DOI:
10.1039/C5RA18724A
(Paper)
RSC Adv., 2015,
5, 90226-90234
Synthesis and aggregation-induced emission properties of pyridine and pyridinium analogues of tetraphenylethylene†
Received
12th September 2015
, Accepted 14th October 2015
First published on 15th October 2015
Abstract
Aggregation-induced emission (AIE) is emerging as an important design element in a variety of new fluorescent-based chemical sensors and bio-imaging agents. In particular, derivatives of tetraphenylethylene (TPE) have been widely utilized in this regard as the TPE framework is a reliable AIE-luminogen. To expand the library of AIE active tetraarylethylenes, we have explored the effects of replacing one or two of the phenyl rings in TPE with pyridine. Efficient synthetic routes that deliver mono- and bis-pyridyl tetraarylethylenes have been developed and the luminescent properties of these heterocyclic TPE analogues, along with their corresponding N-methylated pyridinium salts, have been examined.
Introduction
Organic fluorophores play important roles in chemical biology and materials science. Conjugation of organic dyes to various biomolecules and ligands provides a means to probe enzymatic and cellular processes using fluorescence spectroscopy.1 Likewise, organic fluorophores are fundamental components in several types of functional materials, such as organic light-emitting diodes (OLED) and fluorescent sensors.2
Conventional organic fluorophores typically operate most efficiently under high-dilution conditions, and fluorescence quenching is observed in concentrated solution and the solid state. This feature limits the conditions under which most fluorescent organic compounds can be effectively utilized. Certain classes of organic molecules, however, display the opposite behavior. These compounds exhibit little to no fluorescence in dilute solution, but become markedly more fluorescent when aggregated or in the solid state. The term “aggregation induced emission” (AIE) has been introduced by Tang to describe this behavior, and compounds exhibiting this property are said to be AIE-active fluorophores.3
Although several different types of compounds have been identified as AIE active, the most widely used AIE luminogens in bio- and materials science are derivatives of tetraphenylethylene (TPE, 1, Fig. 1).4 Functionalized TPEs are often non-emissive when dissolved in good solvents, but display a fluorescent signal when combined with poor solvents or upon precipitation/crystallization. The basis for this fluorescence response is believed to reside in restriction of intramolecular motion (e.g., rotations about the phenyl–ethylene bonds) that occurs upon aggregation.3b,5 This property of TPEs has been harnessed as the operative design element in a number of chemical and biochemical fluorescent sensors and detectors.3,4 In particular, incorporation of one or more pyridine rings into the periphery of the TPE core has produced several intriguing AIE-active materials. For example, an N-benzylated derivative of (4-pyridyl)TPE 2 possessing a reactive boronic ester exhibits fluorescence turn-on detection of H2O2 and glucose in aqueous solution.6 Additionally, N-methylpyridinium salts of 2 have been shown to display tunable emission in the solid state as a function of counteranion.7 The 4-vinylpyridyl-functionalized TPE 3 has shown efficacy as a sensing agent for trivalent cations (e.g., Fe3+),8 while simple alkylpyridinium salts prepared from 3 show selective fluorescence response in the presence of pyrophosphate anion.9 Other derivatives of 3 have been used for fluorescence bioimaging (e.g., monitoring of targeted drug delivery and cell apoptosis)10 as well as mitochondrial staining.11 Bis(4-vinylpyridyl)TPE 4 has been used as monomer in construction of polycationic polymers for detection of heparin,12 while the dimethylpyridinium salt displays interesting mechanochromic properties.13 Finally, the tetra(4-pyridyl)TPE 5 has been employed in a number of AIE active constructs, including pH sensitive fluorescence indicators14 and supramolecular polymers.15 TPE 5 also exhibits selective detection of Hg(HSO4)2,16 and has been demonstrated to be an excellent halogen bond acceptor in mediating assembly of crystalline fluorescent halogen bonded networks with iodoarenes.17 The tetratopic array of pyridine groups in 5 has also been harnessed in construction of metal–organic as well as main group-organic coordination polymers,18 and in assembly of dynamic supramolecular coordination complexes.19
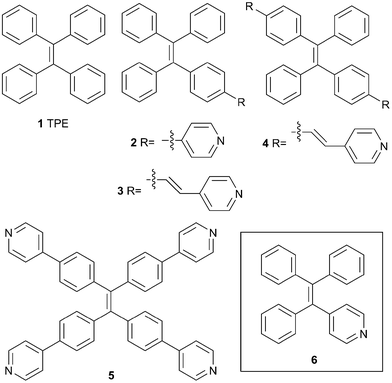 |
| Fig. 1 Examples of pyridine-functionalized TPEs (2–5). | |
A common feature shared by TPE derivatives 2–5 is the attachment of one or more pyridine rings to the periphery of the TPE core. In part, this reflects the accessibility of functionalized TPEs to which heterocyclic arenes (such as pyridine or vinylpyridine) can be readily attached. Only a handful of tetraarylethylene (TAE) analogues of TPE in which one of the core phenyl rings has been replaced with pyridine (e.g., 6) have been reported, and the photophysical properties of these compounds have not been examined.20 Indeed, examples of tetraarylethylenes with other heterocyclic arenes (such as furan, thiophene, and pyrrole) are also rare.21 Given the broad utility of pyridyl-functionalized TPEs 2–5 in diverse areas of supramolecular chemistry, it is anticipated that compounds such as 6 and closely related congeners also should exhibit desirable fluorescent properties while retaining considerable synthetic flexibility for additional elaboration. Moreover, direct replacement of phenyl with pyridine results in TAE derivatives with smaller molecular footprints relative to 2–5 and potentially greater hydrophilicities, issues that are important in biological applications. Thus, we report here a new synthesis of 6 along with several other mono- and bis(pyridyl) tetraarylethylenes. Since much of the utility of 2–5 derives from N-alkylated derivatives, pyridyl tetraarylethylenes prepared in this study were also converted to their associated N-methylpyridinium tetrafluoroborate salts. These novel heterocyclic TPE-inspired materials have been further characterized by fluorescence spectroscopy.
Results and discussion
To access the initial targets of this investigation (triphenyl vinylpyridines 6–8 or TPVPs) we adopted an approach that has proven successful in construction of conventional tetraphenylethylenes. As illustrated in Scheme 1, the lithium anion derived from trimethylsilyl-substituted diphenylmethane was condensed with a series of isomeric phenyl pyridyl ketones (pyridyl = 4-pyridyl, 3-pyridyl, or 2-pyridyl) to effect Peterson olefination.22 The TPVP derivatives 6–8 were obtained in comparable yields. Notably, condensation of diphenylmethane with the same phenyl pyridyl ketones followed by acid-catalyzed (p-TSA) dehydration of the putative carbinol products delivered 6–8 in significantly lower yields.23 The presence of the desired pyridine-containing TAE products was qualitatively indicated by the appearance of long wavelength fluorescent material in TLC analysis of crude reaction mixtures. Each TPVP derivative was then converted to the corresponding N-methylpyridinium salt in good yield.
