DOI:
10.1039/C5RA16050B
(Paper)
RSC Adv., 2015,
5, 95960-95966
Characterizing the distribution of organic matter during composting of sewage sludge using a chemical and spectroscopic approach†
Received
10th August 2015
, Accepted 2nd November 2015
First published on 2nd November 2015
Abstract
The present study aimed to investigate the evolution of organic matters during sewage sludge composting with different carbon additives including glucose, sucrose and straw. The organic matters were divided into a hot water soluble fraction (SOL-H2O), neutral detergent soluble fraction (SOL-ND), hemicellulose-like fraction (HEM), cellulose-like fraction (CEL) and lignin-like fraction (LIG) by a modified Van Soest procedure. Parallel factor (PARAFAC) analysis was applied to identify the number of fluorescent components in excitation–emission matrixes (EEMs) and its relative concentration in each water extractable organic matter (WEOM) sample. The results showed that the degradation of total organic carbon (TOC) in SOL-H2O and SOL-ND, as well as HEM and CEL fractions was accelerated by adding glucose and sucrose. While the straw powder increased the amount of TOC in the HEM and CEL fractions, no promotion on the degradation of TOC was observed. Four components including humic-like, tryptophan-like, microbial byproduct-like and fulvic-like substances were identified by PARAFAC analysis of EEMs. The percentage of humic-like substances in the composted material increased from 42.7% to 51.6% due to the addition of sucrose. The results suggested that adding glucose and sucrose in sewage sludge composting could promote the degradation and humification of organic matters.
1 Introduction
Composting is a process of biodegradation and humification in which organic matters are transformed into inorganics and stable organic substances by microorganisms under control conditions. The raw organic matters are converted into biologically stable and nutrient-rich materials which can be safely applied to improving soil physical and chemical quality. At the same time, large amounts of CO2, H2O and NH3 are emitted during the oxidation of the organic matters. The emission of NH3 can lead to odor pollution and reduction of fertilizer efficiency. Several factors, such as pH, temperature, C/N ratio, moisture content, aeration, etc., impact NH3 emission rate. Theoretically, the initial C/N ratio of sewage sludge is low (6–10), as the excess nitrogen is easily lost in the form of NH3 during thermophilic composting. Thus, addition of extra-carbon source could increase C/N ratio, which seems to be a solution to this problem. However, according to our previous results, the NH3 emission were correlative with the degradation degree of organic matters.1
The addition of extra-carbon source not only increases the C/N ratio of composting materials, but also affects the transformation of organic matter. Although monitoring transformation of organic matter always plays an important role in evaluating the performance of composting process, much attention was paid to the effects of bulking agent and the running condition on compost process.2,3 There is little information available in literature regarding the evolution of organic matter in the sewage sludge with different extra-carbon sources. Shin and Jeong4 found that C/N ratio of composting materials was not convictive enough to describe the decomposition pattern and bio-availability of C and N presented in organic matter. The composting substrates with similar C/N ratios but various chemical compositions showed a significant difference in organic matter evolution. The organic matter can be divided into several components based on its biodegradability, including readily degradable substrate (water soluble material), slowly degradable substrate (hemicelluloses and cellulose), and resistant degradable substrate (lignin).5,6 The chemical composition of organic matter had significant influence on microbial diversity. Chang and Chen7 indicated that the period for composts reaching maturity could increase when a large amount of lignin-based bulking agent was presented in composting substrate.
