DOI:
10.1039/C5RA15906G
(Paper)
RSC Adv., 2015,
5, 73217-73224
Effects of terminal group and chain length on temperature-responsive chromatography utilizing poly(N-isopropylacrylamide) synthesized via RAFT polymerization†
Received
8th August 2015
, Accepted 24th August 2015
First published on 24th August 2015
Abstract
Poly(N-isopropylacrylamide) with two chain lengths (MW: ca. 5000, and ca. 20
000 g mol−1) was synthesized by reversible addition-fragmentation chain transfer (RAFT) polymerization, using 2-dodecylsulfanylthiocarbonylsulfanyl-2-methyl propionic acid as the chain transfer agent. The derivatization of terminal dodecyl groups to maleimide groups was performed through the reduction of terminal trithiocarbonate moieties. These polymers were grafted onto aminopropyl silica using an activated ester–amine coupling method. Five types of polymer modified silica beads were prepared, i.e., long chain length, short chain length, and mixed chain length functionalized with a dodecyl terminal group, and long chain length and short chain length with a maleimide terminal group. The surface properties of the modified polymer silica beads were evaluated by employing them as the stationary phase in HPLC setups and examining the temperature-dependent elution profiles of five steroids. The retention factor of steroids became smaller when the terminal dodecyl group was substituted with a maleimide group. A hydrophobic dodecyl moiety on the outermost surface strongly affected the retention of steroids, and the retention factor of steroids on short chains was larger than that on long chains below the lower critical solution temperature. These results indicate that the chain length and terminal functional group on the outermost surface of the polymer have a critical effect on the characteristics of polymer modified silica bead interfaces.
Introduction
Poly(N-isopropylacrylamide) (PNIPAAm) is one of the most studied temperature-responsive polymers, and exhibits temperature-dependent phase transition in aqueous solutions at its lower critical solution temperature (LCST) of 32 °C.1 PNIPAAm chains are hydrated and hydrophilic below the LCST and dehydrated and hydrophobic above the LCST, which means that its hydrophobicity/hydrophilicity can be controlled by temperature.2,3 Taking advantage of this characteristic, temperature-responsive chromatography systems based on a PNIPAAm-modified stationary phase were developed.4 In these systems, only aqueous solution is used as the mobile phase, but the elution properties can be controlled by external temperature, as the hydrophilic/hydrophobic nature of the surface of the stationary phase changes with temperature.
The LCST and hydrophobicity/hydrophilicity of PNIPAAm can be precisely controlled by the copolymer composition.5,6 In previous studies, PNIPAAm has been functionalized with external stimuli-responsivity to pH,7 ions,8 saccharides,9 and light10 by copolymerization with functional monomers. Furthermore, our group has developed temperature-responsive chromatography systems based on PNIPAAm-based polymers functionalized with butyl methacrylate,11,12 N,N-dimethylaminopropylacrylamide,13,14 and N-acryloyl-3-(2-naphthyl)-L-alanine methyl ester moieties.15 Temperature-responsive separation based on hydrophobic,11,12 charge,13,14 and π–π15 interactions were achieved with these polymers.16,17
Polymer characteristics are also affected by their terminal functionalities.18,19 PNIPAAm with hydrophobic terminal groups exhibits a lower LCST shift compared to the LCST of native PNIPAAm.20–22 Furthermore, when the terminal group is on the outermost surface in, for example, micelles, it affects the LCST more strongly.23,24 Terminal functionalization of PNIPAAm brushes on glass surfaces has also been evaluated for its effect on cell adhesion and detachment. Matsuzaka et al. reported that cell adhesion to hydrophobic dodecyl terminated PNIPAAm brush surfaces was initiated at 31 °C, while their adhesion was significantly suppressed on hydrophilic maleimide terminated PNIPAAm brush surfaces below 33 °C.25 As mentioned above, the nature of the terminal functional group is as a factor that determines the function of the polymers.
In recent decades, much research attention has been paid to the application of controlled/living radical polymerization,26 especially atom transfer radical polymerization (ATRP),27 and reversible addition-fragmentation chain transfer (RAFT).28 For example, Nagase et al. developed PNIPAAm-based temperature-responsive polymer brush surfaces on silica beads29 and monoliths.30 These materials were applied to all-aqueous temperature-responsive chromatography systems for the separation of biomolecules, peptides, and proteins.31–33 Also, the effects of polymer brush density and chain length on separation and stability have been evaluated.34,35
Work focusing on the relationship between the performance of HPLC separation systems and the surface characteristics of polymer brush surfaces prepared via ATRP has been published; however, to the best of our knowledge, there have been no reports on the relationship between the performance of HPLC separation systems and the surface characteristics of polymer grafted surfaces prepared via RAFT polymerization. Therefore, we have focused on the effect of the terminal functional group and chain length of PNIPAAm via RAFT polymerization grafted onto silica beads on the HPLC separation of steroids.
