyethylene glycol amine (mPEG-NH2, molecular weight (MW) = 2000) was purchased from Seebio Biotech, Inc. (Shanghai, China). Ethyl-3-(3-dimethylaminoprop-yl)-carbodiimide HCl (EDC), N-hydroxysuccinimide (NHS), and sodium deoxycholate were purchased from Aladdin Reagent Database Inc. (Shanghai, China). Coumarin-6 (C6) and 4-dimethylaminopyridine (DMAP) were obtained from J&K Scientific Ltd (Beijing, China). Docetaxel was purchased from Jinhe Bio-Technology Co., Ltd (Shanghai, China). Long chain oil (LCT) was purchased from Tieling Beiya Pharmaceutical Oil Co., Ltd (Liaoning, China). Pluronic F68 (F68) was kindly donated by Fengli Jingqiu Commerce and Trade Co., Ltd (Beijing, China). Compritol 888 ATO was provided by Gattefosse Co. (St-Priest, France). Lecithin was purchased from Lipoid (Germany). Dulbecco's Modified Eagle's Medium (DMEM), RPMI 1640 and heat-inactivated fetal bovine serum (FBS) were obtained from Gibco Life Technologies, Inc (NY, USA). 4′6-Diamidino-2-phenylindole (DAPI) and 3-(4,5-dimethylthiazol-2-yl)-2,5-diphenyltetrazolium bromide (tetrazole) (MTT) was bought from Beyotime Institute of Biotechnology (Shanghai, China). Annexin V-FITC/PI double staining assay kit was supplied by KeyGEN (Nanjing, China). All other reagents were analytical grade and used without any further purification.
The mouse L929 fibroblasts cell line and human breast cancer MCF-7 cell line were purchased from American Type Culture Collection (ATCC Distributor Beijing Zhongyuan Ltd., Beijing, China). The human pulmonary adenocarcinoma A549 cell line was provided by Chinese Academy of Sciences (Shanghai, China).
2.2. Cell culture
L929 cells were cultured with RPMI 1640 medium with 10% (v/v) FBS and 1% (v/v) penicillin (100 U mL−1) and streptomycin (100 μg mL−1). MCF-7 and A549 cells were cultured using DMEM supplemented with 10% (v/v) FBS and 1% (v/v) penicillin (100 U mL−1) and streptomycin (100 μg mL−1). All cells were maintained at 37 °C in a humidified 5% CO2 atmosphere.
2.3. Synthesis and characterization of CMP copolymer
Firstly, hydroxyl groups of CMC were acetylated with acetic anhydride according to the literature method.18 Then, L-histidine and mPEG-NH2 were coupled to the acetylated CMC (CMC-Ac) by EDC/NHS-mediated coupling reaction. Specifically, 150 mg of CMC-Ac (0.6 mmol carboxyl) was dissolved in MeCN (1 mL). EDC (0.6 mmol) was dissolved in a mixture of MeCN (6 mL) and distilled water (0.3 mL). NHS (0.6 mmol) and DMAP (0.06 mmol) were dissolved in MeCN (1 mL). L-Histidine (2.0 mmol) was dissolved in distilled water (8 mL). mPEG-NH2 (0.175 mmol) was dissolved in MeCN (0.5 mL). Then EDC, NHS, and DMAP were added to the solution of CMC-Ac, and the solution mixture was stirred for 4 h to activate the carboxyl groups of CMC-Ac. Subsequently, L-histidine and mPEG-NH2 were added, and the mixed solution was stirred for another 48 h at room temperature. The resulting solution was purified by dialysis (molecular weight cut-off 8000 Da, Greenbird Inc., Shanghai, China) against distilled water and freeze-dried. The chemical structure of the CMP copolymer was characterized by proton nuclear magnetic resonance (1H NMR, Bruker 600, Switzerland) using DMSO as the solvent.
Cytotoxicity of CMP copolymer against L929 cells was monitored using the MTT assay. All cells at a logarithmic growth phase, were detached and plated in 96-well microplates (Costar, IL, USA) at densities of 5 × 103 cells per well. The cells were then exposed to various concentrations of CMP solution (50–600 μg mL−1). After 24 h incubation, 20 μL MTT was added to the medium and further incubated for 4 h. The medium in each well was removed and 100 μL DMSO was added to dissolve the internalized purple formazan crystals. The absorbance was monitored at 490 nm in a Thermo Scientific microplate reader (Multiskan GO, Finland). Cell viability was calculated by the following equation:
where
Asample and
Acontrol are the absorbance with and without sample treatment, respectively.
Ablank is the absorbance of the medium.
2.4. Preparation and characterization of DTX-loaded NLCs (DTX-NLCs) and DTX-loaded CNLCs (DTX-CNLCs)
Plain NLCs and CMP-coated NLCs (CNLCs) were prepared by emulsification-ultrasonic and low temperature-solidification method. More specifically, the oil phase consisted of 9 mg DTX (no DTX for blank NLCs), 100 mg Compritol 888 ATO, 50 mg LCT, and 50 mg lecithin. Meanwhile, 10 mL of the aqueous phase consisted of 125 mg F68 and 25 mg sodium deoxycholate. Both phases were heated separately to 76 °C, and then the aqueous phase was added to the lipid phase. The mixture was vigorously stirred with a magnetic stirrer for 15 min, and further treated using a probe-type sonicator (JY92-II, Scientz Biotechnology Co., Ltd., Ningbo, China) at 400 W for 3 min. Finally, DTX-NLCs were obtained by solidification in an ice bath. For the preparation of DTX-CNLCs, 10 mg of the polymer CMP was added to above-mentioned aqueous phase. Other procedures were similar to the formation of DTX-NLCs. The morphological examinations of NLCs and CNLCs were measured under a transmission electron microscopy (TEM, JEM-1200EX, Japan). Samples were negatively stained by 2% phosphotungstic acid and placed on carbon-coated copper grids, followed by drying at room temperature before measurements. The particle size of DTX-NLCs and DTX-CNLCs was analyzed by dynamic light scattering (DLS, Zetasizer Nano-ZS90, Malvern) at 90° scattering angle at 25 °C. The zeta potential was determined by laser doppler velocimetry (LDV) using the same instrument.