In recent years, spectroscopic techniques such as FTIR, UV, NMR and fluorescence spectroscopy have been used to investigate the variations of organic matter structure during composting process. Among these techniques, fluorescence spectroscopy combined with parallel factor (PARAFAC) analysis is widely used in tracking protein-like, fulvic acid-like and humic acid-like substances dynamics.8 The PARAFAC analysis was first proposed by Bro,9 who commented that PARAFAC was one of suitable decomposition methods for multi-way data. The author discussed different multivariate decomposition methods on analysing excitation–emission matrix (EEM) data, and demonstrated that PARAFAC could successfully divided complex EEM data into individual fluorescence component. Moreover, several studies suggested that the EEM companied with PARAFAC is an accurate method to evaluate composts stability.10–13
The purpose of this study is to investigate the effects of carbon sources additives on the evolution of organic matters during sewage sludge composting process. Six composting experiments with different carbon additives (including glucose, sucrose and straw) were conducted using laboratory-scale composting reactors. Chemical and spectroscopic features of the organic matters were analyzed by a modified van Soest procedure and fluorescence spectroscopy as EEM.
2 Materials and methods
2.1 Composting process
The detail descriptions of composting procedure could be found in the paper by li1 and is briefly presented below. Sewage sludge was mixed with different carbon additives. The additives of experiment R1 to R6 were glucose, sucrose, straw powder, straw powder and glucose, straw powder and sucrose and blank respectively. Glucose, sucrose and straw powder were all added at the ratio of 50
:
1 (sludge
:
carbon source). Sawdust was used as bulking agent to ensure the suitable moisture content and porosity of composting materials. The mixing ratio of sludge
:
sawdust was 6
:
1 (wet weight).14 To simulate a natural self-heating process of compost, the temperature procedure was operated according to Lashermes.15 During the 22 days composting, samples were collected at 0, 72, 144, 518 hours.
2.2 Chemical analysis
Moisture content was determined by weight loss of the compost sample after drying at 105 °C for 24 h. The total organic carbon was detected following the standardized method of Walkley–Black wet combustion method. According to the chemical structural of substrate, the organic matter could be divided into five components: (1) hot water soluble fraction (SOL-H2O); (2) neutral detergent solution fraction (SOL-NDS); (3) hemicellulose-like fraction (HEM); (4) cellulose-like fraction (CEL); (5) lignin-like fraction (LIG). The distribution of these fractions in composting substrates was determined using a modified version of van Soest.16 Before analysis, all samples were drying at 60 °C to eliminate organic matter loss caused by heating. The hot water soluble fraction was the weight loss after extracting with water at 100 °C for 30 min. Next procedure was to extract the residue with neutral detergent solution at 100 °C for 60 min. The fraction dissolved in neutral detergent solution was neutral detergent solution fraction. Similarly, the residue was extracted by acid detergent solution and the weight loss after drying was hemicellulose-like fraction. The fraction which was not soluble in acid detergent solution but soluble in 72% H2SO4 was named as cellulose-like fraction. The last procedure was to burn the residual which contained the lignin-like fraction and inert materials. After burning, the residue ash was inert materials.
The distribution of C within the different fractions was measured and expressed as a percentage of total organic carbon (TOC). The biochemical fractions were expressed as a% of DM (dry matter).
2.3 Spectroscopic analysis
Fluorescence determination and analysis techniques referred to Yu11 and Tian.8 Water extractable organic matter (WEOM) was obtained by mixing the composting samples with deionized water (solid to water ratio of 1
:
50) and keeping 120 rpm oscillation frequency for 24 h at room temperature. The extracts of composting samples were centrifuged at 8000 rpm for 10 min. Afterward, the extracts were filtered using 0.45 μm membrane filter and the filtrates were further diluted to adjust dissolved organic carbon (DOC) to 12 mg L−1.13 Fluorescence EEMs were measured with Jasco FP-6500 Spectrofluorometer. The excitation wavelength was set from 220 to 450 nm with a 5 nm increment, while the emission wavelength increased from 250 to 500 nm with a 2 nm increment. The scan speed was 1200 nm min−1, using excitation and emission slit monochromators of 5 nm. The voltage of the photomultiplier tube (PMT) was set at 700 V for low level light detection. The temperature of the samples was maintained at room temperature (20 °C) during the analyses. The spectrum of pure water was used as the blank and it was subtracted from the EEMs of all samples examined to decrease the influence from the first and second-order Rayleigh and Raman scattering. Each scan data of EEM was composed of 151 Em (row) × 47 Ex (column) wavelengths. The EEMs datas were handled by PARAFAC model which is a three-way decomposition method. The PARAFAC analysis was carried out on MATLAB 6.5 (Mathworks) according to Stedman,17 and the relative algorithms and source code could be load at website of http://www.models.life.ku.dk/algorithms. Based on the PARAFAC model, the fluorescence EEMs are arranged in a three-way array named as X with I × J × K, where I is the number of samples, J is the number of emission wavelengths, K is the number of excitation wavelengths. The detail descriptions of PARAFAC analysis could be found elsewhere.9 The concentration of each component was calculated with following equations.13,18 |
In = scorenExn(λmax)Emn(λmax)
| (1) |
|
 | (2) |
In is the fluorescence intensity of component n; scoren is the relative fluorescence intensity of component n obtained from PARAFAC analysis; Exn(λmax) is the max excitation loading for component n and Emn(λmax) is the max emission loading for component n; ITOT is the sum of the fluorescence intensity of all components; Pn is the percentage of the component n.