Two types of PNIPAAm with two different chain lengths bearing hydrophobic dodecyl trithiocarbonate terminal groups were synthesized via RAFT polymerization. The terminal dodecyl trithiocarbonate groups of these polymers were transformed to maleimide group by reduction. By these methods five types of PNIPAAm-grafted stationary phase silica beads were developed: long, short, and mixed chain length with a dodecyl terminal group, and long or short chain length with a maleimide terminal group. The five types of PNIPAAm grafted silica beads were evaluated as temperature-responsive aqueous chromatographic stationary phases for the separation of steroids.
Experimental
Materials and chemicals
N-Isopropylacrylamide (NIPAAm) was kindly provided by KJ chemicals (Tokyo, Japan), and was purified by recrystallization from n-hexane and dried at 25 °C in a vacuum prior to use. N,N-Dimethylformamide (DMF), 1,4-dioxnane, dichloromethane, and 2,2′-azobisisobutyronitrile (AIBN) were purchased from Wako Pure Chemical Industries (Osaka, Japan). 2-Dodecylsulfanylthiocarbonylsulfanyl-2-methyl propionic acid (DMP), maleimide, and sodium hydrosulfite (Na2S2O4) were purchased from Sigma-Aldrich (St Louis, MO, USA). N,N′-Dicyclohexylcarbodiimide (DCC) was purchased from Kanto Chemicals (Tokyo, Japan). Sodium borohydride (NaBH4) was purchased from Tokyo Chemical Industry Co., Ltd (Tokyo, Japan). N-Hydroxysuccinimide (NHS) was purchased from Merck Japan (Tokyo, Japan). Aminopropyl silica (average diameter of 5 μm) was purchased from Nishio Kogyo (Tokyo, Japan). The analytes (uracil, hydrocortisone, prednisolone, dexamethasone, hydrocortisone acetate, and testosterone) were purchased from Wako Pure Chemical Industries.
Water was distilled and passed through a Milli-Q purification system (Millipore, Bedford, MA, USA). All other reagents and solvents were of analytical grade.
Synthesis of dodecyl terminated PNIPAAm
NIPAAm (10.0 g, 88.4 mmol) was dissolved in 20 mL DMF. Then, AIBN (16.4 mg, 0.1 mmol), DMP (182 mg, 0.5 mmol) and the internal standard of 1,3,5-trioxane (0.331 g, 3.67 mmol) were added to the solution. The reaction mixture was degassed by bubbling with nitrogen for 30 min, and then heating to 70 °C for 5 h. The monomer conversion of 95.1% was determined by 1H NMR analysis. The reaction solution was poured into diethyl ether to precipitate the polymer. The crude product was further purified by repeated precipitation from an acetone solution into diethyl ether. The resulting residue was dissolved in water and dialyzed for 3 days using a 3500 Da MWCO dialysis membrane (Spectra/Por, Spectrum Laboratories, CA, USA). After freeze-drying, a pale yellow powder was obtained. The polymer was designated 20
000-D, where 20
000 represents the theoretical molecular weight, and D denotes dodecyl trithiocarbonate.
5000-D was synthesized using a similar procedure, but with NIPAAm (10 g, 88.4 mmol), DMF (20 mL), AIBN (65.7 mg, 0.4 mmol), DMP (729 mg, 2.0 mmol), and 1,3,5-trioxane (0.338 g, 3.75 mmol). Heating for 14 h. The monomer conversion of 98.6% was determined by 1H NMR analysis.
Transformation of terminal dodecyl moiety to maleimide
20
000-D (3.0 g, 0.15 mmol) was dissolved in 200 mL of 0.1 M carbonate–bicarbonate buffer solution (pH 9.0) containing maleimide (437 mg, 4.5 mmol), NaBH4 (2.84 g, 75.1 mmol), and sodium hydrosulfite (52.2 mg, 0.3 mmol), the reaction mixture was stirred for 20 h at 20 °C. After freeze-drying, a white powder was obtained. This was dissolved in acetone and filtered. The filtrate was concentrated under reduced pressure and the residue was dissolved in water, followed by dialysis for 3 days using a 3500 MWCO dialysis membrane. After freeze-drying, a white powder was obtained. The polymer was designated 20
000-M where 20
000 represents the theoretical molecular weight and M denotes maleimide.
The terminal dodecyl moiety of 5000-D was also transformed into maleimide using a similar procedure, but with 5000-D (2.5 g, 0.5 mmol), 0.1 M carbonate–bicarbonate buffer solution (200 mL), maleimide (1.46 g, 15.0 mmol), NaBH4 (9.46 g, 263 mmol), and sodium hydrosulfite (174 mg, 1.0 mmol).
Characterization of temperature-responsive polymers
1H NMR spectra were recorded at 500 MHz using a Varian INOVA-500 spectrometer. The molecular weights and polydispersity index (PDI) of the polymers were determined by gel permeation chromatography (GPC; GPC-8020 system; column: TSK-GEL; mobile phase: DMF containing 10 mM LiCl; TOSOH, Tokyo, Japan). Poly(ethylene oxide) standards were used for calibration. Differential scanning calorimetry (DSC) was performed with a DSC-60 Plus (SHIMADZU, Kyoto, Japan). The LCSTs were determined from the center of the endothermic peak.