2.5. Drug encapsulation efficiency
The prepared NPs were filtered with a microfiltration membrane (0.45 μm) to remove insoluble compounds. Then, an aliquot of DTX-CNLCs or DTX-NLCs was disrupted with methanol, and the total DTX concentration in the colloidal system was quantified by a high performance liquid chromatography (HPLC, LC-10AT, Shimadzu, Japan) equipped with a reverse phase C-18 column (Diamonsil (2), 250 × 4.6 mm, 5 μm).27 The mobile phase consisted of MeCN and deionized water (54/46, v/v) with a flow rate at 1 mL min−1. The detection wavelength was set at 228 nm. Non-encapsulated DTX (free drug) in the colloidal system was separated by ultrafiltration (10 kDa, Millipore) with centrifugation at 20
000 rpm for 60 min, and free drug in the supernatants was measured by HPLC. The EE (%) was calculated using the following equation:
where Ws is the actual amount of DTX encapsulated in NLCs or CNLCs, and Wt is the total amount of DTX added.
2.6. In vitro release studies
To determine drug release profile, aliquots of DTX-NLCs and DTX-CNLCs suspensions were placed in a dialysis bag with a molecular weight cut-off of 3.5 kDa, respectively. The dialysis bag was immersed in 50 mL PBS (pH 5.0 or pH 7.4) containing Tween 80 (0.5% w/v) and shaken at 37 °C in a water bath with 100 rpm. At predetermined time intervals, 1 mL of the incubation medium was removed, while an equivalent volume of fresh medium was added. The amount of DTX released at each time point was determined by HPLC. In vitro release profile was obtained by representing the percentage of drug released with respect to the amount of DTX loaded in the system.
2.7. Pharmacokinetics studies
Ten healthy adult male Wistar rats (mean body weight 220 g, Laboratory Animal Center of Shenyang Pharmaceutical University, Shenyang, Liaoning, China) were housed in a room with controlled temperature and humidity and had free access to food and water. The experimental protocols involving animal study were performed according to the Guidelines for the Care and Use of Laboratory Animals that was approved by the Committee of Ethics of Animal Experimentation of Shenyang Pharmaceutical University.
The rats were randomly divided into two groups and fasted overnight prior to the experiments. Two groups of rats were injected DTX-NLCs and DTX-CNLCs at a dose of 10 mg kg−1 through the caudal vein, respectively. The whole blood samples (0.3–0.5 mL) were collected by puncture of the retro-orbital sinus into heparinized tubes at 0.083, 0.17, 0.25, 0.5, 1, 2, 3, 4, 6, 8, 12 h. The blood samples were centrifuged immediately at 10
000 rpm for 10 min, and the plasma was collected and stored at −75 °C until analysis. Then 100 μL of plasma was mixed with 2 mL of ether and 10 μL paclitaxel (5 μg mL−1) was added as the internal standard. The mixture was mixed for 5 min, and then centrifuged at 10
000 rpm for 10 min. The organic phase was withdrawn and dried under a nitrogen stream at 40 °C. The dried residue was reconstituted with 100 μL of methanol, and quantified by HPLC. Pharmacokinetic parameters were calculated using the DAS (version 2.1.1).
2.8. Cellular uptake studies
The cellular uptake efficiency of plain NLCs and well-designed CNLCs was qualitatively evaluated by confocal laser scanning microscope (CLSM) using coumarin-6 (C6) as a fluorescence probe.28 C6-loaded NLCs (C6-NLCs) and C6-loaded CNLCs (C6-CNLCs) were similarly prepared except that DTX was replaced by C6. MCF-7 cells were seeded into 6-well black plates (Corning, NY, USA) at 1.8 × 105 cells per well. After the cells reached 80% confluence, C6-NLCs and C6-CNLCs dispersed in the cell culture medium (C6 content: 200 ng mL−1) were added into the wells. Cells were washed three times after incubation for 1 h or 4 h, and then fixed with 4% paraformaldehyde (v/v) at room temperature for 15 min, followed by cell nuclei staining with DAPI for another 10 min. Finally, cells were observed by a LSCM (Nikon, C2si, Japan).
The cellular uptake was also analyzed quantitatively by flow cytometry. After 1 h or 4 h of incubation at 37 °C, cells were washed with cold PBS (pH 7.4) to remove C6 formulations, harvested and subsequently resuspended in 0.5 mL PBS for flow cytometrix analysis using FACSCalibur flow cytometry (BD Biosciences, USA) by counting 10
000 cells. The autofluorescence of MCF-7 cells was used as a blank control. All measurements were performed in triplicate.
2.9. Cytotoxicity assays
MCF-7 cells were seeded in 96-well plates at 5 × 103 cells per well and incubated for 24 h at 37 °C. Then cells were incubated with CMP copolymer, DTX-NLCs, DTX-CNLCs, and DTX solution (dissolved in DMSO) at various drug concentrations from 0.001 to 20 μg mL−1 for 24 h and 48 h, respectively. The concentration of CMP copolymer in cell culture was matched to the drug concentration. At designated time intervals, the medium was removed and the wells were washed three times with PBS. MTT assay was used to measure the cell viability according to the method described in Section 2.3. The 50% growth inhibition (IC50) values of different formulations were calculated.