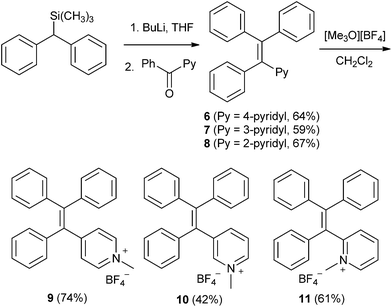 |
| Scheme 1 Synthesis of TPVPs 6–8 and their corresponding N-methylpyridinium salts. | |
The preparative route shown in Scheme 1 works well for construction of unsubstituted TPVPs, however, we found an alternate route involving Suzuki coupling to be more effective for preparation of substituted TPVPs bearing electron donating groups (e.g., OMe) as illustrated in Scheme 2. 4-Pyridyl phenyl ketone 12 was converted to the geminal dibromoalkene 13 by treatment with CBr4 and PPh3. Double Suzuki coupling was then used to install methoxyphenyl groups in the tetraarylethylene framework, affording 14 in good overall yield. Although not typically used to construct tetraarylethylenes, the advantages of similar Suzuki coupling strategies in accessing unsymmetrical tetraphenylethylenes that would be difficult to prepare by other methods has been noted.24 The pyridyl group in 14 was also easily methylated to give 15.
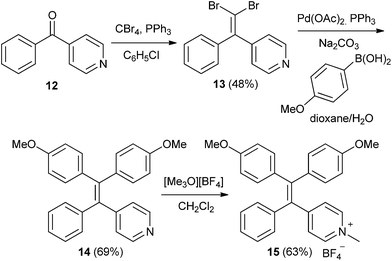 |
| Scheme 2 Double Suzuki coupling for synthesis of substituted TPVP derivatives. | |
Double Suzuki coupling was also employed to construct two representative examples of 1,1-diphenyl-2,2-dipyridylethylenes (DPDPyEs). In these examples the known dibromoalkene25 16 was coupled with either 3- or 4-pyridyl boronic acid (Scheme 3). Coupling proceeded smoothly in each case, and the DPDPyE derivatives 18 and 19 were isolated in serviceable yields. Treatment of each dipyridylethylene with an excess of [Me3O][BF4] in methanol afforded the bis(pyridinium) salts 20 and 21.
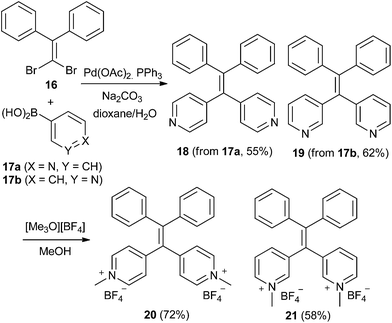 |
| Scheme 3 Synthesis of diphenyl dipyridyl ethylenes (DPDPyEs). | |
X-Ray quality single crystals of two compounds described above (14 and 18) were obtained from slow evaporation of EtOAc/MeOH solutions.26 Their respective molecular structures are shown in Fig. 2. In each case the four aryl rings are arrayed in propeller-like fashion around the central ethylene linkage. The torsion angles of the four arenes with respect to the ethylene bond in 14 range from ∼44° to ∼62°, while the range of torsion angles in 18 is somewhat narrower (51–61°). In 14, individual molecules are part of extended helical assemblies, facilitated in part by solid state C–H⋯N hydrogen bonding between the pyridine nitrogen and a C–H group from a methoxy substituent (see ESI†). The extended packing in 18 features an ABAB-type layer structure down c with adjacent interlayer DPDPyE molecules rotated ∼180° (ESI†).
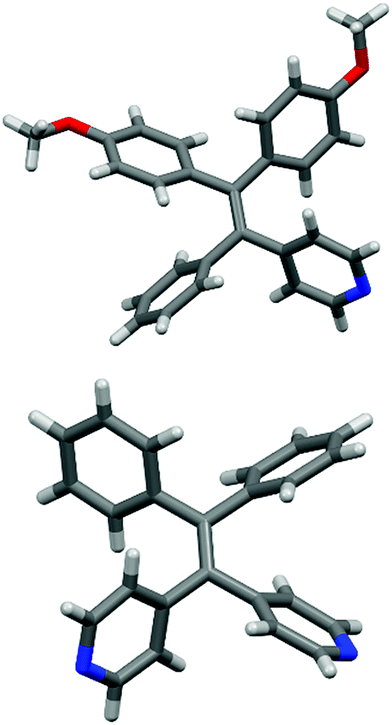 |
| Fig. 2 Molecular structure of TPVP 14 (left) and DPDPyE 18 (right). | |
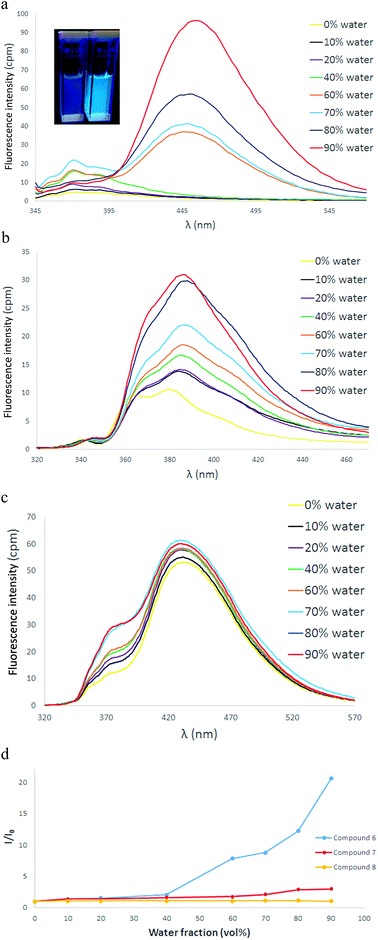 |
| Fig. 3 (a) Fluorescence spectra of 6 in CH3CN/H2O mixture. Inset: picture taken under UV light of 6 in 100% CH3CN (left) and 90 : 10 H2O : CH3CN (right). (b) Fluorescence spectra of 7 in CH3CN/H2O mixture. (c) Fluorescence spectra of 8 in CH3CN/H2O mixture. (d) Plot of relative emission intensity (I/Io) vs. H2O fraction (vol%) for 6–8. Io = emission intensity in 100% CH3CN. Sample concentration 10 μM and λex = 305 nm in each case. | |
The TPVPs 6–8 exhibited similar UV-visible absorption spectra in CH3CN solution. Each compound displayed a relatively featureless absorption spectrum with longest wavelength absorption at ∼340 nm (see ESI†). In contrast, 6–8 did display significant differences in their fluorescence spectra under conditions leading to aggregation induced emission. For example, the fluorescence spectrum of 6 in 100% CH3CN (a medium in which 6 is readily soluble) revealed only minimal emission. However, incremental addition of H2O (a poor solvent for 6) induced a markedly red-shifted fluorescence response with λem ∼ 455 nm (Fig. 3a). Aggregation induced emission effects observed in isomeric TPVPs 7 and 8 under similar conditions (CH3CN/H2O) were much less pronounced (Fig. 3b and c), and the λmax,em of 3-pyridyl isomer 7 appears blue-shifted compared to the 4- and 2-pyridyl analogues 6 and 8. This presumably reflects the more pronounced electron-withdrawing abilities of the substituted pyridines in these latter two TPVPs. The differences in AIE effect among TPVP isomers is further illustrated by comparing plots of relative fluorescence enhancement (I/Io) as a function of water fraction (fH2O) in CH3CN solvent. As illustrated in Fig. 3d, 4-pyridyl-TPVP 6 displays the greatest aggregation-induced fluorescence enhancement, while the 2-pyridyl derivative 8 exhibits only minimal changes in fluorescence intensity. Clearly, the position of the nitrogen atom within the TPVP framework influences the luminescent properties of these materials. The most noticeable departure from typical AIE behavior is exhibited by 2-pyridyl isomer 8, as this material displays essentially constant fluorescence irrespective of aggregation state. We speculate that replacement of a C–H group with the pyridine nitrogen atom may result in reduced steric interactions between the 2-pyridyl ring and adjacent phenyl rings, in turn leading to greater pyridine–ethylene π-orbital overlap and fluorescence emission from both monomers and aggregated oligomers.