The activation of the terminal carboxylic acid in the temperature-responsive polymers
20
000-D (2.5 g, 0.13 mmol) was dissolved in dichloromethane (30 mL). DCC (65 mg, 0.31 mmol) and NHS (36 mg, 0.31 mmol) were then added to the solution. The reaction mixture was stirred at 0 °C for 30 min and then at 25 °C for 24 h. After removal of the precipitated dicyclohexylurea by filtration, the filtrate was poured into diethyl ether to precipitate the terminal carboxylic acid activated 20
000-D, which was designated 20
000-D-SC.
Terminal carboxylic acids of 20
000-M, 5000-D, and 5000-M were activated using a similar procedure, changing the reactant quantities as follows:
20
000-M-SC: 20
000-M (2.5 g, 0.13 mmol), dichloromethane (30 mL), DCC (65 mg, 0.31 mmol), NHS (36 mg, 0.31 mmol). 5000-D-SC and 5000-M-SC: 5000-D or 5000-M (1.6 g, 0.32 mmol), dichloromethane (20 mL), DCC (165 mg, 0.80 mmol), NHS (92 mg, 0.80 mmol).
Modification of aminopropyl silica with temperature-responsive polymer
500 mg of 20
000-D-SC, 125 mg of 5000-D-SC, 500 mg of 20
000-M-SC, or 125 mg 5000-M-SC was dissolved in 20 mL 1,4-dioxane. 1.0 g of aminopropyl silica was then added to the solution. The reaction mixture was shaken at 25 °C for 24 h. This process was repeated three times. The resulting polymer-modified silica was washed with 100 mL of methanol and dried under vacuum at room temperature. The PNIPAAm-grafted silica beads are designated as 20
000-D-S, 5000-D-S, 20
000-M-S, and 5000-M-S.
In a similar way to the above-mentioned repeated process, a successive PNIPAAm grafting with 20
000-D-SC (once) and then 5000-D-SC (three times) was also carried out. The mixed long and short PNIPAAm-grafted silica beads are designated as Mix-D-S.
Characterization of temperature-responsive polymer modified silica beads
For obtaining surface elemental composition, X-ray photoelectron spectroscopy (XPS) measurement was performed for PNIPAAm-modified silica bead surfaces by an XPS (JPS-9010TR, JEOL Ltd, Japan).
To determine the amounts of grafted PNIPAAm, PNIPAAm-modified silica beads were analysed using a PE 2400 series CHNS/O analyser (PerkinElmer, Waltham, MA). Amount of grafted PNIPAAm on silica beads (g m−2) was calculated using the following equation29
where %
CP is the percent carbon increase from that of aminopropyl silica beads as determined by elemental analysis, and %
CP (calcd) is the calculated weight percent of carbon in the PNIPAAm,
S is the specific surface area of the aminopropyl silica beads. The graft of the PNIPAAm on the silica bead was estimated using
where
mp is the amount of grafted copolymer (g m
−2),
NA is Avogadro's number, and
Mn is the number-average molecular weight of the grafted PNIPAAm.
Temperature-responsive chromatography
The polymer-grafted silica beads were packed into a stainless-steel column (100 mm × 2.1 mm i.d.) using a flowing water/methanol solvent mixture (1
:
1) at 35 MPa for 1.5 h. The columns were connected to an HPLC system. HPLC analysis was carried out using a Hitachi D-7000 controller equipped with an L-7100 pump and an L-7405 UV detector (wavelength: 254 nm). The temperature of the column was controlled using an SSC2120 oven (Senshu Scientific Co., Ltd, Tokyo, Japan). The temperature responsive column was used at a flow rate of 0.5 mL min−1, and Milli-Q water was used as the mobile phase. Standard solutions of all analytes were prepared at a concentration of 0.1 mg mL−1. The retention factors of the analytes were calculated using the following equation:
Retention factor = (tR − t0)/t0 |
where t0 and tR are the retention time of uracil and the target analyte, respectively.