2.10. Cell apoptosis assays
Cell apoptosis was determined by the Annexin V-FITC/PI double staining assay. MCF-7 cells were incubated with DTX-NLCs, DTX-CNLCs, or DTX solution (dissolved in DMSO) at a drug concentration of 0.1 μg mL−1 for 48 h. Untreated cells acted as a control. After treatment, cells were collected by centrifugation and washed twice with cold PBS at 1000 rpm for 10 min. Thereafter, cells were gently resuspended in 200 μL binding buffer and incubated with 10 μL of Annexin V-FITC and 5 μL of PI at room temperature in the dark for 15 min. The stained cells were analyzed by FACScan flow cytometer (BD Biosciences, USA).
2.11. In vivo antitumor efficacy studies
The antitumor efficacy study was assessed in 6 week-old male BALB/c nude mice bearing A549 cells (20 ± 2 g, Laboratory Animal Center of Shenyang Pharmaceutical University, Shenyang, Liaoning, China). The animal experiments were performed according to the Guidelines for the Care and Use of Laboratory Animals that was approved by the Committee of Ethics of Animal Experimentation of Shenyang Pharmaceutical University.
For in vivo implantation, 2.5 × 106 A549 cells were suspended in 0.1 mL normal saline and injected subcutaneously into the right flank of mice. When the tumor volume reached 100–250 mm3, these mice were randomly divided into three groups (n = 5), and the day was designated as “day 1”. The three groups were respectively treated by tail vein injection of normal saline, 5 mg kg−1 DTX-NLCs, or 5 mg kg−1 DTX-CNLCs at day 1, 4 and 7. Mice body weights were weighted and tumor volumes were measured using a caliper every day during the experiment period. The tumor size (V) was calculated by the formula: V = 0.5 × length × width2. The mice were sacrificed at day 10, and the excised tumors were weighed. The tumor inhibition ratio (TIR) could be calculated as follows: TIR% = (Wc − Wt)/Wc × 100%. Wc and Wt were the average tumor weight of the control group and treatment group, respectively.
2.12. Statistical analysis
The data were statistically analyzed by using SPSS 17.0 software (Chicago, IL, USA). Statistical analysis was performed as a one-way analysis of variance (ANOVA) and comparisons among groups were performed by independent sample t-tests. The p values <0.05 were considered different. The p values <0.01 were considered significantly different. The p values >0.05 were considered to be not significant. Each experiment was repeated at least three times with a minimum of three samples.
3. Results and discussion
3.1. Characterization of CMP copolymer
As shown in Fig. 1A, the protons of CMC appeared as broad resonances between 2.99 and 5.25 ppm.18,29 The proton signals of the acetyl methyl groups were observed at δ = 1.88–2.07 ppm.30 The peaks at 6.81 and 7.62 ppm were ascribed to 4-H (–N–CH
C–) and 2-H (–N
CH–) imidazole proton of imidazole group, respectively,23 indicating that L-histidine was successfully attached to CMC-Ac. The appearance of an intense signal at 3.70 ppm was ascribed to the PEG methylene units, indicating the incorporation of mPEG. With the result of the analysis, the CMP copolymer (Fig. 1B) was successfully synthesized. The number of mPEG grafted to 100 saccharide units was calculated to be 8.9 ± 1.6% by comparing the integral areas between mPEG signal and acetyl methyl signals. The substitution degree of L-histidine was calculated to be 20.6 ± 0.8% based on the ratio of the integral for the signals of imidazole protons to acetyl methyl protons.
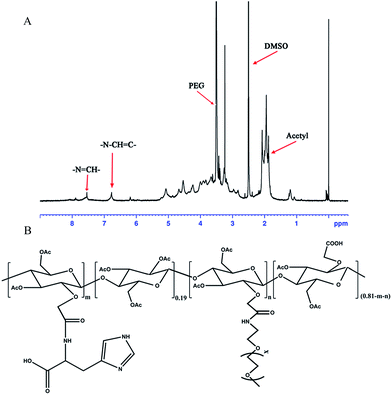 |
| Fig. 1 (A) 1H NMR spectrum of CMP copolymer. (B) The chemical structure of CMP copolymer. (m and n are the substitution degree of L-histidine and mPEG, respectively). | |
Polymers used for surface modification of NPs should have good biocompatibility. L929 cell line was recommended by many standard institutions as reference cell line for the cytotoxicity testing of polymers.31 Based on the result obtained from MTT assay, the cell viability of CMP polymer was more than 90% at all concentrations tested (50–600 μg mL−1), which implied that CMP copolymer showed neglect cytotoxic effect on normal cells.
3.2. Characterization of DTX-NLCs and DTX-CNLCs
This article firstly brought forward the concept of the application of amphiphilic CMP copolymer in surface modification of NLCs. The lipophilic portion of CMP was distributed on the lipid matrix, while the hydrophilic PEG component extended outwards into the external aqueous phase. As shown in Fig. 2, TEM images clearly illustrated the morphology of unmodified NLCs and CNLCs. Compared with bare NLCs (Fig. 2A), CNLCs exhibited a round shape coated with a light-colored layer (Fig. 2B). This finding confirmed that the CMP copolymer was successfully coated on the surface of NLCs. The lipid matrix of this novel nanocarrier, consisting of Compritol 888 ATO and LCT, was intended to accommodate hydrophobic drugs, while lecithin, F68 and sodium deoxycholate were used as emulsifiers to improve the colloidal stability.
