DOI:
10.1039/C5RA16250E
(Paper)
RSC Adv., 2015,
5, 83657-83667
Modified carbon paste and polymeric membrane electrodes for determination of hydroxychloroquine sulfate in pharmaceutical preparations and human urine
Received
12th August 2015
, Accepted 24th September 2015
First published on 24th September 2015
Abstract
This article describes characteristics, performance and application of hydroxychloroquine (HCQ) polymeric membrane (PMEs) and modified carbon paste (CPEs) electrodes which are based on sodium tetraphenylborate (NaTPB) or ammonium reineckate (Rt) as sensing materials. These electrodes showed Nernstian slopes of 29.1 ± 0.2, 28.5 ± 0.4, 28.0 ± 0.5 and 27.5 ± 0.5 mV decade−1 at 25 ± 0.1 °C, with low detection limits of 7.9 × 10−6, 8.2 × 10−6, 6.5 × 10−6 and 5.0 × 10−6 mol L−1 HCQ for PME1, PME2, CPE1 and CPE2 electrodes, respectively. The constructed electrodes were found to be precise and usable within the pH range of 2–8, 2–7, 2–8 and 2–7 for the electrodes, respectively. The electrodes manifest advantages of low resistance, very short response time ≤10 s, and most importantly good selectivity with respect to a number of common inorganic and organic species. Modified carbon paste electrodes showed lower detection limits reaching 5.0 × 10−6, higher thermal stability (0.0010 mV °C−1) and longer life time (54 days) than those of polymeric membrane electrodes. The electrodes were used for determination of hydroxychloroquine in its pure state, pharmaceutical preparation of hydroxychloroquine and biological fluids such as human urine samples.
1. Introduction
Hydroxychloroquine sulfate (HCQS) (Fig. 1), is an antimalarial drug used for treatment of acute attacks of malaria due to Plasmodium vivax, Plasmodium malariae, Plasmodium ovale, and susceptible strains of Plasmodium falciparum,1–3 where it is reported as being half as toxic as the closely related chloroquine (CQ) yet equally active against Plasmodium falciparum,4 and for treatment of a variety of chronic diseases such as rheumatoid arthritis (RA), systemic lupus erythematosus (SLE), discoid lupus (LD), sarcoidosis, Sjögren's syndrome (SS) and photosensitivity diseases.5–8
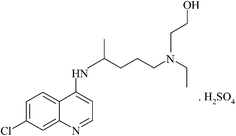 |
| Fig. 1 The chemical structure of hydroxychloroquine sulfate. | |
Owing to the wide use of this drug, various analytical techniques have been applied for determination of HCQS including extraction followed by nonaqueous titrimetric9 or spectrophotometric methods based on reactions with iodide,10 quinones11,12 and cobalt thiocyanate.13 Because of the nonspecificity of most of these reactions, prior extraction of HCQ is commonly involved in the assay methods. Besides, a number of different HPLC methods have been proposed for determination of HCQ14 in biological fluids15 using spectrophotometry,16 fluorescence,17–19 ultraviolet20 or tandem mass spectrometry21 detection. Few electrochemical methods have been used for determination of HCQ was found in the literature, such as, differential pulse voltammetry technique.22 However; these methods suffer from a variety of drawbacks, i.e. they are costly and in-appropriate for large-scale monitoring. Potentiometric sensors based on ion-selective electrodes (ISEs) can overcome these limitations.
Potentiometric sensors are an important class of electrochemical sensors, which detect the relationship between activity of analyte species and the observed potential response with the two-electrode system comprising an indicator electrode and a reference electrode. Ion-selective electrodes (ISEs) with polymeric membranes are the most commonly-used potentiometric sensors. They have been widely used for directly determining various inorganic and organic ions in medical, environmental and industrial analyses.23,24 Compared with other analytical techniques, ISEs have some unique characteristics, such as, small size, easiness of operation, portability and low cost. In the past few years, ISEs have made great progress in improving the lower limit of detection (LOD), exploiting new membrane materials, proposing new sensing concepts and developing deeper theoretical research about potentiometric responses.24
Carbon paste electrodes (CPEs) are considered an important type of ion-selective electrodes notable for their ubiquitous properties that entail advantages over membrane electrodes such as chemical inertness, robustness, renewability, stable response, low ohmic resistance, no need for internal solution and suitability for a variety of sensing and detection applications.25–27 Moreover, CPEs belong to nontoxic and environmentally friendly electrodes. In their case, problems with passivation are simply eliminated by a simple and quick renewal of their surface. The operational mechanism of the carbon paste electrodes depends on the properties of the modifier materials used to import selectivity towards the target species.28 Modified carbon electrodes have been widely used as sensitive and selective sensors in various electroanalytical methods.29
The present work describes construction and investigation of performance characteristics of new PME and CPE types for determination of HCQS in pure form, pharmaceutical preparation and human urine samples.
2. Experimental
2.1 Reagents and materials
All the chemicals used were of analytical grade. Bidistilled water was used throughout all experiments. Spectroscopic graphite powder (1–2 mm), dibutyl phthalate (DBP), dioctyl phthalate (DOP), dioctyl adipate (DOA), dioctyl sebacate (DOS) and tricresyl phosphate (TCP) were purchased from Merck. Sodium tetraphenylborate (NaTPB) and ammonium reineckate (NH4Rt) were obtained from Fluka. Polyvinyl chloride (PVC) of high molecular weight and tetrahydrofuran (THF) were purchased from Aldrich Chemical Company (USA). The metal salts were provided by BDH Company (UK) as nitrates or chlorides. Stock solutions of the metal salts were prepared in bidistilled water and standardized whenever necessary.
Pure-grade HCQS (Mwt = 433.95 g mol−1) was supplied by MINAPHARM Co., Cairo, Egypt. The pharmaceutical preparation (Hydroquine® 200 mg per tablet) was purchased from local drug stores. Standard solution of 1.0 × 10−2 mol L−1 HCQS was freshly prepared by dissolving the accurately weighed amount in bidistilled water. Working solutions of the drug (1.0 × 10−6 to 1.0 × 10−2 mol L−1) were prepared by suitable dilution from the standard solution with bidistilled water.
Stock solution of 1.0 × 10−2 mol L−1 NaTPB or NH4Rt was prepared by dissolving the accurately weighed amount of the pure solid in bidistilled water. Solutions of sodium hydroxide and hydrochloric acid of concentrations within the range (0.1–1.0) mol L−1 were used for adjusting the pH of the medium.
2.2 Apparatus
The electrochemical system of the PMEs and CPEs may be represented as follows:
Ag/AgCl/internal solution/membrane/test solution/Ag/AgCl double-junction reference electrode.
Modified carbon paste electrode/test solution/Ag/AgCl double-junction reference electrode.
An Ag/AgCl double-junction reference electrode (Metrohm 6.0222.100) was used as the external reference. Potentiometric and pH-measurements were carried out using 702 titroprocessor equipped with a 665 dosimat which made by Metrohm (Switzerland). A mLw W20 circulator thermostat was used to control temperature of the test solutions.