3 Results and dicussion
3.1 Compost properties
In our study, the peak temperature values were observed around 72 h in the six composting reactors. The thermophilic phase (T > 55 °C) was maintained for 60 hours because of the smaller size of the laboratory-scale reactor. The temperature differences among the six runs were not significant since the amount of additive carbon sources was not sufficient to generate enough energy to influence the pile temperature.
The initial pH decreased in all runs except the straw powder one which might be caused by bioconversion of glucose and sucrose into acid intermediates species.19 Then pH increased from 8.0–8.5 in all runs on the third day. This increase might be the result of the formation of ammonium ions during protein decomposition.20 Afterward, a combined effect of both ammonia volatilization and biological oxidation of nitrogen to nitrites or nitrates led to the pH change towards neutrality.21
The variation of moisture content maintained within normal range. The moisture contents of all runs fell below 60% at the end of composting which lead to volume reduction of sludge. Slightly lower moisture content than the control was observed in the runs with addition of readily biodegradable carbon sources because of more respiration heat production from organic biodegradation.
3.2 C distributions in different soluble fractions
Fig. 1 shows the quantitative analysis of TOC amounts in different biochemical fractions. Among the five fractions, SOL-H2O and ND-H2O fractions belong to readily degradable substances which can be preferentially used by microorganisms. Water environment is the mass transport media for series of microbial reactions. So many researchers evaluated the stability of compost products through studying the variation of water-soluble components.22,23
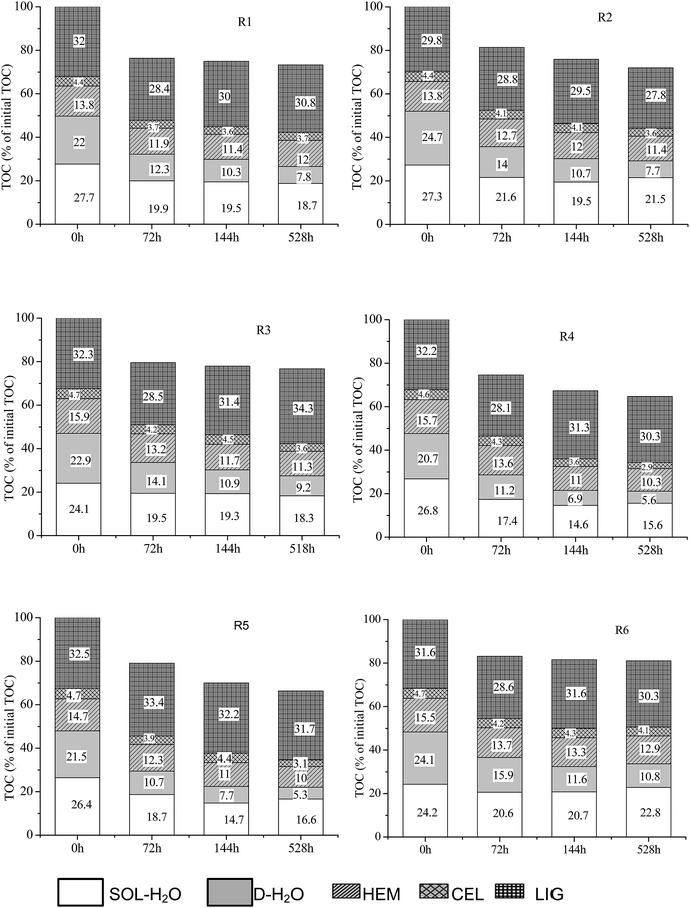 |
| Fig. 1 Evolution of TOC amounts in the biochemical fractions estimated by the van Soest procedure (expressed as a% of the initial TOC amount). | |
At the beginning of composting progress, the amount of SOL-H2O fraction rapidly decreased in the six treatments, and the declining trend slowed down until the thermophilic phase. In R1, R2, R4 and R5, the TOC percentage of SOL-H2O fraction was 27.7%, 27.3%, 26.8% and 26.4% at the beginning respectively, much higher than that in the R3 and R6 due to the addition of glucose and sucrose. However, the TOC percentages of SOL-H2O fraction in the above runs became lower than R3 and R6 at the end of composting. It indicates that the glucose and sucrose added were rapidly degraded by microorganisms, and meanwhile the consumption of the readily degradable organic matter in the original part of material could be accelerated as well.