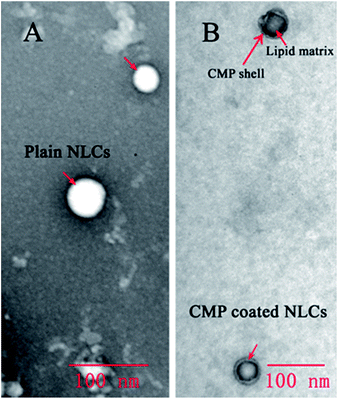 |
| Fig. 2 TEM images of NLCs (A) and CNLCs (B). | |
As shown in Table 1, the mean particle size of both formulations was between 80 and 90 nm, and the polydispersity was acceptable (<0.3). The slightly larger size of DTX-CNLCs was attributed to the attachment of the CMP shell. It is known that particle size and surface properties of NPs play essential roles in determining cellular uptake efficiency and biodistribution.32 NPs with a mean diameter of about 100 nm are suitable for excellent cellular uptake as well as for high drug accumulation at tumor site through the EPR effect.33 Hence, the carefully prepared DTX-CNLCs were in an effective range for intended applications. Zeta potentials of DTX-NLCs and DTX-CNLCs were both negatively-charged (Table 1), which was helpful to prevent the aggregation of NPs through electrostatic repulsion. The EE of DTX-NLCs and DTX-CNLCs was found to be 66.7 ± 4.3% and 64.1 ± 3.7%, respectively (p > 0.05), suggesting that the coating of CMP did not affect drug encapsulation.
Table 1 Characterization of DTX-NLCs and DTX-CNLCs. Values are mean ± SD (n = 3)
Formulation |
Size (nm) |
Polydispersity index (PDI) |
Zeta potential (mV) |
EE (%) |
DTX-NLCs |
81.2 ± 0.13 |
0.258 ± 0.01 |
−26.5 ± 1.47 |
66.7 ± 4.3 |
DTX-CNLCs |
88.7 ± 1.11 |
0.272 ± 0.01 |
−20.9 ± 1.11 |
64.1 ± 3.7 |
3.3. In vitro drug release
The release profiles of DTX from DTX-NLCs and DTX-CNLCs were studied by dialysis method in PBS at pH 7.4 (corresponding to the pH of blood) and 5.0 (corresponding to the pH of lysosomes),34 respectively. Results are presented in Fig. 3A. At pH 7.4, DTX-NLCs exhibited high initial burst release with a release of 12.7 ± 3.2% drugs within 1 h and nearly 57.6 ± 4.8% after 12 h, which might be caused by certain amount of drugs located near outer surface of the particles.35 In contrast, the burst release of DTX from DTX-CNLCs was restrained effectively at pH 7.4, with no more than 3.5 ± 0.6% of DTX released after 1 h (p < 0.01 compared with DTX-NLCs), and only about 32.8 ± 1.1% DTX was released within the initial 12 h (p < 0.01 compared with DTX-NLCs). In the following sustained phase, the accumulated amount of drug released from DTX-CNLCs was 63.9 ± 3.5% in 48 h. The release profile of DTX from CNLCs was fitted with five different model equations, including first kinetics, Higuchi, Baker–Lonsdale, Ritger–Peppas, and Hixcon–Crowell equations. The calculated correlation coefficient (r) was 0.9755, 0.9947, 0.9977, 0.9919, and 0.9807, respectively. The results demonstrated that the kinetics of DTX-CNLCs conformed well to Higuchi, Baker–Lonsdale, and Ritger–Peppas models. The mechanism of drug release mainly depended on the diffusion. The protective CMP shell around the lipid matrix increased diffusion distance of DTX, resulting in a better sustained-release pattern. Moreover, it should be noted that both formulations at pH 5.0 showed a much faster drug-release rate than those at pH 7.4 (p < 0.01), and there was no difference in the drug release at pH 5.0 between DTX-NLCs and DTX-CNLCs (p > 0.05) (Fig. 3A). After 6 h, the drug release percentage from DTX-NLCs and DTX-CNLCs was found to be 86.2 ± 5.8% and 85.6 ± 1.2% (p > 0.05), respectively. Taken together, the results implied that the CMP coating could improve the stability of DTX-CNLCs under physiological conditions, whereas it did not impair the drug release rate in the acidic lysosomes after internalized into cancer cells. This release performance could ensure effective intracellular drug delivery and excellent cytotoxicity of DTX in tumor cells.34,36
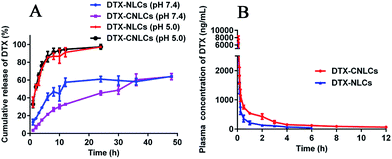 |
| Fig. 3 (A) In vitro DTX release from DTX-NLCs and DTX-CNLCs at pH 5.0 and pH 7.4 (n = 3). (B) Plasma concentration–time profiles in rats after intravenous administration of DTX-NLCs and DTX-CNLCs (n = 5). | |
3.4. Comparison of the pharmacokinetic profiles of DTX-NLCs and DTX-CNLCs
Fig. 3B shows the plasma concentration profiles of DTX after intravenous administration of DTX-NLCs and DTX-CNLCs to rats. In comparison to DTX-NLCs, the DTX-CNLCs blood circulation time was obviously extended (Fig. 3B). The corresponding pharmacokinetic parameters are summarized in Table 2. The plasma half-life (t1/2) of DTX-CNLCs was 2.24-fold longer than that of DTX-NLCs (p < 0.05). In addition, for the DTX-CNLCs, the AUC was 1.94-fold higher, MRT was 2.73-fold longer, and total body clearance (CL) was 2.0-fold lower than that for DTX-NLCs (p < 0.05). The improved pharmacokinetic profile of DTX-CNLCs could be the cause of the introduction of PEG segments in the shell and the superior sustained-release profile (Fig. 3A), which minimized interaction of drug with opsonins and protected DTX from rapid RES clearance.20,37
Table 2 Pharmacokinetic parameters in rats after intravenous administration of DTX-NLCs and DTX-CNLCs (mean ± SD, n = 5)
Parameters |
DTX-NLCs |
DTX-CNLCs |
p < 0.05 compared with the group treated with DTX-NLCs. |
AUC (h ng mL−1) |
2015 ± 211 |
3900 ± 345a |
MRT (h) |
0.791 ± 0.163 |
2.162 ± 0.054a |
t1/2 (h) |
2.169 ± 0.288 |
4.864 ± 0.675a |
CL (L h−1 kg−1) |
4.706 ± 0.562 |
2.355 ± 0.233a |
3.5. Cellular uptake assays
Although PEGylations are able to afford long circulation time of NLCs, there is a debate on whether the stealth agent could result in a negative effect on cellular uptake. Some studies showed that surface modification with PEGylated polymers could reduce cellular uptake and antitumor efficacy, which was possibly due to a decrease in nanoparticle cell interaction.38,39 Here we focused our attention on whether this CMP shell could hinder cell uptake of NLCs.