2.3 Preparation of the ion-pairs compounds
The ion-pairs compounds, HCQ–TPB and HCQ–Rt were prepared by slow addition of 50 mL of 1.0 × 10−2 mol L−1 sodium tetraphenylborate or ammonium reineckate solution to 100 mL of 1.0 × 10−2 mol L−1 HCQS under stirring for 15 min. The resulting precipitates were filtered off through a Whatman filter paper no. 42, washed with cold bidistilled water several times until they became sulfate free (tested using BaCl2 solution) was dried at room temperature and ground to fine powder. The composition of the ion-pairs were confirmed by elemental analysis to be 1
:
2 (HCQ
:
TPB) and 1
:
2 (HCQ
:
Rt).
2.4 Conductometric measurements
Conductometric titrations were followed with a Jenway conductivity meter. 50 mL of 1.0 × 10−3 mol L−1 HCQS solution was transferred to the 100 mL cell and the solution titrated against a 1.0 × 10−2 mol L−1 NaTPB or NH4Rt solution using a microburette. Conductance of each solution was measured after each addition of the titrant. Conductance values were corrected by multiplying by the dilution coefficient and plotted versus molar ratio. The titration plots showed a break which corresponds to the stoichiometry of each ion-pair.
2.5 Electrodes construction
Membranes of different compositions as indicated in Table 1 were prepared. The general procedure to prepare the polymeric membrane was as follows: different percentages of each ion-pair (cover the range of 2–9%), PVC and plasticizer with equal percentages were dissolved in minimum volume of tetrahydrofuran (THF), and the resulting mixture was transferred into a Petri dish of 6 cm diameter. The total weight of constituents in each batch was fixed at 0.35 g. The Petri dish was then covered with a filter paper and left to dry in air. To obtain a uniform membrane thickness, the amount of THF was kept constant, and its evaporation was fixed for 24 h. Thickness of the membrane is about 0.2 mm. A 12 mm diameter disk was cut out from the prepared membrane and glued to one end of a Pyrex glass tube using PVC-THF paste. Ratio of membrane ingredients, time of contact and concentration of conditioning solution were optimized; so that the potentials recorded were reproducible and stable within the standard deviation. Membrane to membrane reproducibility was assured by carefully following the optimum condition of fabrication. The membrane that gave reproducible results and showed best performance was selected for further studies. The optimized electrode body was filled with a solution of 1.0 × 10−1 mol L−1 NaCl and 1.0 × 10−2 mol L−1 HCQS. The electrode was preconditioned before use by soaking in a 1.0 × 10−3 mol L−1 HCQS solution for 30 minutes.
Table 1 Optimization of membrane compositions and their potentiometric response for PME electrodesa
Composition % (w/w) |
LOD (mol L−1) |
LR (mol L−1) |
Slope (mV decade−1) |
RSD (%) |
r2 |
HCQ–TPB |
HCQ–Rt |
PVC |
Plasticizer |
LR: linear range, LOD: limit of detection, RSD: relative standard deviation (three determinations), r2: correlation coefficient. |
PME1 |
3 |
— |
48.5 |
48.5 DBP |
1.6 × 10−5 |
1.0 × 10−4 to 1.0 × 10−2 |
26.2 |
0.60 |
0.9999 |
5 |
— |
47.5 |
47.5 DBP |
7.9 × 10−6 |
1.0 × 10−5 to 1.0 × 10−2 |
29.1 |
0.68 |
0.9999 |
7 |
— |
46.5 |
46.5 DBP |
1.7 × 10−5 |
1.0 × 10−4 to 1.0 × 10−2 |
28.6 |
0.74 |
0.9999 |
9 |
— |
45.5 |
45.5 DBP |
1.6 × 10−5 |
1.0 × 10−4 to 1.0 × 10−2 |
26.5 |
0.81 |
0.9999 |
5 |
— |
47.5 |
47.5 DOS |
2.5 × 10−5 |
1.0 × 10−4 to 1.0 × 10−2 |
24.9 |
0.64 |
0.9997 |
5 |
— |
47.5 |
47.5 DOP |
2.5 × 10−5 |
1.0 × 10−4 to 1.0 × 10−2 |
16.7 |
0.95 |
0.9996 |
5 |
— |
47.5 |
47.5 TCP |
1.6 × 10−5 |
1.0 × 10−4 to 1.0 × 10−2 |
20.6 |
0.77 |
0.9990 |
5 |
— |
47.5 |
47.5 DOA |
1.6 × 10−5 |
1.0 × 10−4 to 1.0 × 10−2 |
27.2 |
0.87 |
0.9999 |
![[thin space (1/6-em)]](https://www.rsc.org/images/entities/char_2009.gif) |
PME2 |
— |
3 |
49.0 |
49.0 DBP |
6.3 × 10−5 |
1.0 × 10−4 to 1.0 × 10−2 |
11.7 |
1.50 |
0.9998 |
— |
5 |
48.5 |
48.5 DBP |
8.2 × 10−6 |
1.0 × 10−5 to 1.0 × 10−2 |
28.5 |
0.70 |
0.9999 |
— |
7 |
47.5 |
47.5 DBP |
2.0 × 10−5 |
1.0 × 10−4 to 1.0 × 10−2 |
19.0 |
2.00 |
0.9990 |
— |
9 |
46.5 |
46.5 DBP |
2.5 × 10−5 |
1.0 × 10−4 to 1.0 × 10−2 |
14.0 |
0.98 |
0.9996 |
— |
5 |
48.5 |
48.5 DOS |
2.0 × 10−5 |
7.9 × 10−5 to 1.0 × 10−2 |
13.0 |
2.00 |
0.9980 |
— |
5 |
48.5 |
48.5 DOP |
2.0 × 10−5 |
1.0 × 10−4 to 1.0 × 10−2 |
10.5 |
0.50 |
0.9990 |
— |
5 |
48.5 |
48.5 TCP |
2.5 × 10−5 |
7.9 × 10−5 to 1.0 × 10−2 |
16.5 |
0.81 |
0.9999 |
— |
5 |
48.5 |
48.5 DOA |
1.6 × 10−5 |
1.0 × 10−4 to 1.0 × 10−2 |
10.0 |
0.98 |
0.9930 |
CPE electrodes were prepared by mixing the required amount of each ion-pair with graphite powder and pasting liquid in a mortar until it was uniformly wetted. The ready-prepared paste is then packed into the hole of the electrode body. A fresh surface was obtained by gently pushing the stainless steel screw forward and polishing the new carbon paste surface with filter paper to obtain a shiny new surface. The electrode was preconditioned before use by soaking in a 1.0 × 10−3 mol L−1 HCQS solution for 30 minutes, and when not in use, the electrode is stored in air.
2.6 Calibration of the electrodes
The conditioned electrodes were immersed in conjunction with An Ag/AgCl double-junction reference electrode in 25 mL aliquots of HCQS in the range of 1.0 × 10−2 to 1.0 × 10−6 mol L−1. They were allowed to equilibrate whilst stirring and recording e.m.f. readings within ±1 mV. The mV concentration profiles were plotted. The regression equations for the linear part of the curves were computed and used for subsequent determination of unknown concentrations of HCQS.