For SOL-H2O fraction, an obvious decline was observed during the heating phases and the thermophilic phase. The composition of SOL-ND section is usually grease-like substances, so there is no significant impact on the TOC percentage of SOL-ND section after adding glucose and sucrose. However, at the end of composting, in R1, R2, R4 and R5, the TOC percentage of SOL-ND fraction was 7.8%, 7.7%, 5.6% and 5.3% respectively, which were much lower than that of R3 and R6. It was similar to the phenomena observed in SOL-H2O, as well as HEM and CEL fractions. This result indicates that the carbon sources additives not only promoted the degradation of readily biodegradable carbon sources, but also accelerated the biodegradation of hemicellulose and cellulose. This promotion was similar with the co-metabolic biodegradation, which was described as that addition of the readily biodegradable carbon sources could enhance the consumption of organic matters in original material.24
HEM and CEL substances are the main components of straw waste, such as straw and corn stalks. In R3, R4 and R5, the TOC of HEM and CEL fraction increased because of straw addition. Degradation of HEM and CEL fractions mainly began at the latter period of thermophilic phase. Most of the carbon sources needed by the reactions occurred at the later period of composting was provided by the degradation of HEM and CEL substances provided.3 This might explain that the percentage of the SOL-H2O TOC increased at the end of composting. Eklind, Y25 found that the degradation of HEM and CEL substances was closely related with the component of substrate. The main component of the CEL fraction in the straw is lignocellulose. The maturity progress could be directly affected by the degradation of macromolecular organic compounds like cellulose and lignin. The highest TOC percentage of LIG fraction was observed in all runs, but the TOC of this fraction decreased slightly since the low amount of lignin was degraded during the cooling phase. It could be related with the complicated structure of lignin.
Degradation of soluble fractions was mainly conducted at the heating and thermophilic phases as described above. Nitrogen loss was also mainly occurred during these phases through ammonia volatilization. Researchers have proposed that ammonia volatilization could be reduced by improving C/N ration through adding carbon sources in previous studies.20,26 Although increasing the C/N ratio can be achieved by adding different kinds of carbon additives, their impacts on degradation process of organic carbon were different. In another word, bioavailability of different carbon sources is varied. Some carbon sources were difficult to be biodegraded such as straw, rice straw, leaves and sawdust, while their effects on control of N loss were not satisfactory.27 In addition, composting reaction period was extended and running cost was increased. Therefore, the bio-availability the carbon sources could affect the nitrogen loss.