Firstly, cellular uptake efficiency of C6-NLCs and C6-CNLCs was evaluated by CLSM studies. The images obtained from each treatment at 1 h and 4 h are listed in Fig. 4A, in which C6 labeled NLCs and CNLCs were detected by the FITC channel. The nuclei were detected by the DAPI channel. All images showed the merged channels of FITC and DAPI channels. The outlines of the cells were clearly shown in the bright field. From Fig. 4A, it is clearly seen that DAPI stained nuclei (blue) were circumvented by green fluorescence in each treatment, indicating that both formulations could quickly enter into the cellular cytoplasm of cancer cells even after 1 h. The longer the incubation time, the stronger fluorescent intensity resulted, representing a time-dependent uptake manner. Flow cytometry was employed to further quantify the uptake levels of the two groups. Cells without C6 treatment were used as a control that showed the autofluorescence. As can be seen in Fig. 4B and C, the results were in accordance with the CLSM images. No obvious difference was found in cellular uptake efficiency between C6-CNLCs and C6-NLCs (p > 0.05). However, a significant difference was found after incubation for 1 and 4 h (p < 0.05). The results consistently demonstrated that the CMP shell did not disturb cellular uptake of NLCs in cancer cells, which might be attributed to their appropriate particle size and excellent particle cell interaction. Many studies prove that NPs mainly rely on the conventional endocytic pathways to enter cells.40 The nano-scaled size played a positive role in cellular uptake efficiency. In this study, both formulations were in an effective range for high cellular uptake. Furthermore, Ernsting et al.18 reported that the PEGylated carboxymethylcellulose conjugate of docetaxel could be internalized in a panel of tumor cell lines and exerted superior toxic effects. The finding seemed to imply that the PEGylated carboxymethylcellulose had good interaction with cell membrane of cancer cells, which also contributed to the uptake.
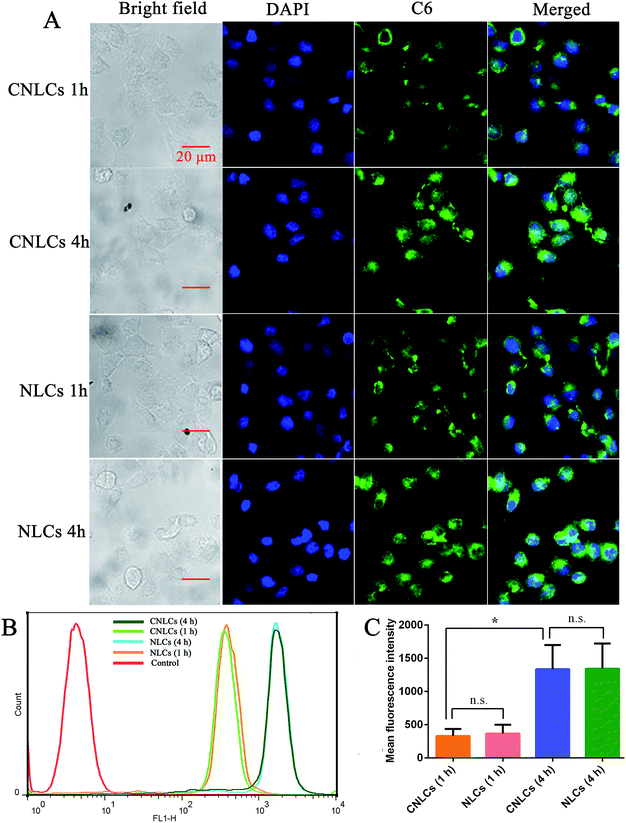 |
| Fig. 4 (A) Confocal microscopy images of MCF-7 cells incubated with C6-NLCs or C6-CNLCs at different times. Scale bars correspond to 20 μm in all the images. (B) Flow cytometry histograms of C6 accumulation in MCF-7 cells at 1 h and 4 h. (C) Flow cytometry analysis of MCF-7 cells incubated with different C6 formulations for 1 h and 4 h, respectively. *p < 0.05; n.s. p > 0.05. | |
3.6. Cytotoxicity
Cytotoxicity of DTX-CNLCs and DTX-NLCs was compared to assess the impact of this CMP shell on in vitro antitumor effect of drug-loaded NPs. The result of cytotoxicity against MCF-7 cells was displayed in Fig. 5. All formulations inhibited the growth of MCF-7 cells in a both time-dependent and dose-dependent manner. The CMP copolymer did not cause obvious cytotoxicity against MCF-7 cells even at 48 h incubation. From Fig. 5A and B, similar cytotoxicity in MCF-7 cells was found for DTX-CNLCs and DTX-NLCs. For example, the viability of MCF-7 cells after 24 h contact with 0.1, 1, 10 μg mL−1 DTX-CNLCs was 56.0 ± 6.8%, 54.0 ± 3.7%, and 45.6 ± 3.9%, respectively, whereas the one treated with DTX-NLCs was 58.8 ± 7.2%, 54.5 ± 3.3%, and 47.0 ± 2.6%, respectively (p > 0.05 between the two treatment groups). After 48 h of incubation, the viability of MCF-7 cells incubated with 0.1, 1, 10 μg mL−1 DTX-CNLCs was 29.1 ± 9.2%, 29.0 ± 6.5%, and 19.2 ± 0.4%, respectively, while the one treated with DTX-NLCs was 33.9 ± 1.5%, 31.2 ± 3.0%, and 20.9 ± 3.8%, respectively (p > 0.05 between the two treatment groups). IC50 was calculated in Table 3. MCF-7 cells exposed to DTX-CNLCs for 24 h or 48 h displayed IC50 values comparable to DTX-NLCs treated cells (p > 0.05). We concluded that this CMP modification could not reduce the cytotoxicity of DTX. On one hand, the two lipid