Several pyridinium-substituted TPE's derived from neutral precursors 2–5 (Fig. 1) have been found to possess interesting photoluminescent properties,7,9,11,13 thus, TPVPs 6–8 were each converted to pyridinium salts to probe the effects of pyridinium ion formation on absorbance and emission in this system. Conversion of the neutral TPVP isomers 6–8 to the corresponding N-methyl pyridinium tetrafluoroborate salts 9–11 was achieved in straightforward fashion upon treatment with [Me3O][BF4]. The longest-wavelength absorptions in the UV/visible spectra of 9–11 were all slightly red-shifted relative to their neutral counterparts (see ESI†). The altered absorption was most pronounced in the 4- and 2-pyridyl TPVP salts 9 and 11, which displayed longest-wavelength absorption at 363 nm and 369 nm, respectively. The effect of pyridine alkylation on the emission properties of 9–11, however, was more significant. These compounds maintained fluorescence in the solid (aggregated) state as readily determined qualitatively by observing the emission of solid samples placed under a UV light. These materials also exhibited fluorescence emission in dilute solution, and the relative intensity of the fluorescence signal was virtually unaltered upon aggregation in each case. The emission spectra of 11 are representative (Fig. 4). This salt is readily dissolved in CH2Cl2 and the solution phase fluorescence spectrum revealed an emission signal at λmax,em = 456 nm. Incremental addition of hexane (a poor solvent for 11) to the CH2Cl2 solution produced a series of emission spectra that were almost identical to the original.27 The isomeric pyridinium salts 9 and 10 exhibited similar luminescent properties in CH2Cl2/hexane solution (see ESI†). It seems that the AIE/AIEE effect has been switched off in tetraarylethylene derivatives 9–11 in favor of more or less constant fluorescence response irrespective of aggregation state. Thus, the neutral compounds 6–8 and their pyridinium derivatives 9–11 display two distinct fluorescent signatures.
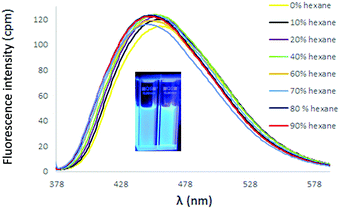 |
| Fig. 4 Fluorescence spectra of 11 in CH2Cl2 and CH2Cl2/hexane mixtures ([11] = 10 μM, λex = 369 nm). Inset: picture taken under UV light of 11 in 100% CH2Cl2 (left) and 90 : 10 hexane : CH2Cl2 (right). | |
The dimethoxy analogue 14 was prepared to help demonstrate the versatility of double-Suzuki reactions for the preparation of functionalized tetraarylethylenes. Additionally, incorporation of two 4-methoxyphenyl groups into the TPVP framework provides a derivative electronically distinct from the parent TPVP 6. Consistent with the greater conjugation in 14, the longest-wavelength absorption in the UV/visible spectrum was slightly red-shifted by ∼23 nm (λmax = 328 nm, see ESI†) compared to 6. The AIE properties of 14 were also examined in acetonitrile/H2O solution, and the associated spectra are illustrated in Fig. 5a. This material exhibited a fluorescence signal at λmax,em = 384 nm in pure CH3CN. Incremental addition of H2O initially produced an enhanced fluorescence emission at the same wavelength up to 60% H2O. Further addition of H2O, however, resulted first in slight bathochromic shift of the emission wavelength, followed by diminished emission at 404 nm with concomitant increase in emission at significantly longer wavelength (490 nm). Such behavior in AIE-active systems may be attributed to subtle changes in the way that individual molecules are associated as a function of aggregation and solvent polarity.11b,28 Red-shifted emission of 14 in solutions with high water content may reflect enhanced conjugation between the methoxyphenyl groups and the electron withdrawing pyridine ring. Additionally, longer wavelength emission upon increased aggregation may indicate charge transfer-type interactions between the electron-deficient pyridine ring and the electron-rich methoxyphenyl groups. Alkylation of the pyridine nitrogen gave the corresponding pyridinium salt (15). Not surprisingly, this salt exhibited a significant bathochromic shift in the absorption spectrum compared to the neutral precursor (λmax = 441 nm). Pyridinium 15 also displayed observable fluorescence in dilute CH2Cl2 solution (Fig. 5b), as was the case with pyridinium TPVPs 9–11. In contrast to 9–11, however, incremental addition of hexane resulted in aggregation-caused quenching (ACQ) of this emission, in line with behavior observed for most organic fluorophores. We speculate that the combination of increased electron density in the methoxyphenyl groups with the known ability of N-alkylpyridinium salts to quench fluorescence of aromatic hydrocarbons may be responsible for this effect.29
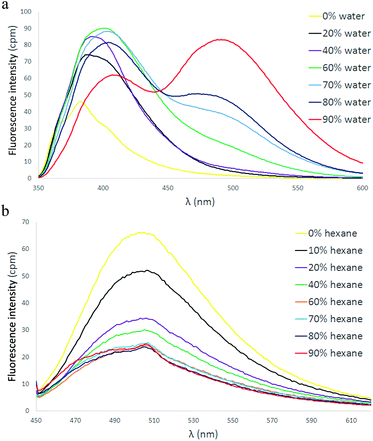 |
| Fig. 5 (a) AIE effect revealed in fluorescence spectra of 14 in CH3CN/H2O mixtures ([14] = 10 μM, λex = 328 nm). (b) ACQ effect shown in fluorescence spectra of 15 in CH2Cl2/hexane mixtures ([15] = 10 μM, λex = 442 nm). | |
A switch to ACQ behavior was also observed in the fluorescence spectra of bis(pyridinium) salts 20, 21. While the neutral DPDPyE precursors 18 and 19 both displayed the expected AIE luminescence, conversion to the corresponding dications afforded salts that displayed fluorescence in dilute solution that became quenched upon aggregation. The fluorescence spectra of 19 and 21 shown in Fig. 6 provide representative illustrations of these phenomena.30 The neutral DPDPyE 19 exhibits an AIE effect in CH3CN/H2O (Fig. 6a), while the initial fluorescence of the corresponding bis(pyridinium) salt 21 in CH2Cl2 is nearly completely quenched upon aggregation in 90% hexane. We ascribe the fluorescence quenching observed in 21 to the presence of two electron deficient N-methylpyridinium groups within the TAE framework, since incorporation of a single N-methylpyridinium group, as in 9–11, fails to quench the inherent fluorescence. Similar spectra were observed in the 4-pyridyl isomers 18 and 20 (ESI†).