Results and discussion
The synthesis and characterization of temperature-responsive PNIPAAm via RAFT polymerization
PNIPAAm with two chain lengths having terminal dodecyl trithiocarbonate groups was synthesized by RAFT polymerization,36 as shown in Scheme 1. RAFT polymerization is a living radical polymerization method that can be used to synthesize well defined homo, gradient, triblock, and star polymers with predetermined molecular weights.28 Many kinds of RAFT chain transfer agents (CTAs) are commercially available, including dithiobenzoates, trithiocarbonates, and dithiocarbamates.37 RAFT polymerization can be used to produce polymers with a controlled molecular weight and a low polydispersity index when the appropriate RAFT agent is used.28
 |
| Scheme 1 Polymerization and terminal substitution of the temperature-responsive polymers. | |
In this work, RAFT polymerizations of NIPAAm were performed with the conventional azo-initiator AIBN as a radical initiator, and DMP as the CTA. DMP with a terminal dodecyl trithiocarbonate group and propionic acid group, which shows good compatibility with acrylamide type monomers,38 was selected for the polymerization of PNIPAAm. Two types of polymerization were conducted using 33 wt% monomer in DMF. The CTA
:
monomer ratios ([M]0/[CTA]0) were set to 177 and 44, as this would theoretically afford polymers with molecular weights of 20
000 and 5000 at 100% conversion. The CTA
:
initiator ratio ([I]0/[CTA]0) was fixed at 0.2. The characterization data of PNIPAAm are summarized in Table 1. PNIPAAm with both chain lengths was synthesized with low PDIs (1.08, and 1.14), and we found that GPC in DMF using poly(ethylene oxide) calibration standards tends to overestimate the molecular weight of PNIPAAm (27
600, and 8400 g mol−1).
Table 1 Physicochemical characteristics of the temperature-responsive polymers
Polymer |
Mn,NMRa |
Mn,GPCb |
PDIc |
LCST (°C) |
Number-averaged molecular weight estimated by 1H NMR. Number-averaged molecular weight estimated by GPC. Estimated by GPC. |
20 000-D |
19 700 |
27 600 |
1.08 |
30.5 |
20 000-M |
|
23 400 |
1.15 |
32.6 |
5000-D |
5000 |
8400 |
1.14 |
27.1 |
5000-M |
|
7900 |
1.12 |
33.6 |
The molecular weights of the PNIPAAm polymers were also estimated by 1H NMR (shown in the ESI†), by comparing the integrated proton signals derived from the NIPAAm methine (1H, 3.9 ppm), and the dodecyl methyl of DMP (3H, t, 0.90 ppm). The molecular weights of 20
000-D and 5000-D estimated by 1H NMR were determined to be 19
700 and 5000, respectively. Dodecyl trithiocarbonate groups were transformed to maleimide groups by adopting a one-pot reaction in which both terminal reduction and coupling with maleimide occurred,25 as shown in Scheme 1. The transformation of dodecyl trithiocarbonate to maleimide was confirmed by 1H NMR (shown in the ESI†) by the disappearance of the triplet signal derived from dodecyl methane. In addition, the transformation is apparent from the color change of the polymer powder from pale yellow to white. After the transformation, the molecular weight, and PDI of PNIPAAm with terminal maleimide group were comparable to PNIPAAm with terminal dodecyl group (Table 1).
The temperature-dependent phase transitions of 20
000-D, 5000-D, 20
000-M, and 5000-M aqueous solutions were examined using DSC, as shown in Fig. S1 in the ESI.† The LCSTs are determined from the center of the endotherm peaks of the polymer solutions. The LCSTs of the temperature-responsive polymers are summarized in Table 1. The LCST of PNIPAAm is known to be approximately 32 °C. However, the LCST of 20
000-D is 30.5 °C, and the LCST of 5000-D is 27.1 °C. The LCST of PNIPAAm can be affected by co-monomer composition,5 terminal group,22 and molecular weight.39 The contribution of the terminal dodecyl trithiocarbonate moiety increases in the case of 5000-D, which has a relatively short chain length. Therefore, while the LCST of 20
000-D is not significantly affected by the terminal moiety, that of 5000-D decreases due to the effect of hydrophobic end-group. The LCST of 5000-M is 33.6 °C, while that of 20
000-M is 32.6 °C. It has been reported that the LCST of PNIPAAm increases with reduced molecular weight.39 These results indicate that the terminal functional moiety and molecular weight have an influence on the physical properties of PNIPAAm.
Characterization of PNIPAAm-grafted silica beads
Temperature-responsive stationary phases were prepared by grafting silica beads with PNIPAAm of each terminal group and chain length. Terminal carboxyl groups derived from the DMP of PNIPAAm were used for immobilizing onto aminopropyl silica by activation with NHS. 20
000-D-SC, 5000-D-SC, 20
000-M-SC, and 5000-M-SC were grafted onto aminopropyl silica (Fig. 1). In addition, mixed chain length PNIPAAm-grafted silica beads were fabricated by successive grafting of PNIPAAm with long and then short chains with dodecyl terminal functional groups (schematic illustrations are shown in Fig. 2). For analysis of the elemental composition of the prepared silica-bead surfaces, XPS measurement was performed, as summarized in Table 2. After modification with PNIPAAm, the carbon and nitrogen content increases, and the silicone and oxygen content decreases, compared with aminopropyl silica. These results indicate that the aminopropyl silica beads were successfully modified with each PNIPAAm.