3.3 PARAFAC analysis of EEM spectra
3.3.1 EEM contours of WEOM from different composts. The EEM contours of WEOM from the treatments of R1–R6 before and after composting were shown in Fig. 2. In general, the fluorescence peaks observed in water samples can be divided into five Regions, including: Region I (Ex/Em = 200–250/250–330), Region II (Ex/Em = 200–250/330–380), Region III (Ex/Em = 250–340/250–380), Region IV (Ex/Em = 250–400/380–450) and Region V (Ex/Em = 200–250/380–450). According to Henderson28 and Hao,29 Region I, II were related to simple aromatic proteins, such as tryptophan and tyrosine, Region III belonged to microbial byproduct-like substances, whereas Region IV belonged to humic-like substance and Region V was reported as fulvic-like substance. Obviously, peaks could be observed only in Regions I, II and III in the samples taken before composting. The existence of these fluorescence EEM peaks indicated that the composting materials were unstable because of the existence of a large number of protein-like substances. As the composting process proceeded, the peaks in Region II and Region III disappeared because of the degradation of protein-like substances by microorganisms. After composting, two dominate peaks could be identified in Region IV and V of each sample EEM contours. We attributed this phenomenon to the reason that the readily degradable organic matters were converted to stable humic-like substances. Several investigators have observed similar peaks in composting samples.30,31 According to previous studies, the presence of the peaks in Regions IV and V is associated with the humic-like substances and indicates the degree of humification and maturation.32 In the treatment of R1, R2, R4 and R5, the fluorescence intensities in the Regions IV and V were higher than those in the treatments of R3 and R6, in which no glucose and sucrose were added. The possible explanation was that addition of glucose and sucrose could enhance microbial activity and thus accelerated maturity process of composting. There is evidence that the formation of humic-like substance is related to microbial activity and degradation of organic matter.8,33 Therefore, the results of fluorescence EEMs showed that addition of carbon sources influenced the degradation and maturity process of organic matter in the compost products.
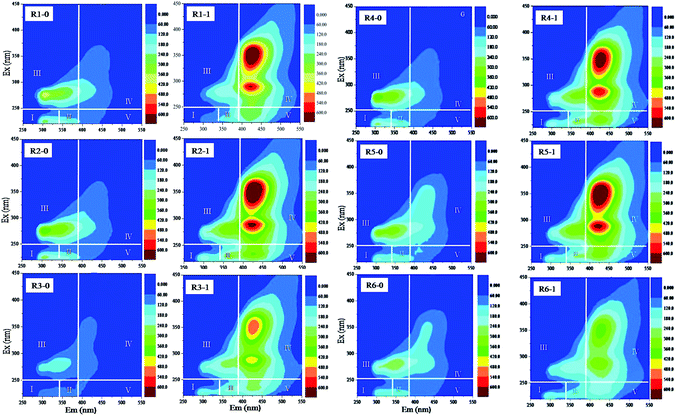 |
| Fig. 2 The EEM spectra of WEOM at different stages of composting. | |
3.3.2 PARAFAC analysis. The analysis presented above is qualitative determination of EEM contours by visual observation. However, it is not hard to see that there is distinct overlapping phenomenon among peaks. To accurately measure the characterization of EEM contours and peaks intensity, PARAFAC analysis has been applied. Recent studies have indicated that PARAFAC analysis can provide a useful way of analysis multi-way spectral data.34The analysis result is sensitive to the number of components. The core consistency diagnostic is a suggested approach to find the appropriate number of components.35 The core consistency of the model can give a realistic description of the data. It should be close to 100%. According to the results of the core consistency diagnostic (Fig. 4), the core consistency decreased from approximately 100–60% when the number of components changed from 3 to 4 or 5. Then the residual sum of squares method was used to test whether the numbers of components were reasonable. Generally, a higher number of components has a lower residual sum of squares. When no significant changes can be observed on the residual sum of squares, there is no need to increase the number of components. Based on the results of residual sum of squares, four-component model had lower residual sum of squares than three-component model. Simultaneously, when the number increased from 4 to 5, small change occurred on the residual sum of squares. Hence, the four-component model for PARAFAC analysis was optimal.