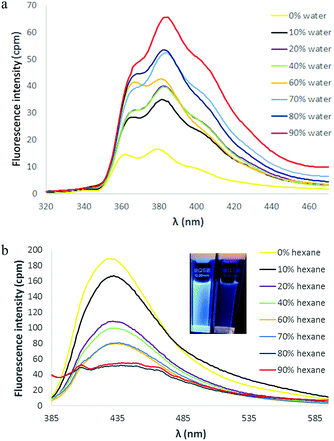 |
| Fig. 6 (a) AIE effect in fluorescence spectra of DPDPyE 19 in CH3CN/H2O mixtures ([19] = 10 μM, λex = 305 nm). (b) ACQ effect in fluorescence spectra of DPDPyE pyridinium salt 21 in CH2Cl2/hexane mixtures ([21] = 10 μM, λex = 363 nm). Inset: picture taken under UV light of 21 in 100% CH2Cl2 (left) and 21 in 90 : 10 hexane : CH2Cl2 (right). | |
A final class of TPVP derivative prepared and characterized as part of this study is shown in Scheme 4 and features two TPVP scaffolds linked through a common alkyl chain. Construction of 24 and 25 was easily achieved through prolonged heating of 6 and either 1,3-dichloropropane or 1,10-dibromodecane (2
:
1 molar ratio 6
:
dihaloalkane) in CH3CN. Potassium iodide was added to increase the reactivity of the haloalkane. The bis(TPVP) derivatives were ultimately isolated by column chromatography as iodide salts in good yield. The UV spectra of 24 and 25 both exhibited red-shifted longest wavelength absorptions relative to the TPVP precursor 6, with 24 exhibiting a more pronounced shift (λabs = 390 nm) than 25 (λabs = 369 nm). The emission spectra in CH2Cl2 of both 24 and 25 revealed a fluorescence signal at similar λmax (∼459 nm) that was completely quenched upon addition of hexane, most likely due to the presence of iodide counterions.31 Indeed, conversion of these linked TPVPs to the corresponding PF6 salts 26 and 27 produced a fluorescence response in CH2Cl2/hexane that varied according to length of alkyl spacer. As shown in Fig. 7, addition of hexane to a CH2Cl2 solution of 26 (three-carbon linker) elicited a diminution of fluorescence intensity (accompanied by a slight blue shift), similar to the behaviour of DPDPyE salts. In contrast, the fluorescence spectra of 27 (ten-carbon linker) in CH2Cl2 did not dramatically change upon addition of hexane, similar to the behaviour of N-methylpyridinium TPVP salt 9 (ESI†).
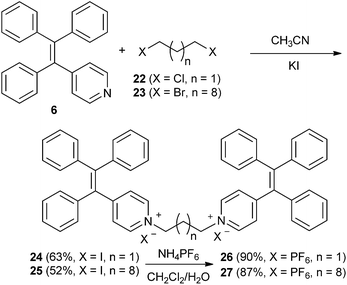 |
| Scheme 4 Synthesis of alkyl-linked TPVP salts. | |
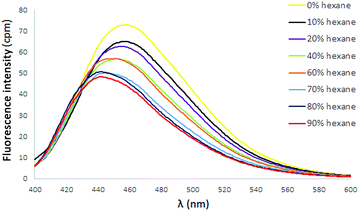 |
| Fig. 7 Fluorescence spectra of 26 in CH2Cl2/hexane mixtures ([26] = 10 μM, λex = 390 nm). | |
Conclusions
We have developed effective synthetic routes to novel tetraarylethylenes in which one or two of the core aryl rings have been replaced with heterocyclic (pyridine) groups. The tactics used to prepare these pyridine-containing TAEs also should be broadly applicable to the synthesis of other heterocyclic tetraarylethylenes incorporating alternative heteroarenes. The TPVP and DPDPyE derivatives 6–8, 14, and 18, 19 function as AIE-luminogens, in keeping with their similarity to the prototypical AIE active compound tetraphenylethylene. Conversion of these materials to N-methyl pyridinium salts, however, results in divergent fluorescent properties. Specifically, mono-pyridinium salts 9–11 exhibit observable luminescence in both dilute solution and upon aggregation, while mono-pyridinium 15 and bis(pyridinium) salts 20, 21 experience aggregation-caused quenching. Likewise, linking two TPVP frameworks with a short alkyl spacer also produces a fluorescent pyridinium salt that exhibits diminished emission upon aggregation. The ACQ effect in bis(TPVP) salts, however, is attenuated upon increasing the length of the alkyl spacer. The departure of these pyridinium-TAE salts from the typical AIE activity associated with closely related compounds indicates that additional factors other than restriction of intramolecular bond rotations influence the luminescence of these compounds. Nonetheless, the diverse fluorescence signatures observed in these pyridine/pyridinium-TAEs offer opportunities for developing new sensors and imaging agents based on turn-on or turn-off luminescence response. Currently, we are developing applications of (heteroaryl)arylethylenes along these lines while also examining the synthesis of additional heterocyclic analogues of TPE.
Experimental
General
All commercially available starting materials, reagents, and solvents were used as supplied, unless otherwise stated. Reactions were performed under inert atmosphere using solvents that were dried and purified by passage through activated alumina or activated molecular sieves. Reported yields are isolated yields. Purification of all final products was accomplished by silica gel flash column chromatography. Chloroform
:
methanol or hexane
:
ethyl acetate were used as elution solvents. Proton (1H) and carbon (13C) NMR were collected on Bruker NMR spectrometers at 300 MHz or 400 MHz for 1H and 100 MHz or 75 MHz for 13C. Chemical shifts (δ) are reported in parts-per million (ppm) relative to tetramethylsilane or residual undeuterated solvent. Melting points were recorded using a capillary melting point apparatus and are uncorrected. High resolution mass spectra were obtained in positive ion mode using electron spray ionization (ESI) on a double-focusing magnetic sector mass spectrometer. UV-visible spectra were obtained using quartz cuvettes on a Varian Cary 100-Scan dual-beam spectrophotometer. Each measurement was done in duplicate and compared to solvent blank. Blank samples were prepared using HPLC grade acetonitrile or methylene chloride. Fluorescence spectra were obtained in air at room temperature using a Horiba Jobin Yvon Fluoromax-4 spectrofluorimeter using 3 mL quartz cuvettes. Samples were prepared using HPLC grade solvents. X-Ray diffraction data were collected on a Nonius Kappa CCD diffractometer equipped with Mo Kα radiation with λ = 0.71073 Å. Structures were solved by direct methods and data was refined by full-matrix least-squares refinement on F2 against all reflections.