2.7 Selectivity
The potentiometric selectivity coefficients (KpotHCQ,j) of HCQ electrodes were evaluated at different concentrations of both HCQ and the interferents according to IUPAC recommendations using the separate solutions method (SSM) in case of inorganic cations (Li+, K+, Mg2+, Ca2+, Co2+, Mn2+, Fe2+, Cu2+, NH4+),30,31 and the matched potential method (MPM)32–34 in case of neutral species (amino acids, sugars, vitamins, and urea). In the separate solution method, the potential of a cell comprising a working electrode and a reference electrode is measured in two separate solutions, where, E1 is the potential measured in 1.0 × 10−3 mol L−1 HCQS, E2 is the potential measured in 1.0 × 10−3 mol L−1 of the interfering ion, z1 and z2 are the charges of hydroxychloroquine and interfering species, respectively, and S is slope of the electrode calibration plot. The selectivity coefficients were determined by the separate solution method using the rearranged Nikolsky equation:31
KpotHCQ,j = ((E1 − E2)/S) + (1 + (z1/z2))log a |
In 1995, IUPAC recommendation32 prescribes the MPM33,34 as the method of choice for ions of different charge. MPM is considered32 a purely operational method which is not relying on any theoretical or empirical model equation. The quantity used to express the extent of interference is the ratio of the primary ion concentration increment to the interfering ion concentration that gives the same potential change in a constant initial background of primary ion.
The selectivity coefficient was determined by measuring the change in potential upon increasing the primary ion activity from an initial value of aA to áA, and aB represents the activity of interfering ion added to the reference solution of primary ion of activity aA which also brings the same potential change. It is given by expression:
In the present study aA and áA were kept at 1.0 × 10−4 and 1.2 × 10−4 mol L−1 HCQS and aB was experimentally determined.
2.8 Potentiometric determination of hydroxychloroquine sulfate
The standard addition method was applied35 in which small increments of the standard solution 10−2 mol L−1 of HCQS were added to 50 mL aliquot samples of various concentrations from pure drug or pharmaceutical preparations. The change in millivolt reading was recorded for each increment and used to calculate the concentration of HCQS sample solution using the following equation:
where Cx and Vx are the concentration and the volume of the unknown, respectively, Cs and Vs are the concentration and the volume of the standard solution, respectively, S is the slope of the calibration graph and ΔE is the change in mV due to the addition of the standard solution.
2.9 Potentiometric titration of hydroxychloroquine sulfate
Aliquots of 1.0 × 10−2 mol L−1 drug solution (pure or tablet) were transferred into 50 mL volumetric flasks and made up to the mark with bidistilled water. Different concentrations of HCQS were prepared, then titrated potentiometrically with a standard solution of 1.0 × 10−2 mol L−1 NaTPB. The volume of the titrant at equivalence point was obtained using the conventional S-shaped curves. The differential graph of the titration curves have also been constructed to obtain well defined and sharp end points using the computer program “Origin Lab”.
2.10 Determination of hydroxychloroquine sulfate in pharmaceutical preparations
An accurate weight of Hydroquine® (200 mg per tablet) tablets ground and finely powdered in a small Petri dish and dissolved in the bidistilled water up to 30 mL by stirring for 1 h. The solution was filtered in a 50 mL measuring flask. The residue was washed three times with bidistilled water and the volume was completed to the mark using bidistilled water to form 1.0 × 10−2 mol L−1 HCQS solution. The resulting potentials of drug solution were directly measured using the constructed electrodes.
2.11 Determination of hydroxychloroquine sulfate in human urine
Different amounts of HCQS (0.43–4.34 mg) and 5 mL of urine of a healthy person were transferred to 50 mL measuring flask and completed to the mark using bidistilled water. Contents of the measuring flask were transferred to a 100 mL beaker and then subjected to standard addition method.
3. Results and discussion
3.1 Optimization the compositions of the electrodes
HCQ cation was found to form 1
:
2 water insoluble ion-pair with each of sodium tetraphenylborate and ammonium reineckate as indicated by elemental analysis data and ascertained using conductometric titration (Fig. 2). The prepared ion-pairs were identified and examined in PME and CPE electrodes responsive for HCQ cation (Fig. 3).
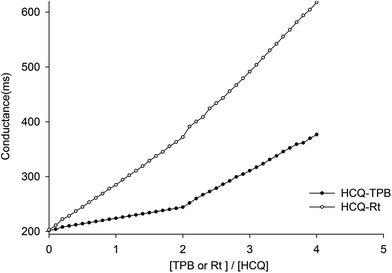 |
| Fig. 2 Conductometric titration curves of 1.0 × 10−3 mol L−1 HCQ against 1.0 × 10−2 mol L−1 NaTPB or Rt. | |
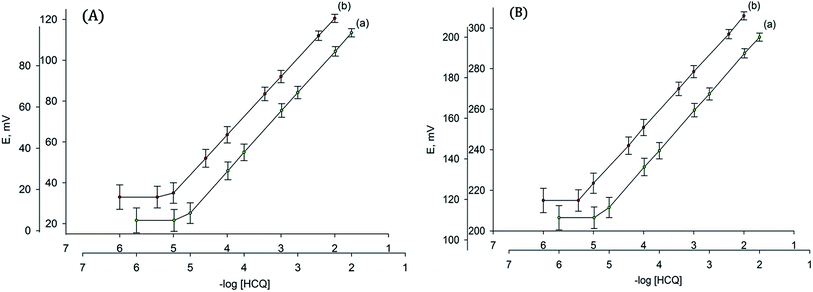 |
| Fig. 3 Calibration curves of HCQ electrodes: (A) (a) PME1, (b) PME2 and (B) (a) CPE1, (b) CPE2. | |
It is well known that the sensitivity, linear dynamic range and selectivity obtained for a given electrode depend significantly on the composition of the electrode. Effect of the composition on the potential response of the proposed electrodes was studied by varying the percentage of the ion-pairs (HCQ–TPB or HCQ–Rt) while keeping the percentage of the PVC or graphite powder and the plasticizer equal (1
:
1) (Tables 1 and 2).