After determining the number of components, the PARAFAC model outputted the fluorescence peak positions and intensities (Fig. 3). All the EEM contours of composting samples could be distinctly divided into four components, including component 1 [Ex/Em = 355/426], component 2 [Ex/Em = 275/306], component 3 [Ex/Em = 290/390] and component 4 [Ex/Em = 250/478]. According to Chen,36 component 1 was originated from humic-like substances, component 4 was associated with fulvic-like substances, whereas component 2 belonged to tryptophan-like substances and component 3 was similar to the terrestrial humic-like substances.37,38 ARAFAC analysis can provide quantitative information about the distribution of different components. The distribution of Pn for the various components in each sample is shown in Fig. 4.
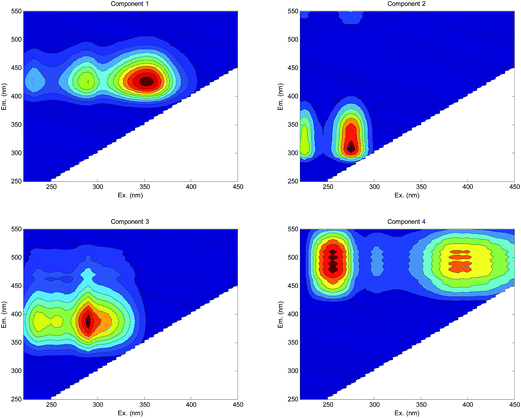 |
| Fig. 3 The EEM spectra of the four components identified by PARAFAC analysis. | |
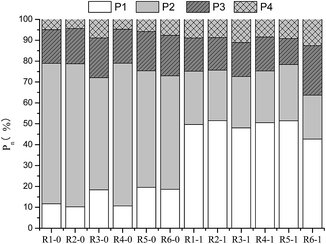 |
| Fig. 4 Distribution of the percentage of the four components in each sample. | |
P1, P2, P3 and P4 represented the fluorescence intensity of component 1, 2, 3 and 4, respectively. Obviously, the fluorescence intensity of component 1 and 4 increased significantly, whereas component 2 decreased after composting. This result indicates that the protein-like substances were rapidly degraded by composting microorganisms, meanwhile, a large amount of humic-like substances was generated.
During the composting, humification reactions were closely related with the degradation of readily degradable substances such as carbohydrates.39 The formation of humic substances was caused by the reactions of polymerization/repolymerization which were determined by microbial activity and enzymes.40 The percentages of humic-like substances were 51.5% and 51.6% in R2 and R5, which were higher than the control experiment R6 of 42.7%. This ratio seemed to be most sensitive to describe the humification degree, as reported by several authors.41 One possible reason for this is that humification process could be promoted by addition of readily degradable carbon sources. This point could also be illustrated from the analysis of Fig. 2. The higher intensity of the fluorescence peaks in Regions IV and V was observed in the treatment of experiments in which readily degradable carbon additives (glucose and sucrose) were added. On the other hand, microbial activity was stimulated because of addition of sucrose and glucose as mentioned above. The conversion rate of organic matter, which was closely related to the humification process, was promoted as well.
4 Conclusions
The evolution of organic matter during co-composting of sewage sludge with various carbon additives were characterized by both C mass loss in biochemical fractions and Fluorescence EEMs. The results indicated that addition of glucose and sucrose increased the amount of C in SOL-H2O fraction by 12.6% and 11.4% compared with the control group. The addition of straw increased the amount of C in HEM and CEL fractions. The addition of mixture of sucrose and straw promoted the carbon degradation in all biochemical fractions except LIG fraction. The PARAFAC analysis showed that the protein-like substances were rapidly biodegraded, and a large amount of humic-like substances was generated after composting. Sucrose and straw were the best carbon sources which could promoted the maturity progress. Furthermore, the results suggested that the readily degradable carbon additive had a better performance on degradation and humification of organic matters in composting process as compared with straw.