Synthetic procedures
4-(1,2,2-Triphenylvinyl)pyridine (6). A solution of benzhydryl(trimethyl)silane (2.88 g, 12.0 mmol, prepared according to the reported procedure22) in 30 mL THF was cooled to 0 °C and n-BuLi (2.5 M solution in hexane, 4.0 mL, 10 mmol) was added via syringe. The resulting dark red solution was stirred at 0 °C for 2 h. 4-Benzoylpyridine (1.65 g, 9.00 mmol) in 20 mL THF was added slowly to the reaction mixture. The reaction was then allowed to warm to room temperature and maintained overnight. The reaction was quenched with methanol and the solvent was evaporated to furnish an oily residue. The crude product was purified by flash column chromatography using ethyl acetate/hexane as eluent to yield TPVP 6 (1.92 g, 64%) as an off-white solid. Mp 199–201 °C. 1H NMR (300 MHz, CDCl3) δ 6.91–7.15 (m, 17H), 8.34 (d, 2H, J = 5.9 Hz). 13C NMR (100 MHz, CDCl3) δ 127.1, 128.0, 128.1, 128.3, 128.8, 129.0, 129.1, 131.1, 132.2, 132.3, 139.3, 143.4, 143.7, 143.9, 144.7, 150.4, 152.8. HRMS (ESI): calcd for C25H20N [M + H]+, 334.1596; found, 334.1599.
3-(1,2,2-Triphenylvinyl)pyridine (7). Using the procedure given above on 1.65 g (9.00 mmol) of 3-benzoylpyridine, TPVP 7 (1.77 g, 59%) was obtained initially as a reddish solid that was de-colorized by treatment with activated charcoal to afford a white solid. Mp 156–159 °C. 1H NMR (300 MHz, CDCl3) δ 7.09–7.41 (m, 17H), 8.31 (s, 1H), 8.37 (d, 1H, J = 5.1 Hz). 13C NMR (75 MHz, CDCl3) δ 125.3, 129.4, 129.5, 129.6, 130.4, 130.6, 130.7, 131.1, 133.8, 133.9, 134.0, 139.9, 140.9, 145.4, 145.5, 145.6, 145.7, 149.9, 154.6. HRMS (ESI): calcd for C25H20N [M + H]+, 334.1596; found, 334.1590.
2-(1,2,2-Triphenylvinyl)pyridine (8). Using the procedure given above on 1.65 g (9.00 mmol) of 2-benzoylpyridine, TPVP 8 (2.01 g, 67%) was obtained as a white solid. Mp 178–181 °C. 1H NMR (300 MHz, DMSO-d6) δ 6.96–7.22 (m, 16H), 7.53–7.61 (m, 2H), 8.45 (d, 1H, J = 5.4 Hz). 13C NMR (75 MHz, CDCl3) δ 123.9, 129.1, 129.3, 129.4, 129.5, 130.3, 130.4, 130.6, 130.9, 133.7, 133.9, 138.1, 143.3, 144.8, 145.6, 145.8, 145.9, 152.1, 164.8. HRMS (ESI): calcd for C25H20N [M + H]+, 334.1596; found, 334.1598.
1-Methyl-4-(1,2,2-triphenylvinyl)pyridinium tetrafluoroborate (9). Trimethyloxonium tetrafluoroborate (73 mg, 0.5 mmol) was added to a solution of 6 (100 mg, 0.3 mmol) in 25 mL of CH2Cl2 at 0 °C. The reaction was then allowed to warm to room temperature and monitored by TLC until disappearance of starting material was complete. The solvent was evaporated under reduced pressure and the residue was purified by flash column chromatography using methanol as eluent to give 96.5 mg (74%) of 9 as a yellow solid. Mp 133–134 °C. 1H NMR (300 MHz, CDCl3) δ 4.28 (s, 3H), 7.02–7.41 (m, 17H), 8.37 (d, 2H, J = 6.2 Hz). 13C NMR (100 MHz, CDCl3) δ 48.9, 129.0, 129.2, 129.4, 129.9, 130.1, 130.2, 130.7, 132.1, 132.4, 132.5, 136.3, 141.5, 141.8, 142.4, 144.9, 151.4, 162.3. HRMS (ESI): calcd for C26H22N [M]+, 348.1752; found, 348.1766.
1-Methyl-3-(1,2,2-triphenylvinyl)pyridinium tetrafluoroborate (10). Using the procedure given for the preparation of 9, 7 (100 mg, 0.3 mmol) was converted to 10 (54.8 mg, 42%). Mp > 200 °C. 1H NMR (300 MHz, acetone-d6) δ 3.87 (s, 3H), 7.31–7.68 (m, 15H), 8.29 (t, 1H), 8.61 (d, 1H, J = 6.9 Hz), 8.87 (s, 1H), 9.03 (d, 1H, J = 6.0 Hz). 13C NMR (100 MHz, acetone-d6) δ 48.8, 128.1, 128.6, 128.7, 128.9, 129.5, 129.6, 131.7, 132.2, 132.3, 134.9, 135.4, 140.4, 142.1, 142.5, 143.0, 144.2, 145.0, 147.8, 150.0. HRMS (ESI): calcd for C26H22N [M]+, 348.1752; found, 348.1758.
1-Methyl-2-(1,2,2-triphenylvinyl)pyridinium tetrafluoroborate (11). Using the procedure given for the preparation of 9, 8 (100 mg, 0.3 mmol) was converted to 11 (79.6 mg, 61%). Mp 150–153 °C. 1H NMR (300 MHz, acetone-d6) δ 4.36 (s, 3H), 7.19–7.34 (m, 16H), 7.97–8.15 (m, 2H) 8.78 (d, 1H, J = 5.1 Hz). 13C NMR (75 MHz, acetone-d6) δ 49.8, 130.7, 130.8, 131.1, 131.3, 131.4, 132.4, 133.2, 133.4, 133.5, 133.6, 133.9, 141.5, 143.4, 143.7, 144.4, 147.8, 149.4, 153.8, 158.1. HRMS (ESI): calcd for C26H22N [M]+, 348.1752; found, 348.1755.