Table 2 Optimization of CPE electrodes compositions and their potentiometric responsea
Composition % (w/w) |
LOD (mol L−1) |
LR (mol L−1) |
Slope (mV decade−1) |
RSD (%) |
r2 |
HCQ–TPB |
HCQ–Rt |
Graphite |
Plasticizer |
LR: linear range, LOD: limit of detection, RSD: relative standard deviation (three determinations), r2: correlation coefficient. |
CPE1 |
3 |
— |
48.5 |
48.5 DBP |
2.0 × 10−5 |
1.0 × 10−4 to 1.0 × 10−2 |
26.2 |
1.20 |
0.9998 |
5 |
— |
47.5 |
47.5 DBP |
6.5 × 10−6 |
1.0 × 10−5 to 1.0 × 10−2 |
28.0 |
0.44 |
0.9999 |
7 |
— |
46.5 |
46.5 DBP |
1.6 × 10−5 |
1.0 × 10−4 to 1.0 × 10−2 |
27.5 |
0.78 |
0.9990 |
9 |
— |
45.5 |
45.5 DBP |
1.6 × 10−5 |
1.0 × 10−4 to 1.0 × 10−2 |
25.5 |
0.62 |
0.9998 |
5 |
— |
47.5 |
47.5 DOS |
2.5 × 10−5 |
1.0 × 10−4 to 1.0 × 10−2 |
27.5 |
1.50 |
0.9990 |
5 |
— |
47.5 |
47.5 DOP |
2.5 × 10−5 |
1.0 × 10−4 to 1.0 × 10−2 |
27.0 |
0.80 |
0.9980 |
5 |
— |
47.5 |
47.5 TCP |
2.0 × 10−5 |
1.0 × 10−4 to 1.0 × 10−2 |
26.0 |
0.77 |
0.9990 |
5 |
— |
47.5 |
47.5 DOA |
2.5 × 10−5 |
1.0 × 10−4 to 1.0 × 10−2 |
37.5 |
0.55 |
0.9980 |
![[thin space (1/6-em)]](https://www.rsc.org/images/entities/char_2009.gif) |
CPE2 |
— |
2 |
49.0 |
49.0 DBP |
1.3 × 10−5 |
1.0 × 10−4 to 1.0 × 10−2 |
18.0 |
0.90 |
0.9960 |
— |
3 |
48.5 |
48.5 DBP |
5.0 × 10−6 |
5.0 × 10−6 to 1.0 × 10−2 |
27.5 |
1.30 |
0.9999 |
— |
5 |
47.5 |
47.5 DBP |
2.0 × 10−5 |
1.0 × 10−4 to 1.0 × 10−2 |
16.0 |
1.60 |
0.9999 |
— |
7 |
46.5 |
46.5 DBP |
6.3 × 10−5 |
1.0 × 10−4 to 1.0 × 10−2 |
17.5 |
1.40 |
0.9970 |
— |
3 |
48.5 |
48.5 DOS |
1.0 × 10−5 |
4.0 × 10−5 to 1.0 × 10−2 |
13.5 |
1.60 |
0.9995 |
— |
3 |
48.5 |
48.5 DOP |
2.0 × 10−5 |
7.9 × 10−4 to 1.0 × 10−2 |
23.0 |
1.60 |
0.9993 |
— |
3 |
48.5 |
48.5 TCP |
6.3 × 10−5 |
1.0 × 10−4 to 1.0 × 10−2 |
16.5 |
0.81 |
0.9970 |
— |
3 |
48.5 |
48.5 DOA |
1.6 × 10−5 |
1.0 × 10−4 to 1.0 × 10−2 |
35.0 |
0.61 |
0.9930 |
The results showed that the compositions of 5% (HCQ–TPB) and 47.5% of each of DBP and PVC for PME1, 5% of (HCQ–Rt) and 47.5% of each of DBP and PVC for PME2, 5% (HCQ–TPB) and 47.5% of each of DBP and graphite for CPE1 and 3% of (HCQ–Rt) and 48.5% of each of DBP and graphite for CPE2 gave good Nernstian slopes [29.1 ± 0.2, 28.5 ± 0.4, 28.0 ± 0.5 and 27.5 ± 0.5 mV decade−1 at 25 ± 0.1 °C, respectively] over a relatively wide concentration range of HCQS (1.0 × 10−5 to 1.0 × 10−2 mol L−1, 1.0 × 10−5 to 1.0 × 10−2, 1.0 × 10−5 to 1.0 × 10−2 and 5.0 × 10−6 to 1.0 × 10−2 mol L−1, with detection limits of 7.9 × 10−6, 8.2 × 10−6, 6.5 × 10−6 and 5.0 × 10−6 mol L−1 for PME1, PME2, CPE1 and CPE2 electrodes, respectively) (Fig. 3).
3.2 Effect of the plasticizers
Plasticizers can improve solubility of the sensing materials and lower the overall electrodes bulk resistance; due to their polarity characteristics. They influence detection limit, selectivity and sensitivity of the electrodes.36 In exploration for a suitable plasticizer for constructing these electrodes, we used five plasticizers with different polarities including DBP, DOP, DOS, DOA and TCP. The results (Tables 1 and 2) revealed that DBP was the best of the plasticizers tested. Poor sensitivities for the electrodes plasticized by DOP, DOS, DOA and TCP were due to low solubilities or low distributions of HCQ–TPB and HCQ–Rt ion-pairs in these solvents.37 The electrode using DBP as a plasticizer provided not only higher potentiometric response but also a wide response range, more stable potential reading and lower detection limit. Therefore, we used DBP as a suitable plasticizer for further studies.
3.3 Effect of the internal filling solution
The key to the improvements of detection limit has been the reduction of zero current ion flux effects that enhance the primary ion activities in the sensed sample layer near the membrane surface. This has been achieved by careful adjustment of the composition of the inner solution by reducing ion fluxes in the membrane and the thickness of the aqueous diffusion layer.38 Primary ions contaminating the sample in the sensed layer originated either from the membrane or from the internal solution.38 To investigate the effect of the internal filling solution composition on PME1 and PME2 electrodes response, the electrodes were filled with different inner filling solutions and calibration graphics were plotted for each case. The obtained results in Table 3 showed that the best results in terms of slope, limit of detection and working concentration range have been obtained with internal solutions of concentration 1.0 × 10−2 mol L−1 HCQ + 1.0 × 10−1 mol L−1 NaCl and 1.0 × 10−4 mol L−1 HCQ + 1.0 × 10−1 mol L−1 NaCl for PME1 and PME2 electrodes, respectively.
Table 3 Effect of internal filling solution on the potential response of PME electrodesa
Internal filling solution |
LR, mol L−1 |
LOD, mol L−1 |
Slope, mV decade−1 |
LR: linear range, LOD: limit of detection. |
PME1 |
10−2 mol L−1 HCQS |
6.30 × 10−6 to 3.50 × 10−4 |
5.0 × 10−6 |
42.8 ± 0.4 |
10−3 mol L−1 HCQS |
1.00 × 10−5 to 5.00 × 10−4 |
8.7 × 10−6 |
38.8 ± 0.4 |
10−4 mol L−1 HCQS |
1.00 × 10−4 to 1.00 × 10−2 |
6.3 × 10−6 |
35.0 ± 0.5 |
10−2 mol L−1 HCQS + 10−1 mol L−1 NaCl |
1.00 × 10−5 to 1.00 × 10−2 |
7.9 × 10−6 |
29.1 ± 0.2 |
10−3 mol L−1 HCQS + 10−1 mol L−1 NaCl |
2.00 × 10−5 to 5.00 × 10−4 |
8.7 × 10−6 |
30.1 ± 0.1 |
10−4 mol L−1 HCQS + 10−1 mol L−1 NaCl |
5.00 × 10−5 to 1.00 × 10−2 |
1.1 × 10−5 |
30.9 ± 0.9 |
![[thin space (1/6-em)]](https://www.rsc.org/images/entities/char_2009.gif) |
PME2 |
10−2 mol L−1 HCQS |
1.00 × 10−5 to 1.00 × 10−3 |
1.0 × 10−5 |
22.5 ± 0.5 |
10−3 mol L−1 HCQS |
5.01 × 10−4 to 1.00 × 10−2 |
7.6 × 10−5 |
24.9 ± 0.5 |
10−4 mol L−1 HCQS |
5.01 × 10−4 to 1.00 × 10−2 |
5.9 × 10−5 |
30.3 ± 0.3 |
10−2 mol L−1 HCQS + 10−1 mol L−1 NaCl |
5.01 × 10−4 to 1.00 × 10−2 |
1.3 × 10−4 |
26.4 ± 0.4 |
10−3 mol L−1 HCQS + 10−1 mol L−1 NaCl |
1.00 × 10−4 to 1.00 × 10−2 |
3.4 × 10−5 |
24.0 ± 0.1 |
10−4 mol L−1 HCQS + 10−1 mol L−1 NaCl |
1.00 × 10−5 to 1.00 × 10−2 |
8.2 × 10−5 |
28.5 ± 0.4 |
3.4 Life time and regeneration of the electrodes
The Life times of the constructed electrodes were determined for interval range till the electrodes lost Nernstian behaviour. This behaviour was attributed to the leaching of the active ingredients (ion-pair and plasticizer) to the bathing solution. Response of the electrodes has been measured by recording the calibration graph at 25 °C at different intervals. Life times of the electrodes were found to be 35, 7, 54 and 42 days for PME1, PME2, CPE1 and CPE2 electrodes, respectively during which the electrodes showed a slight gradual decrease in the slope and an increase in the detection limit. Carbon paste electrodes showed a relatively long-term stability than the polymeric membrane electrodes which may be attributed to the diminishing of the ion-pair leaching from the electrode matrix; due to absence of the internal filling solution.