NPs displayed similar uptake behavior (Fig. 4). On the other hand, after internalized by tumor cells, NLCs could quickly release DTX in acid environment (lysosomes), with or without CMP coating (Fig. 3A). From Fig. 5B, we also observed that the cytotoxicity of both NPs was much higher than that of DTX solution. After 48 h treatment, the IC50 of DTX-NLCs and DTX-CNLCs on MCF-7 cells was 170.9- and 151.9-fold lower than that of DTX solution (p < 0.01), respectively (Table 3). The significantly increased cytotoxicity of DTX was due to the better solubility of poorly water-soluble DTX after encapsulated into NLCs.41
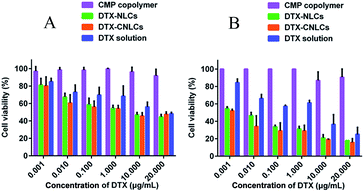 |
| Fig. 5 Cytotoxic effects of CMP copolymer, DTX-NLCs, DTX-CNLCs, and DTX solution on MCF-7 cells for 24 h (A) or 48 h (B). Data are means ± SD (n = 3). | |
Table 3 The IC50 values of DTX-NLCs, DTX-CNLCs and DTX solution on MCF-7 cells after treatment at different time (n = 3)
|
DTX-NLCs (μg mL−1) |
DTX-CNLCs (μg mL−1) |
DTX solution (μg mL−1) |
24 h |
1.957 ± 0.634 |
1.796 ± 0.351 |
13.45 ± 1.134 |
48 h |
0.016 ± 0.004 |
0.018 ± 0.009 |
2.735 ± 0.520 |
3.7. Evaluation of apoptosis
The ultimate goal of nanoparticle formulations of anticancer agents is to kill as many cancer cells as possible. In this study, the MCF-7 cell apoptosis rate was performed quantitatively by Annexin V-PI staining assay, as shown in Fig. 6. Annexin-V-FITC+/PI− (the lower right quadrant) cells were considered as early apoptotic cells, while Annexin-V-FITC+/PI+ (the upper right quadrant) cells were considered as late apoptotic cells. The induced cell apoptosis was calculated by counting the apoptotic percentage of the early and late periods. After 48 h incubation with DTX solution, DTX-NLCs, and DTX-CNLCs, the apoptotic percentage was determined to be 15.9 ± 0.44% (Fig. 6B), 40.9 ± 2.0% (Fig. 6C), and 42.6 ± 1.5% (Fig. 6D), respectively. These results revealed that DTX-NLCs and DTX-CNLCs exhibited comparable apoptosis rate at the same incubation condition (p > 0.05), whereas both nanoparticle groups induced more apoptosis than the free DTX group (p < 0.01). The findings were consistent with the observed results of in vitro cytotoxicity.
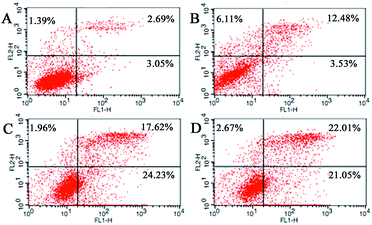 |
| Fig. 6 Cell apoptosis analysis of MCF-7 cells by flow cytometry using staining of AnnexinV-FITC and PI treated with blank medium (A), DTX solution (B), DTX-NLCs (C), and DTX-CNLCs (D) for 48 h. | |
3.8. In vivo antitumor effect
The results of antitumor effect in A549-bearing nude mice are shown in Fig. 7. From Fig. 7A, it is clearly observed that both DTX-based treatments significantly decreased the tumor growth compared to that in control (normal saline) (p < 0.01). More interestingly, the tumor volume treated with DTX-CNLCs was smaller than that treated with identical doses of unmodified DTX-NLCs (p < 0.05), indicating that the antitumor effect of DTX-CNLCs was stronger than that of DTX-NLCs. Fig. 7B displays the weight of the stripped tumors at the end of the treatment. The tumor inhibitory rate of DTX-NLCs and DTX-CNLCs was calculated to be 36.2% and 57.1% (p < 0.05), respectively. These findings illustrated that DTX-CNLCs exhibited more effective inhibition on established tumor growth than DTX-NLCs. We concluded that the introduction of CMP copolymer promoted anticancer activities of NLCs in the tumor-bearing mice. It was speculated that the higher antitumor activity of DTX-CNLCs was achieved by the following mechanism. Both CMP-modified NLCs and unmodified NLCs could passively accumulate in tumor site via the EPR effect. However, in comparison to the bare NLCs, the CMP coating not only decreased drug burst release (Fig. 3A), but also helped DTX possess a longer circulation time in vivo (Fig. 3B), which contributed to steady drug accumulation in tumors, resulting in a superior antitumor activity.