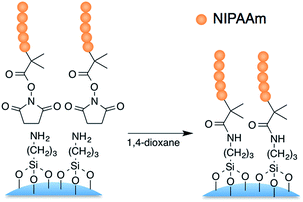 |
| Fig. 1 Schematic illustration of grafting of PNIPAAm onto aminopropyl silica beads. | |
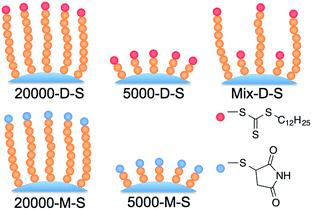 |
| Fig. 2 Schematic illustration of PNIPAAm-grafted silica beads with different polymer chain lengths and terminal functional groups. | |
Table 2 Elemental composition of PNIPAAm-modified silica beads by XPS analysis
Elemental composition (%) (n = 3) |
|
C |
N |
O |
Si |
20 000-D-S |
38.4 ± 0.3 |
3.6 ± 0.1 |
40.7 ± 0.1 |
17.3 ± 0.3 |
5000-D-S |
34.3 ± 0.4 |
3.7 ± 0.1 |
43.8 ± 0.2 |
18.2 ± 0.2 |
Mix-D-S |
38.9 ± 0.4 |
4.3 ± 0.1 |
39.6 ± 0.2 |
17.2 ± 0.2 |
20 000-M-S |
39.9 ± 0.2 |
3.9 ± 0.1 |
39.7 ± 0.1 |
16.6 ± 0.2 |
5000-M-S |
39.8 ± 0.8 |
4.3 ± 0.5 |
41.2 ± 0.5 |
14.7 ± 0.5 |
Aminopropyl silica |
24.4 ± 0.1 |
1.0 ± 0.1 |
51.2 ± 0.1 |
23.4 ± 0.2 |
Temperature-responsive chromatography utilizing PNIPAAm modified silica beads
The effect of column temperature on the elution behavior of the steroid analytes was evaluated. The structures and log
P values (the partition coefficients in the 1-octanol/water system) of the steroids are shown in Fig. 3. Fig. 4 shows the chromatograms of the steroids using the five types of temperature-responsive column with changing column temperature from 10 °C to 50 °C. Fig. 5 shows the temperature-dependent retention factors of each column derived from the retention times shown in Fig. 4. The retention times of the steroids on all columns increase with increasing temperature, and the elution order of steroids follows their log
P values, as well as those obtained in our previous temperature-responsive chromatography system.4,11,15 The temperature-responsive chromatography systems retained the steroids by hydrophobic interactions between the PNIPAAm-modified surfaces and the steroids. The effects of polymer chain length and terminal group on the temperature-responsive elution behavior of the steroids are discussed below.
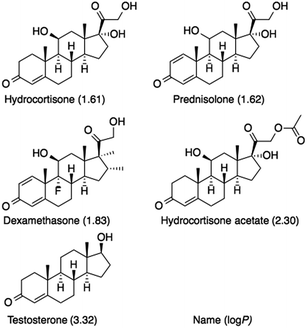 |
| Fig. 3 Chemical structures and log P values of the steroid analytes. | |
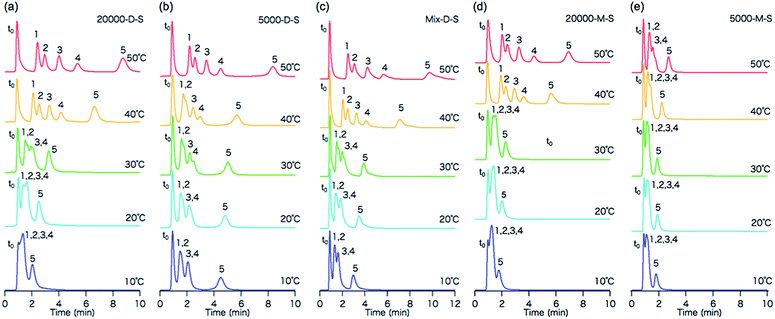 |
| Fig. 4 Temperature-dependent chromatograms of the steroids on (a) 20 000-D-S, (b) 5000-D-S, (c) Mix-D-S, (d) 20 000-M-S, and (e) 5000-M-S HPLC columns. Peaks (t0) uracil, (1) hydrocortisone, (2) prednisolone, (3) dexamethasone, (4) hydrocortisone acetate, and (5) testosterone. | |
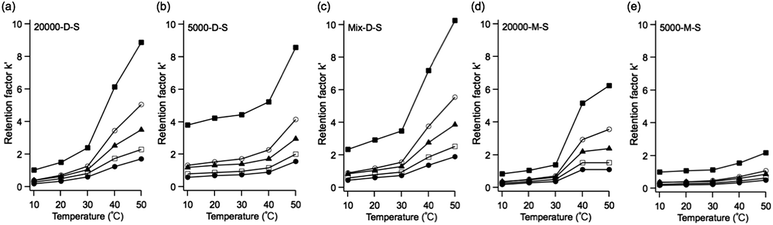 |
| Fig. 5 Temperature-dependent retention factors of five steroids on (a) 20 000-D-S, (b) 5000-D-S, (c) Mix-D-S, (d) 20 000-M-S, and (e) 5000-M-S HPLC columns: (●) hydrocortisone, (□) prednisolone, (▲) dexamethasone, (○) hydrocortisone acetate, and (■) testosterone. | |
Polymer chain length-dependent elution behavior of steroids on dodecyl terminated PNIPAAm temperature-responsive chromatography systems
To investigate the influence of polymer chain length on the temperature-dependent elution behavior of systems using the stationary phases with dodecyl terminal groups, the retention factors of testosterone on 20
000-D-S, 5000-D-S, and Mix-D-S were compared, as shown in Fig. 6a (the retention factors of other steroids are shown in Fig. S2 in the ESI†).