The regeneration of the HCQ electrodes was successfully achieved by soaking the expired electrodes for 24 h in a solution that was 1.0 × 10−2 mol L−1 sodium tetraphenylborate or ammonium reineckate, followed by soaking for 3 h in 1.0 × 10−2 mol L−1 HCQS solution.39 The calibration graphs for exhausted electrodes showed (slopes 21.8 ± 0.4, 22.4 ± 0.1, 19.0 ± 0.3 and 23.0 ± 0.5 mV decade−1) and after regeneration (slopes 28.0 ± 0.2, 28.5 ± 0.5, 28 ± 0.4 and 27.2 ± 0.2 mV decade−1) for PME1, PME2, CPE1 and CPE2 electrodes, respectively (Fig. 4). It was found that the life span of the regenerated electrodes is limited to 8 h; due to the ease of leaching of the lipophilic salts from the gel layer at the surface of each electrode compared with those that are attached homogeneously through the solvent mediator.
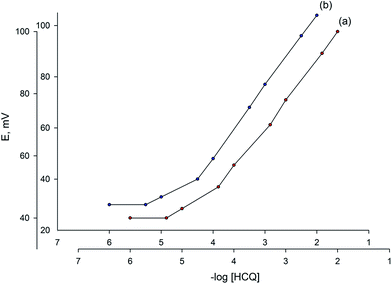 |
| Fig. 4 Calibration curves of (a) expired and (b) regenerated PME1 electrode. | |
3.5 Dynamic response time and repeatability of the electrodes
The dynamic response time40 is defined as the time which elapses between the instant at which an ion-selective electrode and a reference electrode (ISE cell) are brought into contact with a sample solution. The dynamic response time of each electrode was tested by measuring the time required to achieve a steady-state potential (within ±1 mV) after successive immersions of the electrode in a series of drug solutions, each having a 10-fold increase in concentration from 1.0 × 10−5 to 1.0 × 10−2 mol L−1. The electrodes yielded steady potentials within 10 s as shown in Fig. 5.
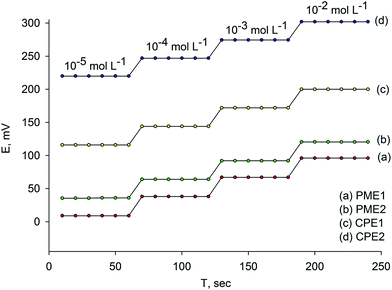 |
| Fig. 5 Dynamic response time of HCQ electrodes for step change in concentrations of HCQS from low to high. | |
Repeatability of the potential readings for each electrode was examined by subsequent measurement in 1.0 × 10−3 mol L−1 HCQS solution immediately after measuring in 1.0 × 10−2 mol L−1 HCQS solution (Fig. 6). The potentiometric response of the electrodes showed no memory effect.
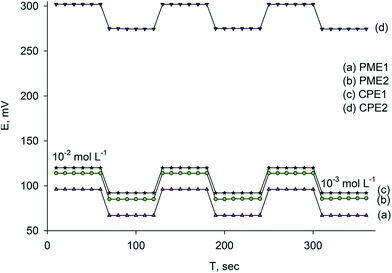 |
| Fig. 6 Dynamic response of HCQ electrodes for several high-to-low sample cycles. | |
3.6 Effect of the pH
The most important factor in functioning of most ion selective electrodes is the medium acidity expressed as pH value. The effect of the pH of the solution on the response of the proposed electrodes was studied for two concentrations of HCQS (1.0 × 10−3 and 1.0 × 1.0−4 mol L−1) in the pH range of 1.5–11.0. The pH was adjusted with (0.1–1.0 mol L−1) solutions of hydrochloric acid or sodium hydroxide. The results showed that the potential response remained almost constant over the pH ranges 2–8, 2–7, 2–8 and 2–7 for PME1, PME2, CPE1 and CPE2 electrodes, respectively which can be taken as the working pH ranges of the electrodes (Fig. 7). At higher pH values, the potential showed a sharp decrease; due to the formation of the nonprotonated [HCQ], leading to a decrease in concentration of [HCQ+]. However, at lower pH values, the decrease in the potential may have been due to interference of hydronium ion.
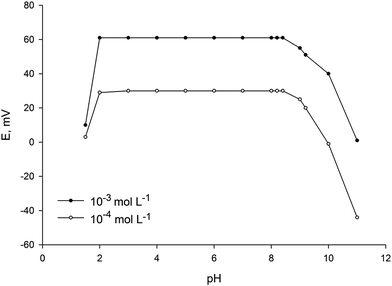 |
| Fig. 7 Effect of pH of the test solutions on the potential response of PME1 electrode. | |
PME1, PME2, CPE1 and CPE2 electrodes response were checked with bidistilled water, 0.1 mol L−1 acetate buffer pH 5.0 or 0.1 mol L−1 phthalate buffer pH 5.0. The best results were achieved in bidistilled water in the case of these electrodes; because it provided not only a higher Nernstian slope but also a stable potential reading. Therefore, bidistilled water was used for all the constructed electrodes.
3.7 Selectivity of the electrodes
The potentiometric selectivity coefficient of an electrode, as one of the most important characteristics, is defined by its relative response for the primary ion over the other ions present in the solution.41 The selectivity coefficients values of the polymeric membrane and carbon paste electrodes reflect a very high selectivity of the investigated electrodes for the HCQ cation. The inorganic cations do not interfere; owing to the differences in ionic size and consequently their mobilities and permeabilities as compared with [HCQ+]. The selectivity sequence significantly differs from the so called Hofmeister selectivity sequence42 (i.e. selectivity is solely based on lipophilicity of cation). In case of non-ionic species, the high selectivity is mainly attributed to the difference in polarity and to the lipophilic nature of their molecules relative to HCQ cation. Mechanism of selectivity is mainly based on the stereospecificity and electrostatic environment, and is dependent on how much fitting is present between locations of the lipophilicity sites in two competing species in the bathing solution side and those present in the receptor of the ion-exchanger43 (Table 4).