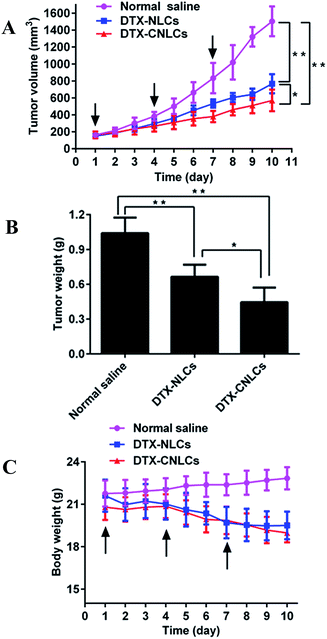 |
| Fig. 7 In vivo antitumor efficacy in A549-bearing nude mice treated with normal saline, DTX-NLCs, and DTX-CNLCs. (A) Tumor growth curves calculated by changes in tumor size. (B) The weight of the stripped tumors at the end of the treatments after 10 days observation. (C) Body weight changes for the tumor-bearing mice. Data are means ± SD (n = 5). *p < 0.05; **p < 0.01. | |
The changes in mice body weight against time were shown in Fig. 7C. Compared with the normal saline treated group, there was an appreciable body weight decrease rate treated with DTX-NLCs and DTX-CNLCs (less than 10%). There was no significant difference in mice body weight loss between the DTX-NLCs and DTX-CNLCs treated groups (p > 0.05), demonstrating good biocompatibility of the CMP copolymer.
In summary, the CMP shell in NLCs could increase antitumor effects. Due to the EPR effect, nanoparticles with a small size (<200 nm) have the ability to be passively accumulated in the areas of tumors.42 Generally, long circulation is necessary to provide sufficient time for drug accumulation in tumor sites. A sound strategy was implemented to conjugate PEG to the backbone of carboxymethylcellulose. The hydrophilic PEG segment is expected to provide protection from opsonization in blood circulation. As expected, the PEGylated carboxymethylcellulose copolymer on the surface of NLCs helped increase the retention time. On the other hand, the protective CMP shell around NLCs decreased drug burst release, which then prevented fast elimination of DTX. For these reasons, DTX-CNLCs could accumulate in tumor site constantly. Furthermore, the cytotoxicity and apoptosis studies provided sufficient evidence to elucidate that the CMP shell did not impair the ability of anticancer drugs to kill cancer cells. Based on the benefits of this CMP coating, therefore, DTX-CNLCs achieved superior antitumor activity in the tumor-bearing mice.
4. Conclusion
In this study, NLCs modified with the well-designed CMP copolymer exhibited attractive properties for DTX delivery. DTX-CNLCs achieved better sustained-release profile at pH 7.4 in vitro and possessed a longer circulation effect in vivo, in comparison to DTX-NLCs. Interestingly, this CMP modification did not affect the cellular uptake and cytotoxicity of NLCs. Finally, treatment with DTX-CNLCs resulted in an enhanced antitumor activity. Consequently, the CMP shell in NLCs is necessary to increase antitumor efficiency.
Notes and references
- R. H. Muller, M. Radtke and S. A. Wissing, Adv. Drug Delivery Rev., 2002, 54, S131–S155 CrossRef CAS.
- R. H. Muller, M. Radtke and S. A. Wissing, Int. J. Pharm., 2002, 242, 121–128 CrossRef CAS.
- M. J. Tsai, P. C. Wu, Y. B. Huang, J. S. Chang, C. L. Lin, Y. H. Tsai and J. Y. Fang, Int. J. Pharm., 2012, 423, 461–470 CrossRef CAS PubMed.
- D. H. Liu, Z. H. Liu, L. L. Wang, C. Zhang and N. Zhang, Colloids Surf., B, 2011, 85, 262–269 CrossRef CAS PubMed.
- L. J. Jia, D. R. Zhang, Z. Y. Li, F. F. Feng, Y. C. Wang, W. T. Dai, C. X. Duan and Q. Zhang, Drug Delivery, 2010, 17, 11–18 CrossRef CAS PubMed.
- X. Lin, R. C. Gao, Y. Zhang, N. Qi, Y. Zhang, K. Zhang, H. B. He and X. Tang, Expert Opin. Drug Delivery, 2012, 9, 767–781 CrossRef CAS PubMed.
- S. D. Li and L. Huang, Mol. Pharmaceutics, 2008, 5, 496–504 CrossRef CAS PubMed.
- F. Wan, J. You, Y. Sun, X. G. Zhang, F. D. Cui, Y. Z. Du, H. Yuan and F. Q. Hu, Int. J. Pharm., 2008, 359, 104–110 CrossRef CAS PubMed.
- S. Essa, J. M. Rabanel and P. Hildgen, Int. J. Pharm., 2011, 411, 178–187 CrossRef CAS PubMed.
- L. J. Jia, J. Y. Shen, D. R. Zhang, C. X. Duan, G. P. Liu, D. D. Zheng, X. N. Tian, Y. Liu and Q. Zhang, Int. J. Biol. Macromol., 2012, 50, 523–529 CrossRef CAS PubMed.
- X. Liu, Z. H. Zhang, Y. Q. Jiang, Y. Hu, Z. L. Wang, J. L. Liu, R. H. Feng, J. Zhang and G. H. Huang, Drug Delivery, 2015, 22, 223–229 CrossRef CAS PubMed.
- C. Zhao, T. Fan, Y. Yang, M. Wu, L. Li, Z. Zhou, Y. Jian, Q. Zhang and Y. Huang, Int. J. Pharm., 2013, 450, 11–20 CrossRef CAS PubMed.
- Z. H. Liu, Y. P. Jiao, Y. F. Wang, C. R. Zhou and Z. Y. Zhang, Adv. Drug Delivery Rev., 2008, 60, 1650–1662 CrossRef CAS PubMed.
- X.-Y. Ying, D. Cui, L. Yu and Y.-Z. Du, Carbohydr. Polym., 2011, 84, 1357–1364 CrossRef CAS PubMed.
- X. Y. Yang, Y. X. Li, M. Li, L. Zhang, L. X. Feng and N. Zhang, Cancer Lett., 2013, 334, 338–345 CrossRef CAS PubMed.
- L. Q. Yang, J. L. Kuang, J. Wang, Z. Q. Li and L. M. Zhang, Macromol. Biosci., 2008, 8, 279–286 CrossRef CAS PubMed.
- K. Zhu, T. Ye, J. Liu, Z. Peng, S. Xu, J. Lei, H. Deng and B. Li, Int. J. Pharm., 2013, 441, 721–727 CrossRef CAS PubMed.