Acknowledgements
This work was financially supported by National Natural Science Foundation of China (51278146, 51408151), Major Science and Technology Program for Water Pollution Control and Treatment (2013ZX07201007), National Water Pollution Control and Treatment Science and Technology Major Project (2014ZX07202-011), Specialized Research Fund for the Doctoral Program of Higher Education (20132302120075).
Notes and references
- Y. Li, W. Li, B. Liu, K. Wang, C. Su and C. Wu, Int. Biodeterior. Biodegrad., 2013, 85, 624–630 CrossRef CAS.
- E. K. Lhadi, H. Tazi, M. Aylaj, P. L. Genevini and F. Adani, Bioresour. Technol., 2006, 97, 2117–2123 CrossRef CAS PubMed.
- J. Doublet, C. Francou, M. Poitrenaud and S. Houot, Waste Manag., 2010, 30, 1922–1930 CrossRef CAS PubMed.
- H.-S. Shin and Y.-K. Jeong, Environ. Technol., 1996, 17, 433–438 CrossRef CAS.
- A. Mottet, E. François, E. Latrille, J. P. Steyer, S. Déléris, F. Vedrenne and H. Carrère, Chem. Eng. J., 2010, 160, 488–496 CrossRef CAS.
- L. Zhao, W.-M. Gu, P.-J. He and L.-M. Shao, Water Res., 2011, 45, 2322–2330 CrossRef CAS PubMed.
- J. I. Chang and Y. J. Chen, Bioresour. Technol., 2010, 101, 5917–5924 CrossRef CAS PubMed.
- W. Tian, L. Li, F. Liu, Z. Zhang, G. Yu, Q. Shen and B. Shen, Bioresour. Technol., 2012, 110, 330–337 CrossRef CAS PubMed.
- R. Bro, Chemom. Intell. Lab. Syst., 1997, 38, 149–171 CrossRef CAS.
- J. B. Fellman, M. P. Miller, R. M. Cory, D. V. D'Amore and D. White, Environ. Sci. Technol., 2009, 43, 6228–6234 CrossRef CAS PubMed.
- G.-H. Yu, Y.-H. Luo, M.-J. Wu, Z. Tang, D.-Y. Liu, X.-M. Yang and Q.-R. Shen, Bioresour. Technol., 2010, 101, 8244–8251 CrossRef CAS PubMed.
- X. Guo, X. He, H. Zhang, Y. Deng, L. Chen and J. Jiang, Microchem. J., 2012, 102, 115–122 CrossRef CAS.
- X.-S. He, B.-D. Xi, X. Li, H.-W. Pan, D. An, S.-G. Bai, D. Li and D.-Y. Cui, Chemosphere, 2013, 93, 2208–2215 CrossRef CAS PubMed.
- Y. B. Li, W. G. Li, C. D. Wu and K. Wang, Bioresour. Technol., 2013, 136, 385–393 CrossRef CAS PubMed.
- G. Lashermes, E. Barriuso, M. Le Villio-Poitrenaud and S. Houot, Waste Manag., 2012, 32, 271–277 CrossRef CAS PubMed.
- P. J. van Soest and R. H. Wine, J. - Assoc. Off. Anal. Chem., 1967, 50, 50–55 CAS.
- C. A. Stedmon, R. Bro, C. A. Stedmon and R. Bro, Limnol. Oceanogr.: Methods, 2008, 6, 572–579 CrossRef CAS.
- C. A. Stedmon, S. Markager and R. Bro, Mar. Chem., 2003, 82, 239–254 CrossRef CAS.
- P. M. Ndegwa, S. A. Thompson and K. C. Das, Bioresour. Technol., 2000, 71, 5–12 CrossRef CAS.
- L. Wang, Y. Zhang, J. Lian, J. Chao, Y. Gao, F. Yang and L. Zhang, Bioresour. Technol., 2013, 136, 281–287 CrossRef CAS PubMed.