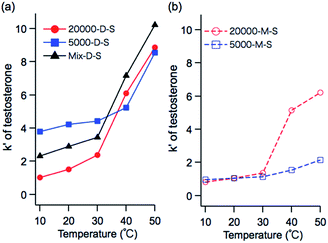 |
| Fig. 6 Temperature-dependent retention factors of testosterone on PNIPAAm grafted silica columns with (a) dodecyl trithiocarbonate terminal groups, and (b) maleimide terminal groups. | |
At 10–30 °C, i.e., below the LCST, the retention factor of 5000-D-S was larger than that of 20
000-D-S. In contrast, at 40 and 50 °C, i.e., above the LCST, the retention factor of 20
000-D-S was larger than that of 5000-D-S. Though the polymer chain of PNIPAAm exhibits hydrophilic characteristics below the LCST, the terminal dodecyl trithiocarbonate moiety exhibits hydrophobic characteristics. In the case of 5000-D-S, the contribution of the terminal hydrophobic group to the polymer chain of PNIPAAm is more significant than in 20
000-D-S; therefore, the dodecyl group contributes more hydrophobicity to 5000-D-S than 20
000-D-S. The polymer chain of PNIPAAm exhibits hydrophobic characteristics above the LCST. The 20
000-D-S with the long polymer chain has the stronger hydrophobic surface, and a larger hydrophobic interaction with steroids than 5000-D-S. In the case of Mix-D-S, the retention factor is the average of the values for 20
000-D-S and 5000-D-S below the LCST, and the retention factor was largest above the LCST. Mix-D-S was fabricated with 20
000-D and 5000-D; therefore, the hydrophobic contribution of the terminal dodecyl trithiocarbonate moiety to the polymer chain on the silica beads leads to the middle value below the LCST.
The larger retention factor of Mix-D-S above the LCST is assumed to be due to the higher density of the PNIPAAm brush than that of 20
000-D-S. Nagasaki et al.40 compared the surface PEG density on a gold surface after only long PEG treatment and after a mixed treatment with short PEG and long PEG. The mixed PEG treatment produced a higher PEG surface density than that with long PEG treatment only. The amounts, and grafted density of PNIPAAm with terminal dodecyl group on the silica beads were determined by CHN elemental analysis for the comparison of 20
000-D-S, 5000-D-S, and Mix-D-S (Table 3). The total grafted PNIPAAm (20
000-D + 5000-D) of Mix-D-S was larger than that of 20
000-D. In addition, the total graft density of Mix-D-S was higher than 20
000-D-S. Therefore, PNIPAAm brush surface of Mix-D-S shows a stronger hydrophobic interaction with steroids.
Table 3 Characterization of PNIPAAm-grafted silica beads by elemental analysis
|
Grafted copolymer (mg m−2) |
Graft density (chains × 10−3 nm−2) |
20 000-D |
5000-D |
Total |
20 000-D |
5000-D |
Total |
20 000-D-S |
0.268 ± 0.002 |
|
0.268 ± 0.002 |
8.20 ± 0.06 |
|
8.20 ± 0.06 |
5000-D-S |
|
0.282 ± 0.003 |
0.282 ± 0.003 |
|
34.0 ± 0.4 |
34.0 ± 0.4 |
Mix-D-S |
0.211 ± 0.001 |
0.111 ± 0.003 |
0.322 ± 0.004 |
6.45 ± 0.05 |
13.4 ± 0.3 |
19.9 ± 0.4 |
As can be seen from the chromatograms, 20
000-D-S cannot separate steroids (except testosterone) below the LCST due to its lower hydrophobic interactions, and the separation of steroids can be achieved only by changing the hydrophobic surface with increasing temperature. In contrast, 5000-D-S separates steroids below the LCST more efficiently, and the change in the degree of separation is only moderate with increasing temperature.
Polymer chain length-dependent elution behavior of steroids on maleimide terminated PNIPAAm temperature-responsive chromatography systems
To investigate the influence of polymer chain length on the temperature-dependent elution behavior of stationary phases with a maleimide terminal group, the retention factors of testosterone on 20
000-M-S, and 5000-M-S were compared, as shown in Fig. 6b (the retention factors of other steroids are shown in Fig. S2 in the ESI†).