- M. J. Ernsting, W. L. Tang, N. MacCallum and S. D. Li, Bioconjugate Chem., 2011, 22, 2474–2486 CrossRef CAS PubMed.
- M. J. Ernsting, M. Murakami, E. Undzys, A. Aman, B. Press and S. D. Li, J. Controlled Release, 2012, 162, 575–581 CrossRef CAS PubMed.
- M. J. Ernsting, W. L. Tang, N. W. MacCallum and S. D. Li, Biomaterials, 2012, 33, 1445–1454 CrossRef CAS PubMed.
- J. L. Wu, C. G. Liu, X. L. Wang and Z. H. Huang, J. Mater. Sci.: Mater. Med., 2012, 23, 1921–1929 CrossRef CAS PubMed.
- J. S. Park, T. H. Han, K. Y. Lee, S. S. Han, J. J. Hwang, D. H. Moon, S. Y. Kim and Y. W. Cho, J. Controlled Release, 2006, 115, 37–45 CrossRef CAS PubMed.
- J. J. Gu, X. Wang, X. Y. Jiang, Y. Z. Chen, L. C. Chen, X. L. Fang and X. Y. Sha, Biomaterials, 2012, 33, 644–658 CrossRef CAS PubMed.
- W. H. Jiang, L. Yang, L. P. Qiu, J. W. Xu, X. C. Yang, J. Wang, H. Zhoud and D. K. Wang, RSC Adv., 2015, 5, 53835–53845 RSC.
- M. T. Huizing, V. H. Misser, R. C. Pieters, W. W. ten Bokkel Huinink, C. H. Veenhof, J. B. Vermorken, H. M. Pinedo and J. H. Beijnen, Cancer Invest., 1995, 13, 381–404 CrossRef CAS.
- B. Nuijen, M. Bouma, J. H. Schellens and J. H. Beijnen, Invest. New Drugs, 2001, 19, 143–153 CrossRef CAS.
- X. Li, X. Tian, J. Zhang, X. Zhao, X. H. Chen, Y. H. Jiang, D. K. Wang and W. S. Pan, Int. J. Nanomed., 2011, 6, 1167–1184 CAS.
- M. S. Muthu, S. A. Kulkarni, J. Q. Xiong and S. S. Feng, Int. J. Pharm., 2011, 421, 332–340 CrossRef CAS PubMed.
- H. T. Pang, X. G. Chen, H. J. Park, D. S. Cha and J. F. Kennedy, Carbohydr. Polym., 2007, 69, 419–425 CrossRef CAS PubMed.
- H. J. Cho, I. S. Yoon, H. Y. Yoon, H. Koo, Y. J. Jin, S. H. Ko, J. S. Shim, K. Kim, I. C. Kwon and D. D. Kim, Biomaterials, 2012, 33, 1190–1200 CrossRef CAS PubMed.
- D. Fischer, Y. X. Li, B. Ahlemeyer, J. Krieglstein and T. Kissel, Biomaterials, 2003, 24, 1121–1131 CrossRef CAS.
- C. L. Lo, M. H. Chou, P. L. Lu, I. W. Lo, Y. T. Chiang, S. Y. Hung, C. Y. Yang, S. Y. Lin, S. P. Wey, J. M. Lo and G. H. Hsiue, Int. J. Pharm., 2013, 456, 424–431 CrossRef CAS PubMed.
- F. Alexis, E. Pridgen, L. K. Molnar and O. C. Farokhzad, Mol. Pharmaceutics, 2008, 5, 505–515 CrossRef CAS PubMed.
- L. P. Qiu, Z. Li, M. X. Qiao, M. M. Long, M. Y. Wang, X. J. Zhang, C. M. Tian and D. W. Chen, Acta Biomater., 2014, 10, 2024–2035 CrossRef CAS PubMed.
- M. Sun, S. F. Nie, X. Pan, R. W. Zhang, Z. Y. Fan and S. Wang, Colloids Surf., B, 2014, 113, 15–24 CrossRef CAS PubMed.
- Z. Li, L. P. Qiu, Q. Chen, T. N. Hao, M. X. Qiao, H. X. Zhao, J. Zhang, H. Y. Hu, X. L. Zhao, D. W. Chen and L. Mei, Acta Biomater., 2015, 11, 137–150 CrossRef CAS PubMed.
- S. M. Moghimi and J. Szebeni, Prog. Lipid Res., 2003, 42, 463–478 CrossRef CAS.
- X. P. Han, Z. B. Li, J. Sun, C. Luo, L. Li, Y. H. Liu, Y. Q. Du, S. H. Qiu, X. Y. Ai, C. N. Wu, H. Lian and Z. G. He, J. Controlled Release, 2015, 197, 29–40 CrossRef CAS PubMed.
- Y. Li, R. Liu, J. Yang, Y. Shi, G. Ma, Z. Zhang and X. Zhang, Biomaterials, 2015, 41, 1–14 CrossRef CAS PubMed.
- S. Martins, S. Costa-Lima, T. Carneiro, A. Cordeiro-da-Silva, E. B. Souto and D. C. Ferreira, Int. J. Pharm., 2012, 430, 216–227 CrossRef CAS PubMed.
- Y. Y. Chen, X. M. Yang, L. Zhao, L. Almasy, V. M. Garamus, R. Willumeit and A. H. Zou, Colloids Surf., A, 2014, 455, 36–43 CrossRef CAS PubMed.
- J. B. Huang, H. Zhang, Y. Yu, Y. Chen, D. Wang, G. Q. Zhang, G. C. Zhou, J. J. Liu, Z. G. Sun, D. X. Sun, Y. Lu and Y. Q. Zhong, Biomaterials, 2014, 35, 550–566 CrossRef CAS PubMed.
|
This journal is © The Royal Society of Chemistry 2015 |