4-(2,2-Dibromo-1-phenylvinyl)pyridine (13). 4-Benzoylpyridine (182 mg, 1.00 mmol) was dissolved in chlorobenzene (50 mL). Carbon tetrabromide (662 mg, 2.00 mmol) and PPh3 (1.05 g, 4.00 mmol) were added and the reaction mixture was heated to reflux and maintained for 3 d. After this time the reaction mixture was allowed to cool to rt and insoluble material was removed by filtration. The filtrate was treated with 50 mL of 1 M aq. HCl. The aqueous layer was separated and basified with 1 M aq. NaOH until pH 12. The aqueous phase was extracted with CH2Cl2 (2 × 50 mL), and the combined organic fractions were dried over anhydrous Na2SO4, filtered, and concentrated under reduced pressure. The crude product was purified by flash column chromatography using ethyl acetate/hexane as eluent to yield 13 (162 mg, 48%) as a buff solid. Mp 109–112 °C. 1H NMR (300 MHz, CDCl3) δ 7.26–7.43 (m, 7H), 8.65 (d, 2H, J = 5.2 Hz). 13C NMR (100 MHz, CDCl3) δ 93.4, 124.5, 129.6, 129.7, 129.8, 141.1, 146.4, 149.9, 151.1. HRMS (ESI): calcd for C13H10Br2N [M + H]+, 337.9180; found, 337.9182.
4-(2,2-Bis(4-methoxyphenyl)-1-phenylvinyl)pyridine (14). Compound 13 (338 mg, 1.00 mmol) was dissolved in 50 mL of dioxane
:
water (4
:
1). The flask was charged with Na2CO3 (690 mg, 5.00 mmol), Pd(OAc)2 (28 mg, 0.12 mmol), PPh3 (130 mg, 0.50 mmol), and 4-methoxyphenylboronic acid (760 mg, 5.00 mmol). The reaction mixture was heated to reflux overnight. After cooling to rt, the reaction mixture was extracted with ethyl acetate (3 × 50 mL) and the combined organic fractions were dried over anhydrous Na2SO4, filtered, and concentrated under reduced pressure. The crude product was purified by flash column chromatography using ethyl acetate/hexane as eluent to yield 271 mg (69%) of 14 as a creamy white solid. Mp 174–176 °C. 1H NMR (400 MHz, CDCl3) δ 3.74 (s, 3H), 3.78 (s, 3H), 6.63–6.68 (m, 4H), 6.91–7.15 (m, 11H), 8.33 (d, 2H, J = 6.1 Hz). 13C NMR (100 MHz, CDCl3) δ 56.1, 56.2, 114.1, 114.4, 115.8, 117.2, 127.1, 127.7, 129.1, 132.3, 133.6, 136.2, 136.4, 137.4, 143.9, 150.3, 153.4, 159.6, 159.9. HRMS (ESI): calcd for C27H24NO2 [M + H]+, 394.1807; found, 394.1810. X-Ray quality crystals of 14 were grown by slow evaporation of EtOAC/MeOH solution at rt.
1-Methyl-4-(2,2-bis(4-methoxyphenyl)-1-phenylvinyl)pyridinium tetrafluoroborate (15). Using the procedure for the preparation of 9 given above, 118 mg (0.30 mmol) 14 was converted to 15 (90.7 mg, 63%). Mp 169–171 °C. 1H NMR (400 MHz, acetone-d6) δ 3.72 (s, 3H), 3.78 (s, 3H), 4.43 (s, 3H), 6.70–7.23 (m, 13H), 7.52 (d, 2H, J = 7.0 Hz), 8.84 (d, 2H, J = 6.9 Hz). 13C NMR (100 MHz, acetone-d6) δ 48.6, 55.9, 56.1, 114.3, 115.2, 128.6, 129.6, 130.3, 132.6, 133.7, 134.2, 134.6, 135.1, 135.3, 142.9, 145.6, 149.8, 160.6, 161.1, 162.5. HRMS (ESI): calcd for C28H26NO2 [M]+, 408.1964; found, 408.1952.
4,4′-(2,2-Diphenylethene-1,1-diyl)dipyridine (18). Dibromoethene 16 (ref. 25) (338 mg, 1.00 mmol) was dissolved in 50 mL of dioxane
:
water (4
:
1). The flask was charged with Na2CO3 (690 mg, 5.00 mmol), Pd(OAc)2 (28 mg, 0.12 mmol), PPh3 (130 mg, 0.50 mmol) and 4-pyridine boronic acid 17a (615 mg, 5.00 mmol). The reaction was heated to reflux under argon overnight. After cooling, the reaction mixture was extracted with ethyl acetate (3 × 50 mL) and the combined organic fractions were dried over anhydrous Na2SO4, filtered, and concentrated under reduced pressure. The crude product was purified by flash column chromatography using ethyl acetate/hexane as eluent to yield 18 (183 mg, 55%) as a creamy white solid. Mp 162–165 °C. 1H NMR (300 MHz, CDCl3) δ 6.95 (d, 4H, J = 6.0 Hz), 7.06–7.09 (m, 4H), 7.19–7.26 (m, 6H), 8.43 (d, 4H, J = 6.0 Hz). 13C NMR (75 MHz, CDCl3) δ 124.1, 128.4, 130.5, 130.8, 133.5, 144.4, 148.4, 152.3, 152.8. HRMS (ESI): calcd for C24H19N2 [M + H]+, 335.1548; found, 335.1555. X-Ray quality crystals of 18 were grown by slow evaporation of EtOAc/MeOH solution at rt.
3,3′-(2,2-Diphenylethene-1,1-diyl)dipyridine (19). Using the procedure for the preparation of 18 given above and substitution of 3-pyridine boronic acid (17b) in place of 17a, DPDPyE 19 (207 mg, 62%) was obtained as a white solid. Mp 159–161 °C. 1H NMR (300 MHz, CDCl3) δ 7.06–7.20 (m, 5H), 7.47–7.75 (m, 9H), 8.31 (s, 2H), 8.39 (d, 2H, J = 4.9 Hz). 13C NMR (75 MHz, CDCl3) δ 125.5, 130.8, 131.1, 131.2, 133.8, 134.7, 134.8, 140.8, 144.8, 150.4, 154.5. HRMS (ESI): calcd for C24H19N2 [M + H]+, 335.1548; found, 335.1553.
4,4′-(2,2-Diphenylethene-1,1-diyl)bis(1-methylpyridinium) bis(tetrafluoroborate) (20). A mixture of trimethyloxonium tetrafluoroborate (219 mg, 1.50 mmol) and 18 (100 mg, 0.30 mmol) in 25 mL MeOH was heated to reflux for 5 d. The crude product was purified by flash column chromatography using methanol as eluent to yield 20 (109 mg, 72%) as an orange solid. Mp 139–141 °C. 1H NMR (400 MHz, DMSO-d6) δ 4.24 (s, 6H), 7.12–7.34 (m, 10H), 7.72 (d, 4H, J = 6.8 Hz), 8.82 (d, 4H, J = 6.5 Hz). 13C NMR (75 MHz, DMSO-d6) δ 50.7, 129.2, 131.8, 132.7, 134.1, 136.3, 142.8, 148.2, 156.1, 159.6. HRMS (ESI): calcd for C13H12N [M]2+/2, 182.0969; found, 182.0973.