Table 4 Selectivity coefficient values of HCQ electrodes
Interferent |
KpotHCQ,j |
PME1 |
PME1 |
CPE1 |
CPE2 |
SSM |
MPM |
SSM |
MPM |
SSM |
MPM |
SSM |
MPM |
K+ |
1.8 |
— |
1.5 |
— |
2.7 |
— |
4.3 |
— |
NH4+ |
2.1 |
— |
1.8 |
— |
1.9 |
— |
2.7 |
— |
Li+ |
2.7 |
— |
2.7 |
— |
2.0 |
— |
3.3 |
— |
Fe2+ |
3.1 |
— |
3.2 |
— |
1.5 |
— |
1.5 |
— |
Ca2+ |
3.6 |
— |
2.9 |
— |
4.1 |
— |
4.1 |
— |
Mg2+ |
3.3 |
— |
2.0 |
— |
4.3 |
— |
4.1 |
— |
Mn2+ |
3.2 |
— |
2.2 |
— |
1.6 |
— |
1.9 |
— |
Cu2+ |
3.5 |
— |
1.5 |
— |
4.1 |
— |
2.4 |
— |
Co2+ |
3.5 |
— |
1.4 |
— |
1.8 |
— |
2.2 |
— |
Vitamine C |
— |
1.5 |
— |
1.8 |
— |
2.4 |
— |
2.3 |
Vitamine B6 |
— |
1.4 |
— |
1.7 |
— |
2.4 |
— |
2.4 |
Glucose |
— |
2.0 |
— |
1.4 |
— |
1.8 |
— |
2.5 |
Fructose |
— |
2.0 |
— |
1.4 |
— |
1.8 |
— |
2.0 |
Lactose |
— |
2.1 |
— |
1.5 |
— |
1.6 |
— |
2.4 |
Maltose |
— |
2.0 |
— |
1.6 |
— |
1.9 |
— |
2.0 |
Urea |
— |
1.9 |
— |
1.7 |
— |
1.7 |
— |
1.9 |
Glycine |
— |
2.1 |
— |
1.7 |
— |
1.8 |
— |
1.8 |
β-Alanine |
— |
2.0 |
— |
2.1 |
— |
1.8 |
— |
1.9 |
Hestidine |
— |
2.1 |
— |
1.8 |
— |
1.9 |
— |
1.6 |
CPEs are indeed low-resistant. This advantage is achieved; due to the high impact of the electronically conducting material – carbon to the overall conductivity of the electrode phase. In turn, this causes the sensitivity of CPE1 and CPE2 potential to RedOx agents in samples, such as Co2+, Mn2+, and Fe2+. The values of selectivity coefficient for these alkaline metal ions are found somewhat high compared with those for other inorganic cations. This behavior is mainly attributed to the impact of RedOx interaction in case of CPEs (Table 4).
3.8 Effect of temperature
Calibration plots were constructed in the test solution temperatures (15, 20, 25, 30, 40, and 50 °C) for polymeric membrane and carbon paste electrodes. For determination of the thermal coefficient (dE°/dt) of the electrodes, the standard electrode potentials (E°) at different temperatures were plotted vs. t-25, where t is the temperature of the test solution. A straight line is obtained according to the Antropov's equation44
E° = E°25 + (dE° /dt)(t-25) |
Slopes of the straight lines obtained represent the thermal coefficients of the electrodes, amounting to 0.0013, 0.0017, 0.0010 and 0.0016 mV °C−1 for PME1, PME2, CPE1 and CPE2 electrodes, respectively. These low values of thermal temperature coefficients reveal that the electrodes have thermal stability within the studied temperature range (15–50 °C).
3.9 Analytical applications
The investigated electrodes were proved to be useful in the potentiometric determination of HCQ in pure solutions and in its pharmaceutical preparations by applying the potentiometric titration (Fig. 8) and standard additions methods. Collective results are given in Tables 5 and 6. From the results, it is evident that the present electrodes are very useful as potentiometric sensors for the micro-determination of HCQ in pure solutions and its pharmaceutical preparations. The results in Table 7 show that the calculated F- and t-values did not exceed the theoretical values, reflecting the accuracy and precision of the applied method.
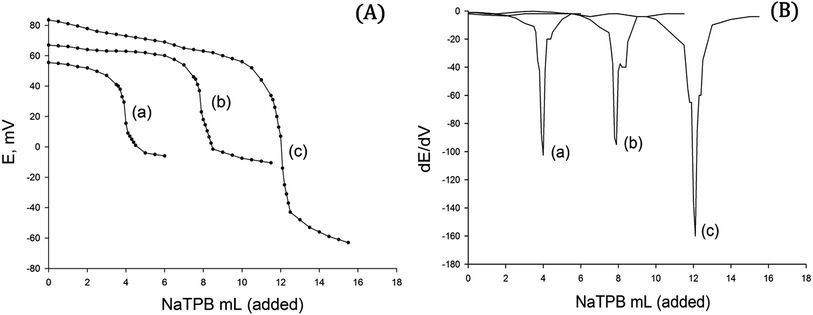 |
| Fig. 8 (A) Potentiometric titration curves of (a) 2 (b) 4 and (c) 6 mL of 10−2 mol L−1 HCQS using PME1 electrode and 10−2 mol L−1 NaTPB as titrant and (B) its first order derivative. | |
Table 5 Determination of HCQ applying standard addition method
Sample |
Taken (mg) |
PME1 |
PME2 |
CPE1 |
CPE2 |
Recovery (%) |
RSD (%) |
Recovery (%) |
RSD (%) |
Recovery (%) |
RSD (%) |
Recovery (%) |
RSD (%) |
Pure sample |
0.43 |
98.4 |
0.65 |
101.0 |
2.30 |
97.0 |
2.50 |
99.4 |
1.03 |
1.08 |
98.9 |
1.76 |
99.5 |
2.50 |
97.5 |
0.53 |
99.7 |
1.72 |
2.17 |
98.6 |
1.53 |
100.0 |
2.20 |
99.2 |
2.46 |
100.4 |
0.55 |
4.34 |
99.5 |
1.30 |
99.8 |
1.80 |
100.0 |
1.60 |
100.0 |
0.90 |
Hydroquine® (200 mg per tablet) sample |
0.43 |
99.5 |
1.88 |
100.7 |
0.72 |
100.3 |
1.55 |
99.6 |
1.36 |
1.08 |
99.4 |
0.36 |
97.2 |
1.30 |
99.2 |
2.30 |
97.2 |
1.44 |
2.17 |
99.0 |
1.31 |
99.3 |
0.67 |
99.6 |
0.82 |
97.4 |
1.96 |
4.34 |
100.1 |
1.60 |
100.2 |
0.44 |
100.1 |
1.50 |
99.8 |
1.00 |
Urine sample |
0.43 |
101.0 |
1.10 |
99.0 |
1.40 |
99.6 |
1.76 |
100.6 |
0.55 |
1.08 |
100.5 |
1.15 |
100.4 |
1.50 |
99.9 |
1.32 |
100.7 |
0.42 |
2.17 |
100.2 |
0.50 |
98.4 |
1.10 |
99.6 |
1.18 |
100.5 |
1.05 |
4.34 |
99.8 |
0.53 |
99.9 |
1.00 |
99.6 |
1.50 |
100.2 |
0.64 |
Table 6 Determination of HCQ applying potentiometric titration method
Sample |
Taken (mg) |
PME1 |
PME2 |
CPE1 |
CPE2 |
Recovery (%) |
RSD (%) |
Recovery (%) |
RSD (%) |
Recovery (%) |
RSD (%) |
Recovery (%) |
RSD (%) |
Pure sample |
8.70 |
100.0 |
1.00 |
102.2 |
1.60 |
100.0 |
0.40 |
102.5 |
0.77 |
17.40 |
98.8 |
0.40 |
101.3 |
1.30 |
98.8 |
0.86 |
100.0 |
0.89 |
26.04 |
100.8 |
0.70 |
100.0 |
0.86 |
100.0 |
0.90 |
100.8 |
0.36 |
Hydroquine® (200 mg per tablet) sample |
8.70 |
100.0 |
0.77 |
102.5 |
1.80 |
102.5 |
1.30 |
100.0 |
1.10 |
17.40 |
100.0 |
0.60 |
100.0 |
1.40 |
101.3 |
0.86 |
97.5 |
1.30 |
26.04 |
99.2 |
0.30 |
99.2 |
0.73 |
100.0 |
0.61 |
100.0 |
0.50 |