- A. Yadav and V. K. Garg, Rev. Environ. Sci. Biotechnol., 2011, 10, 243–276 CrossRef CAS.
- G. Gigliotti, K. Kaiser, G. Guggenberger and L. Haumaier, Biol. Fertil. Soils, 2002, 36, 321–329 CrossRef CAS.
- N. Hartlieb, B. Marschner and W. Klein, Sci. Total Environ., 2001, 278, 1–10 CrossRef CAS PubMed.
- B. C. Qi, C. Aldrich, L. Lorenzen and G. M. Wolfaardt, Ind. Eng. Chem. Res., 2004, 43, 6309–6316 CrossRef CAS.
- Y. Eklind and H. Kirchmann, Bioresour. Technol., 2000, 74, 125–133 CrossRef CAS.
- S. M. Troy, T. Nolan, W. Kwapinski, J. J. Leahy, M. G. Healy and P. G. Lawlor, J. Environ. Manage., 2012, 111, 70–77 CrossRef CAS PubMed.
- M. A. Chowdhury, A. de Neergaard and L. S. Jensen, Biosystems Eng., 2014, 124, 63–77 CrossRef.
- R. K. Henderson, A. Baker, K. R. Murphy, A. Hambly, R. M. Stuetz and S. J. Khan, Water Res., 2009, 43, 863–881 CrossRef CAS PubMed.
- R. Hao, H. Ren, J. Li, Z. Ma, H. Wan, X. Zheng and S. Cheng, Water Res., 2012, 46, 5765–5776 CrossRef CAS PubMed.
- D. Said-Pullicino, F. G. Erriquens and G. Gigliotti, Bioresour. Technol., 2007, 98, 1822–1831 CrossRef CAS PubMed.
- G.-H. Yu, M.-J. Wu, Y.-H. Luo, X.-M. Yang, W. Ran and Q.-R. Shen, Waste Manag., 2011, 31, 1729–1736 CrossRef CAS PubMed.
- F. C. Marhuenda-Egea, E. Martínez-Sabater, J. Jordá, R. Moral, M. A. Bustamante, C. Paredes and M. D. Pérez-Murcia, Chemosphere, 2007, 68, 301–309 CrossRef CAS PubMed.
- M. del Carmen Vargas-García, F. Francisca Suárez-Estrella, M. José López and J. Moreno, Process Biochem., 2006, 41, 1438–1443 CrossRef.
- B. Hua, F. Dolan, C. McGhee, T. E. Clevenger and B. Deng, Int. J. Environ. Anal. Chem., 2007, 87, 135–147 CrossRef CAS.
- C. M. Andersen and R. Bro, J. Chemom., 2003, 17, 200–215 CrossRef CAS.
- W. Chen, P. Westerhoff, J. A. Leenheer and K. Booksh, Environ. Sci. Technol., 2003, 37, 5701–5710 CrossRef CAS PubMed.
- X. Yao, Y. Zhang, G. Zhu, B. Qin, L. Feng, L. Cai and G. Gao, Chemosphere, 2011, 82, 145–155 CrossRef CAS PubMed.
- H. Wu, Z. Zhou, Y. Zhang, T. Chen, H. Wang and W. Lu, Bioresour. Technol., 2012, 110, 174–183 CrossRef CAS PubMed.
- L. El Fels, M. Zamama, A. El Asli and M. Hafidi, Int. Biodeterior. Biodegrad., 2014, 87, 128–137 CrossRef CAS.
- U. Tomati, E. Madejon and E. Galli, Compost Sci. Util., 2000, 8, 108–115 CrossRef.
- A. Vergnoux, M. Guiliano, Y. Le Dreau, J. Kister, N. Dupuy and P. Doumenq, Sci. Total Environ., 2009, 407, 2390–2403 CrossRef CAS PubMed.
Footnote |
† Electronic supplementary information (ESI) available. See DOI: 10.1039/c5ra16050b |
|
This journal is © The Royal Society of Chemistry 2015 |