3,3′-(2,2-Diphenylethene-1,1-diyl)bis(1-methylpyridinium) bis(tetrafluoroborate) (21). Using the procedure given for the preparation of 20, 100 mg 19 was converted to bis(pyridinium) salt 21 (88.3 mg, 58%). Mp 164–167 °C. 1H NMR spectrum: (300 MHz, acetone-d6) δ 4.38 (s, 6H), 7.27–7.39 (m, 10H), 8.07–8.19 (m, 2H), 8.68 (d, 2H, J = 6.9 Hz), 8.89 (s, 2H), 8.93 (d, 2H, J = 5.6 Hz). 13C NMR (100 MHz, acetone-d6) δ 49.2, 128.5, 129.7, 129.8, 132.1, 133.0, 133.1, 133.8, 141.2, 142.0, 144.9, 150.6. HRMS (ESI): calcd for C13H12N [M]2+/2, 182.0969; found, 182.0971.
1,1′-(Propane-1,3-diyl)bis(4-(1,2,2-triphenylvinyl)pyridinium) diiodide (24). Compound 6 (134 mg, 0.40 mmol), KI (66.4 mg, 0.40 mmol), and 1,3-dichloropropane (22.4 mg, 0.20 mmol) were combined in 50 mL CH3CN and heated to reflux for 6–7 d. After this time the solvent was evaporated and the residue purified by flash column chromatography using methanol as the eluent to afford 24 (121 mg, 63%) as a yellow-orange solid. Mp 135–138 °C. 1H NMR (300 MHz, methanol-d4) δ 2.07–2.13 (m, 2H), 4.73 (t, 4H, J = 7.2 Hz), 7.08–7.36 (m, 30H), 7.62 (d, 4H, J = 6.8 Hz), 8.81 (d, 4H, J = 6.9 Hz). 13C NMR (100 MHz, CDCl3) δ 30.7, 56.2, 129.1, 129.4, 129.6, 130.1, 130.2, 130.7, 130.8, 130.9, 132.1, 132.3, 132.5, 141.4, 141.6, 142.1, 144.4, 152.4, 163.3. HRMS (ESI): calcd for C53H44N2I [M]+, 835.2549; found, 835.2559.
1,1′-(Decane-1,10-diyl)bis(4-(1,2,2-triphenylvinyl)pyridinium) diiodide (25). Using the procedure given above and 1,10-dibromodecane (60 mg, 0.20 mmol) in place of dichloropropane, 25 (110 mg, 52%) was obtained as a yellow solid. Mp 186–189 °C. 1H NMR (300 MHz, acetone-d6) δ 1.22–1.41 (m, 16H), 4.86 (t, 4H, J = 7.3 Hz), 7.13–7.34 (m, 30H), 7.71 (d, 4H, J = 5.9 Hz), 9.27 (d, 4H, J = 6.6 Hz). 13C NMR (100 MHz, acetone-d6) δ 26.3, 29.4, 30.3, 32.1, 61.2, 128.8, 128.9, 129.4, 129.5, 129.6, 129.7, 130.5, 131.9, 132.3, 132.4, 137.3, 141.9, 142.4, 142.9, 145.5, 149.3, 161.8. HRMS (ESI): calcd for C30H29N [M]2+/2, 403.2300; found, 403.2297.
Hexafluorophosphate salts 26 and 27. The preparation of 26 is representative. Diiodide salt 24 (51 mg, 0.052 mmol) was dissolved in 5 mL CH2Cl2. A solution of saturated aq. NH4PF6 (25 mL) was added and the biphasic mixture was vigorously stirred at rt overnight. The layers were separated and the organic phase was dried over anhydrous Na2SO4, filtered, and concentrated to afford 26 (47 mg, 90%) as a yellow-orange solid. Mp 204–207 °C. Negative ion LRMS (ESI) confirmed the presence of PF6 (m/z = 145) and the absence of iodide. In similar fashion, 25 (62 mg, 0.058 mmol) was converted to 27 (55 mg, 87%). Mp 165–168 °C.
Acknowledgements
We thank the Department of Chemistry, University of Iowa and the UI Graduate College for a Radiochemistry Summer Fellowship (M.G.). We also thank Dale C. Swenson and Christopher J. Kassl for assistance with X-ray crystallography.
Notes and references
-
(a) S. W. Yun, N. Y. Kang, S. J. Park, H. H. Ha, Y. K. Kim, J. S. Lee and Y. T. Chang, Acc. Chem. Res., 2014, 47, 1277–1286 CrossRef CAS PubMed;
(b) J. Chan, S. C. Dodani and C. J. Chang, Nat. Chem., 2012, 4, 973–984 CrossRef CAS PubMed;
(c) H. Kobayashi, M. Ogawa, R. Alford, P. L. Choyke and Y. Urano, Chem. Rev., 2010, 110, 2620–2640 CrossRef CAS PubMed;
(d) L. D. Lavis and R. T. Raines, ACS Chem. Biol., 2008, 3, 142–155 CrossRef CAS PubMed.
-
(a) B. Rout, L. Motiei and D. Margulies, Synlett, 2014, 1050–1054 Search PubMed;
(b) K. P. Carter, A. M. Young and A. E. Palmer, Chem. Rev., 2014, 114, 4564–4601 CrossRef CAS PubMed;
(c) M. Zhu and C. Yang, Chem. Soc. Rev., 2013, 42, 4963–4976 RSC.
-
(a) Y. Hong, J. W. Y. Lam and B. Z. Tang, Chem. Commun., 2009, 4332–4353 RSC;
(b) J. Mei, Y. Hong, J. W. Y. Lam, A. Qin, Y. Tang and B. Z. Tang, Adv. Mater., 2014, 26, 5429–5479 CrossRef CAS PubMed.
-
(a) J. Liang, B. Z. Tang and B. Liu, Chem. Soc. Rev., 2015, 44, 2798–2811 RSC;
(b) D. Ding, K. Li, B. Liu and B. Z. Tang, Acc. Chem. Res., 2013, 46, 2441–2453 CrossRef CAS PubMed;
(c) Y. Hong, J. W. Y. Lam and B. Z. Tang, Chem. Soc. Rev., 2011, 40, 5361–5388 RSC;
(d) M. Wang, G. Zhang, D. Zhang, D. Zhu and B. Z. Tang, J. Mater. Chem., 2010, 20, 1858–1867 RSC.
- N. L. C. Leung, N. Xie, W. Yuan, Y. Liu, Q. Wu, Q. Peng, Q. Miao, J. W. Y. Lam and B. Z. Tang, Chem.–Eur. J., 2014, 20, 15349–15353 CrossRef CAS PubMed.
- F. Hu, Y. Huang, G. Zhang, R. Zhao and D. Zhang, Tetrahedron Lett., 2014, 55, 1471–1474 CrossRef CAS PubMed.