Table 7 Statistical comparison between the results of an analysis of a pharmaceutical preparation Hydroquine® tablets applying standard addition and potentiometric titration methods
Parameters |
Standard addition method |
Potentiometric titration method |
Average of four determinations. Average of three determinations, SD: standard deviation, RSD: relative standard deviation. Tabulated F-value at 95% confidence level. Tabulated t-value at 95% confidence level and five degrees of freedom. |
PME1 |
Mean recovery (%) |
99.7a |
99.50b |
SD |
0.46 |
0.46 |
RSD (%) |
0.46 |
0.47 |
F-ratio |
3.60 (9.55)c |
|
t-test |
0.57 (2.57)d |
|
![[thin space (1/6-em)]](https://www.rsc.org/images/entities/char_2009.gif) |
PME2 |
Mean recovery (%) |
100.1a |
97.9b |
SD |
1.60 |
1.72 |
RSD (%) |
1.61 |
1.71 |
F-ratio |
1.24 (9.55)c |
|
t-test |
0.97 (2.57)d |
|
![[thin space (1/6-em)]](https://www.rsc.org/images/entities/char_2009.gif) |
CPE1 |
Mean recovery (%) |
99.3a |
98.8b |
SD |
0.50 |
1.25 |
RSD (%) |
0.50 |
1.23 |
F-ratio |
6.26 (9.55)c |
|
t-test |
2.23 (2.57)d |
|
![[thin space (1/6-em)]](https://www.rsc.org/images/entities/char_2009.gif) |
CPE2 |
Mean recovery (%) |
98.5a |
97.9b |
SD |
1.40 |
1.44 |
RSD (%) |
1.42 |
1.50 |
F-ratio |
1.08 (9.55)c |
|
t-test |
0.46 (2.57)d |
|
Determination of HCQ was carried using the standard addition method in pure drug, in pharmaceutical preparations and also in spiked human urine samples without fear of interference caused by the excipients expected to be present in tablets. The mean recoveries obtained for determination of HCQ in spiked urine samples were in range of 99.8–101.0, 98.4–100.4, 99.6–99.9 and 100.2–100.7% for PME1, PME2, CPE1 and CPE2 electrodes, respectively (Table 5). The results obtained from the standard addition method of the drug were compared with those obtained from the potentiometric titration method by applying F- and t-tests.45
For ruggedness of the method, a comparison was performed between the intra- and inter-day assay results for HCQ obtained by two M. Sc. students. The RSD values for the intra- and inter-day assays of HCQ in the cited formulations performed in the same laboratory by the two analysts did not exceed 2.50%. On the other hand, the robustness was examined while the parameter values (pH of the eluent and the laboratory temperature) were being deliberately slightly changed. HCQ recovery percentages were good under most conditions and they didn't show any significant change when the critical parameters were modified.
3.10 Comparison with reported methods
Performance characteristics of the proposed electrode and those of the reported methods are compiled in Table 8 for comparison. It is clear that the detection limits of the constructed electrodes are lower than that of pulse differential voltammetry method.22 In addition, range of concentration of the constructed electrodes are wider than that of both pulse differential voltammetry and spectrophotometry methods,22 which indicates the ability of the constructed electrodes to face such automated methods.
Table 8 Comparison of the proposed HCQ ion-selective electrode method with published methodsa
Method |
LR mol L−1 |
LOD mol L−1 |
r2 |
RSD % |
Ref. |
P.W: present Work. |
Spectrophotometry |
4.0 × 10−6 to 2.0 × 10−5 |
2.30 × 10−8 |
0.9999 |
0.36 |
22 |
Pulse differential voltammetry |
2.0 × 10−5 to 5.0 × 10−4 |
2.60 × 10−5 |
0.9999 |
0.46 |
22 |
![[thin space (1/6-em)]](https://www.rsc.org/images/entities/char_2009.gif) |
Ion selective electrode |
PME1 |
1.0 × 10−5 to 1.0 × 10−2 |
7.9 × 10−6 |
0.9999 |
0.68 |
[P.W] |
PME2 |
1.0 × 10−5 to 1.0 × 10−2 |
8.2 × 10−6 |
0.9999 |
0.70 |
[P.W] |
CPE1 |
1.0 × 10−5 to 1.0 × 10−2 |
6.5 × 10−6 |
0.9999 |
0.44 |
[P.W] |
CPE2 |
5.0 × 10−5 to 1.0 × 10−2 |
5.0 × 10−6 |
0.9999 |
1.30 |
[P.W] |
4. Conclusion
The present work describes the fabrication of HCQ polymeric membrane and modified carbon paste electrodes are based on HCQ–TPB and HCQ–Rt as electroactive materials. The proposed electrodes showed Nernstian slopes with low detection limits, fast response time (10 s) and long operational life time in the concentration range 5.0 × 10−6 to 1.0 × 10−2 mol L−1. Characterization of the constructed electrodes showed that modified carbon paste electrodes can detect concentrations to 5.0 × 10−6 mol L−1 HCQ with high thermal stability about (0.0010 mV °C−1) for long life time (54 days). This, in turn, indicates superiority of the modified carbon paste electrodes over polymeric membrane electrodes. The fabricated electrodes were successfully applied to the potentiometric determination of HCQ in pure solutions, pharmaceutical preparations and spiked urine samples. The constructed electrodes showed high sensitivity, reasonable selectivity, fast static response, long-term stability, applicability over a wide pH range with minimal pretreatment for the sample, in addition to other advantages over the previously reported methods.
References
- M. T. Hoekenga, Am. J. Trop. Med. Hyg., 1955, 4, 221 CAS.
- A. Korolkovas and J. H. Burkhalter, Química Farmacêutica, ed. R. de Janeiro, Guanabara-Koogan, 1988 Search PubMed.