While the retention factors with 20
000-M-S drastically increase, those with 5000-M-S only slightly increase with increasing temperature. It has been reported that PNIPAAm chain collapse depends on the molecular weight and grafting density,41 and low molecular weight PNIPAAm does not collapse above the LCST.42 In the case of temperature-responsive chromatography systems, it is expected that the low molecular weight PNIPAAm on the silica beads (5000-M-S) does not completely collapse above the LCST. Therefore, the hydrophobic interaction between 5000-M-S and steroids is weaker than that of 20
000-M-S.
Polymer chain length-dependent elution behavior of steroids on maleimide terminated PNIPAAm temperature-responsive chromatography systems
To investigate the influence of the terminal functional group on the temperature-dependent elution behavior of each polymer chain length, the retention factors of testosterone on dodecyl trithiocarbonate and maleimide terminal group PNIPAAm were compared, as shown in Fig. 7 (the retention factors of other steroids are shown in Fig. S2 in the ESI†). The retention factors decrease with substitution of hydrophobic dodecyl trithiocarbonate with hydrophilic maleimide terminal groups for all chain lengths. As the polymer chain becomes shorter, the retention factor decreases more significantly with the substitution of the terminal group. These results indicate that the contribution of terminal groups increases for shorter chain lengths.
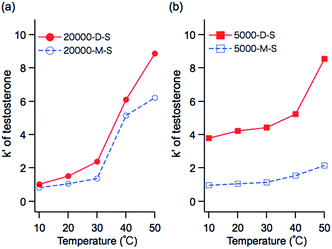 |
| Fig. 7 Temperature-dependent retention factors of testosterone on PNIPAAm-grafted silica columns with a molecular weight of (a) 20 000 g mol−1, and (b) 5000 g mol−1. | |
Conclusions
We have successfully synthesized four types of PNIPAAm with two chain lengths and two types of terminal functional group. These polymers were grafted onto aminopropyl silica, and five types of polymer modified silica beads were prepared. The elution behaviors of steroids on HPLC systems employing these beads as the stationary phase were observed in order to characterize the PNIPAAm modified silica surfaces. The retention factors of the steroids became smaller with the substitution of the terminal hydrophobic dodecyl group with a maleimide group. It was found that the retention factors of steroids were significantly affected by the hydrophobicity of the terminal substituent. The retention factor of 5000-D-S was larger than that of 20
000-D-S below the LCST, and the retention factor of 20
000-D-S was larger than that of 5000-D-S above the LCST. It was found that the effect of the hydrophobic interaction of the polymer chain was larger with a longer polymer chain. In contrast, the effect of the hydrophobic interaction derived from the terminal hydrophobic dodecyl group was larger with a shorter polymer chain. In the case of Mix-D-S, which is assumed to afford a higher density PNIPAAm brush, the retention factor was largest above the LCST. The retention factors with 20
000-M-S drastically increased, and those with 5000-M-S only slightly increased, with increasing temperature. It was found that different temperature-responsive changes in retention factor were obtained by changing molecular weight. These results indicated that the polymer chain length and terminal substituent have a significant effect on the function of the temperature-responsive columns. Furthermore, with the modification of the terminal functional moiety, it is possible to add a further separation mode in addition to the temperature-dependent hydrophobic separation mode.
Acknowledgements
This study was supported in part by a Grant-in-Aid for Scientific Research (B) (Grant No. 25293009) from the Ministry of Education, Culture, Sports, Science and Technology, Japan. We acknowledge Kenichi Nagase (Tokyo Women's Medical University) for helping us with the elemental analysis.
Notes and references
- M. Heskins and J. E. Guillet, J. Macromol. Sci., Chem., 1968, 2, 1441–1455 CrossRef CAS PubMed.
- H. G. Schild, Prog. Polym. Sci., 1992, 17, 163–249 CrossRef CAS.
- M. Chen, M. Dong, R. Havelund, V. R. Regina, R. L. Meyer, F. Besenbacher and P. Kingshott, Chem. Mater., 2010, 22, 4214–4221 CrossRef CAS.
- H. Kanazawa, K. Yamamoto, Y. Matsushima, N. Takai, A. Kikuchi, Y. Sakurai and T. Okano, Anal. Chem., 1996, 68, 100–105 CrossRef CAS PubMed.
- Y. Hiruta, M. Shimamura, M. Matsuura, Y. Maekawa, T. Funatsu, Y. Suzuki, E. Ayano, T. Okano and H. Kanazawa, ACS Macro Lett., 2014, 3, 281–285 CrossRef CAS.
- H. Feil, Y. H. Bae, J. Feijen and S. W. Kim, Macromolecules, 1993, 26, 2496–2500 CrossRef CAS.
- Y. Hiruta, T. Funatsu, M. Matsuura, J. Wang, E. Ayano and H. Kanazawa, Sens. Actuators, B, 2015, 207, 724–731 CrossRef CAS PubMed.
- Y. Hiruta, Y. Nagumo, Y. Suzuki, T. Funatsu, Y. Ishikawa and H. Kanazawa, Colloids Surf., B, 2015, 132, 299–304 CrossRef CAS PubMed.
- D. Wang, T. Liu, J. Yin and S. Liu, Macromolecules, 2011, 44, 2282–2290 CrossRef CAS.