- F. Hu, G. Zhang, C. Zhan, W. Zhang, Y. Yan, Y. Zhao, H. Fu and D. Zhang, Small, 2015, 11, 1335–1344 CrossRef CAS PubMed.
- X. Chen, X. Y. Shen, E. Guan, Y. Liu, A. Qin, J. Z. Sun and B. Z. Tang, Chem. Commun., 2013, 49, 1503–1505 RSC.
- H. R. Xu, K. Li, S. Y. Jiao, S. L. Pan, J. R. Zeng and X. Q. Yu, Analyst, 2015, 140, 4182–4188 RSC.
-
(a) Y. Yuan, Y. Chen, B. Z. Tang and B. Liu, Chem. Commun., 2014, 50, 3868–3870 RSC;
(b) H. Shi, N. Zhao, D. Ding, J. Liang, B. Z. Tang and B. Liu, Org. Biomol. Chem., 2013, 11, 7289–7296 RSC.
-
(a) W. Zhang, R. T. K. Kwok, Y. Chen, S. Chen, E. Zhao, C. Y. Y. Yu, J. W. Y. Lam, Q. Zheng and B. Z. Tang, Chem.
Commun., 2015, 51, 9022–9025 CAS;
(b) N. Zhao, M. Li, Y. Yan, J. W. Y. Lam, Y. L. Zhang, Y. S. Zhao, K. S. Wong and B. Z. Tang, J. Mater. Chem. C, 2013, 1, 4640–4646 RSC.
- D. Y. Chen, J. B. Shi, Y. M. Yu, B. Tong, J. G. Zhi and Y. P. Dong, Sci. China: Chem., 2013, 56, 1239–1246 CrossRef CAS.
- T. Hu, B. Yao, X. Chen, W. Li, Z. Song, A. Qin, J. Z. Sun and B. Z. Tang, Chem. Commun., 2015, 51, 8849–8852 RSC.
-
(a) X. Yao, X. Ma and H. Tian, J. Mater. Chem. C, 2014, 2, 5155–5160 RSC;
(b) P. P. Kapadia, J. C. Widen, M. A. Magnus, D. C. Swenson and F. C. Pigge, Tetrahedron Lett., 2011, 52, 2519–2522 CrossRef CAS PubMed;
(c) P. P. Kapadia, M. A. Magnus, D. C. Swenson and F. C. Pigge, J. Mol. Struct., 2011, 1003, 82–86 CrossRef CAS PubMed.
- F. C. Pigge, J. Mol. Eng. Mater., 2013, 1, 1340009 CrossRef.
- G. Huang, G. Zhang and D. Zhang, Chem. Commun., 2012, 48, 7504–7506 RSC.
- F. C. Pigge, P. P. Kapadia and D. C. Swenson, CrystEngComm, 2013, 15, 4386–4391 RSC.
-
(a) X. Yan, T. R. Cook, P. Wang, F. Huang and P. J. Stang, Nat. Chem., 2015, 7, 342–348 CrossRef CAS PubMed;
(b) Q. Gong, Z. Hu, B. J. Deibert, T. J. Emge, S. J. Teat, D. Banerjee, B. Mussman, N. D. Rudd and J. Li, J. Am. Chem. Soc., 2014, 136, 16724–16727 CrossRef CAS PubMed;
(c) B. Icli, E. Solari, B. Kilbas, R. Scopelliti and K. Severin, Chem.–Eur. J., 2012, 18, 14867–14874 CrossRef CAS PubMed.
- B. Kilbas, S. Mirtschin, R. Scopelliti and K. Severin, Chem. Sci., 2012, 3, 701–704 RSC.
- A. Richardson Jr, J. B. Choudary and D. E. Holtkamp, J. Med. Chem., 1975, 18, 689–691 CrossRef CAS.
-
(a) K. Garg, E. Ganapathi, P. Rajakannu and M. Ravikanth, Phys. Chem. Chem. Phys., 2015, 17, 19465–19473 RSC;
(b) E. Zhao, H. Deng, S. Chen, Y. Hong, C. W. T. Leung, J. W. Y. Lam and B. Z. Tang, Chem. Commun., 2014, 50, 14451–14454 RSC;
(c) A. Bolzoni, L. Viglianti, A. Bossi, P. R. Mussini, S. Cauteruccio, C. Baldoli and E. Licandro, Eur. J. Org. Chem., 2013, 7489–7499 CrossRef CAS PubMed;
(d) D. Jana and B. K. Ghorai, Tetrahedron, 2012, 68, 7309–7316 CrossRef CAS PubMed;
(e) X. F. Duan, J. Zeng, J. W. Lü and Z. B. Zhang, Synthesis, 2007, 713–718 CrossRef CAS.
- N. S. Mills, C. Tirla, M. A. Benish, A. J. Rakowitz, L. M. Bebell, C. M. M. Hurd and A. L. M. Bria, J. Org. Chem., 2005, 70, 10709–10716 CrossRef CAS PubMed.
- M. Banerjee, S. J. Emond, S. V. Lindeman and R. Rathore, J. Org. Chem., 2007, 72, 8054–8061 CrossRef CAS PubMed.
-
(a) G. F. Zhang, Z. Q. Chen, M. P. Aldred, Z. Hu, T. Chen, Z. Huang, X. Meng and M. Q. Zhu, Chem. Commun., 2014, 50, 12058–12060 RSC;
(b) G. F. Zhang, H. Wang, M. P. Aldred, T. Chen, Z. Q. Chen, X. Meng and M. Q. Zhu, Chem. Mater., 2014, 26, 4433–4446 CrossRef CAS.
- J. C. Barnes, M. Juríček, N. L. Strutt, M. Frasconi, S. Sampath, M. A. Giesener, P. L. McGrier, C. J. Bruns, C. L. Stern, A. A. Sarjeant and J. F. Stoddart, J. Am. Chem. Soc., 2013, 135, 183–192 CrossRef CAS PubMed.
- ESI†.
- This solvent combination has been used to probe for AIE activity in other N-methylpyridinium TPE derivatives, see ref. 7.
- Z. Wang, J. H. Ye, J. Li, Y. Bai, W. Zhang and W. He, RSC Adv., 2015, 5, 8912–8917 RSC.
- G. A. Davis, J. Chem. Soc., Chem. Commun., 1973, 728–729 RSC.
- The effect of fhexane > 90% on the fluorescence of 20 and 21 was qualitatively examined, however no enhanced emission was observed at higher hexane content.
- The fluorescence quenching effect of iodide is well known and has been observed in other TPE derivatives, cf. ref. 7 and 9.
Footnote |
† Electronic supplementary information (ESI) available: Absorption and fluorescence spectra, NMR spectra, crystallographic data, cif files. CCDC 1421838 and 1421839. For ESI and crystallographic data in CIF or other electronic format see DOI: 10.1039/c5ra18724a |
|
This journal is © The Royal Society of Chemistry 2015 |