- H. Ginsburg and T. G. Geary, Biochem. Pharmacol., 1987, 36, 1567 CrossRef CAS.
- Aminoquinolines, in Handbook of Experimental Pharmacology, ed. McChesney and E. W., Fitch, W. Peters and W. H. G. Richards, Springer: Verlag, Berlin, 1984, vol. 2, pp. 3–60, C. D. 68 Search PubMed.
- N. J. Olsen, M. A. Schleich and D. R. Karp, Semin. Arthritis Rheum., 2013, 43, 264 CrossRef CAS PubMed.
- S. E. Tett, D. J. Cutler, C. Beck and R. O. Day, J. Rheumatol., 2000, 27, 1656 CAS.
- A. J. McLachlan, S. E. Tett, D. J. Cutler and R. O. Day, Br. J. Clin. Pharmacol., 1993, 36, 78 CrossRef CAS PubMed.
- T. A. Shapiro and D. D. Goldberg, L. S. Goodman and A. Gilman, As Bases Farmacológicas da Terapêutica, McGraw-Hill, Rio de Janeiro, 2006, p. 927 Search PubMed.
- British Pharmacopoeia, edn V, VI, 1st, London, 1993, p. 339 Search PubMed.
- A. M. Salam, M. Issa and H. J. Lymona, J. Pharm. Belg., 1986, 41, 314 Search PubMed.
- B. S. Sastry, E. V. Rao, M. V. Suryanarayana and C. S. P. Sastry, Pharmazie, 1986, 41, 739 CAS.
- P. J. Volin, J. Chromatogr., Biomed. Appl., 1995, 666, 347 CrossRef CAS.
- Y. M. Wei, G. A. Nygard and S. K. W. Khalil, J. Liq. Chromatogr., 1994, 17, 3479 CrossRef CAS PubMed.
- S. E. Tett, D. J. Cutler and K. F. Brown, J. Chromatogr., Biomed. Appl., 1986, 383, 236 CrossRef CAS.
- K. Croes, P. T. McCarthy and R. J. Flanagan, J. Anal. Toxicol., 1994, 18, 255 CrossRef CAS PubMed.
- D. R. Brocks, F. M. Pasutto and F. J. Jamali, J. Chromatogr., Biomed. Appl., 1992, 581, 83 CrossRef CAS.
- J. F. Chaulet, Y. Robet, J. M. Prevosto, O. Soares and J. L. J. Brazier, Chromatographia, 1993, 613, 303 CAS.
- J. F. Chaulet, C. Mounier, O. Soares and J. L. Brazier, Anal. Lett., 1991, 24, 665 CrossRef CAS PubMed.
- S. B. Williams, L. C. Patchen and F. C. Churchill, J. Chromatogr., Biomed. Appl., 1988, 433, 197 CrossRef CAS.
- G. Morris Raymond, J. Chromatogr., Biomed. Appl., 1986, 338, 422 CrossRef.
- L. Z. Wang, R. Y. Ong, T. M. Chin, W. L. Thuya, S. C. Wan, A. L. Wong, S. Y. Chan, P. C. Ho and B. C. Goh, J. Pharm. Biomed. Anal., 2012, 61, 86 CrossRef CAS PubMed.
- P. M. Maria Lara Arguelho, J. F. Andrade and N. R. Stradiotto, J. Pharm. Biomed. Anal., 2003, 32, 269 CrossRef.
- The Principles of Ion-Selective Electrodes and of MembraneTransport, ed. Morf and W. E., Elsevier, New York, 1981 Search PubMed.
- Glass Electrodes for Hydrogen and Other Cations, ed. Eisenman and Dekker, New York, 1967 Search PubMed.
- A. Solymanpour and M. Ghasemian, Measurement, 2015, 59, 14 CrossRef PubMed.
- H. M. Abu Shawish, A. A. Dalou, N. Ab Ghalwa, G. I. Kharnish, J. Hammad and A. H. Basheer, Drug Test. Anal., 2013, 5, 213 CrossRef CAS PubMed.
- H. M. Abu Shawish, N. Ab Ghalwa, S. M. saadeh and H. El Harazeem, Food Chem., 2013, 138, 126 CrossRef CAS PubMed.
- K. Kalcher, J. M. Kauffmann, J. Wang, I. Svancara, K. Vytras, C. Neuhold and Z. Yang, Electroanalysis, 1995, 7, 5 CrossRef CAS PubMed.
- R. F. Aglan, G. G. Mohamed and H. A. Mohamed, AJAC, 2012, 3, 576 CrossRef.
- E. Baumann, Anal. Chim. Acta, 1968, 42, 127 CrossRef CAS.
- G. G. Guilbault, R. A. Durst, M. S. Frant, H. Freiser, E. H. Hansen, T. S. Light, E. Pungor, G. A. Rechnitz, N. M. Rice, T. J. Rohm, W. Simon and J. D. R. Thomas, Pure Appl. Chem., 1976, 48, 127 Search PubMed.
- Y. Umezawa, P. Buhlmann, K. Umezawa, K. Tohda and S. Amemiya, IUPAC, Pure Appl. Chem., 2000, 72, 1851 CrossRef CAS.
- Y. Umezawa, P. Buhlmann, K. Umezawa and H. Sato, Pure Appl. Chem., 1995, 67, 507 CrossRef.
- V. P. Y. Gadzekpo and G. D. Christian, Anal. Chim. Acta, 1984, 164, 279 CrossRef CAS.
- R. Y. Xie, V. P. Y. Gadzekpo, A. M. Kadry, Y. A. tbrahim, J. Ruzicka and G. D. Christian, Anal. Chim. Acta, 1986, 184, 259 CrossRef CAS.
- A. R. Fakhari, T. A. Raji and H. Naeimi, Sens. Actuators, B, 2005, 104, 317 CrossRef CAS PubMed.
- R. P. Armstrong and G. Horvoi, Electrochim. Acta, 1990, 35, 1 CrossRef CAS.
- J. Sutter, A. Radu, S. Peper, E. Bakker and E. Pretsch, Anal. Chim. Acta, 2004, 523, 53 CrossRef CAS PubMed.
- Dynamic characteristic of ion-selective electrodes, E. Lindner, K. Toth and E. Pungor, CRC Press, BocaRaton, USA, 1988 Search PubMed.
- R. P. Buck and E. Lindner, Pure Appl. Chem., 1994, 66, 2527 CrossRef CAS.
- K. A. Singh and S. Mehtab, Sens. Actuators, B, 2007, 123, 429 CrossRef PubMed.
- F. Hofmeister, Arch. Exp. Pathol. Pharmakol., 1888, 24, 247 Search PubMed.
- N. T. Abdel-Ghani, A. F. Shoukry and R. M. El Nashar, Analyst, 2001, 126, 79 RSC.
- Theoretical Electrochemistry, ed. L. I. Antropov and Mir, Moscow, 1972 Search PubMed.
- Statistics for Analytical Chemistry, ed. J. C. Miller and J. N. Miller, Ellis Horwood, Chichester, UK, 3rd edn, 1993 Search PubMed.
|
This journal is © The Royal Society of Chemistry 2015 |
Click here to see how this site uses Cookies. View our privacy policy here.