- H. Akiyama and N. Tamaoki, Macromolecules, 2007, 40, 5129–5132 CrossRef CAS.
- H. Kanazawa, Y. Kashiwase, K. Yamamoto, Y. Matsushima, A. Kikuchi, Y. Sakurai and T. Okano, Anal. Chem., 1997, 69, 823–830 CrossRef CAS.
- K. Nagase, M. Kumazaki, H. Kanazawa, J. Kobayashi, A. Kikuci, Y. Akiyama, M. Annaka and T. Okano, ACS Appl. Mater. Interfaces, 2010, 2, 1247–1253 CAS.
- H. Kanazawa, M. Nishikawa, A. Mizutani, C. Sakamoto, Y. Morita-Murase, Y. Nagata, A. Kikuchi and T. Okano, J. Chromatogr., A, 2008, 1191, 157–161 CrossRef CAS PubMed.
- T. Nishio, E. Ayano, Y. Suzuki, H. Kanazawa and T. Okano, J. Chromatogr., A, 2011, 1218, 2079–2084 CrossRef CAS PubMed.
- T. Nishio, R. Kanazashi, A. Nojima, H. Kanazawa and T. Okano, J. Chromatogr., A, 2012, 1228, 148–154 CrossRef CAS PubMed.
- E. Ayano and H. Kanazawa, Anal. Sci., 2014, 30, 167–173 CrossRef CAS.
- K. Sakata, K. Ohkubo, Y. Hiruta, E. Ayano and H. Kanazawa, Kobunshi Ronbunshu, 2014, 71, 293–301 CrossRef CAS.
- H. Willcock and R. K. O'Reilly, Polym. Chem., 2010, 1, 149–157 RSC.
- J. Du, H. Willcock, J. P. Patterson, I. Portman and R. K. O'Reilly, Small, 2011, 7, 2070–2080 CrossRef CAS PubMed.
- J. E. Chung, M. Yokoyama, K. Suzuki, T. Aoyagi, Y. Sakurai and T. Okano, Colloids Surf., B, 1997, 9, 37–48 CrossRef CAS.
- S. Furyk, Y. Zhang, D. Ortiz-Acosta, P. S. Cremer and D. E. Bergbreiter, J. Polym. Sci., Part A: Polym. Chem., 2006, 44, 1492–1501 CrossRef CAS PubMed.
- P. de, S. R. Gondi and B. S. Sumerlin, Biomacromolecules, 2008, 9, 1064–1070 CrossRef CAS PubMed.
- M. Nakayama and T. Okano, Biomacromolecules, 2005, 6, 2320–2327 CrossRef CAS PubMed.
- M. Nakayama and T. Okano, Macromolecules, 2008, 41, 504–507 CrossRef CAS.
- N. Matsuzaka, M. Nakayama, H. Takahashi, M. Yamato, A. Kikuchi and T. Okano, Biomacromolecules, 2013, 14, 3164–3171 CrossRef CAS PubMed.
- W. A. Braunecker and K. Matyjaszewski, Prog. Polym. Sci., 2007, 32, 93–146 CrossRef CAS PubMed.
- K. Matyjaszewski and J. Xia, Chem. Rev., 2001, 101, 2921–2990 CrossRef CAS PubMed.
- G. Moad, E. Rizzardo and S. H. Thang, Aust. J. Chem., 2005, 58, 379–410 CrossRef CAS.
- K. Nagase, J. Kobayashi, A. Kikuchi, Y. Akiyama, H. Kanazawa and T. Okano, Langmuir, 2007, 23, 9409–9415 CrossRef CAS PubMed.
- K. Nagase, J. Kobayashi, A. Kikuchi, Y. Akiyama, H. Kanazawa and T. Okano, ACS Appl. Mater. Interfaces, 2013, 5, 1442–1452 CAS.
- K. Nagase, S. F. Yuk, J. Kobayashi, A. Kikuchi, Y. Akiyama, H. Kanazawa and T. Okano, J. Mater. Chem., 2011, 21, 2590 RSC.
- K. Nagase, J. Kobayashi, A. Kikuchi, Y. Akiyama, H. Kanazawa and T. Okano, Biomacromolecules, 2014, 15, 1204–1215 CrossRef CAS PubMed.
- K. Nagase, J. Kobayashi, A. Kikuchi, Y. Akiyama, H. Kanazawa and T. Okano, Biomacromolecules, 2014, 15, 3846–3858 CrossRef CAS PubMed.
- K. Nagase, J. Kobayashi, A. Kikuchi, Y. Akiyama, H. Kanazawa and T. Okano, ACS Appl. Mater. Interfaces, 2012, 4, 1998–2008 CAS.
- K. Nagase, J. Kobayashi, A. Kikuchi, Y. Akiyama, H. Kanazawa and T. Okano, Langmuir, 2008, 24, 511–517 CrossRef CAS PubMed.
- J. Chiefari, Y. K. Chong, F. Ercole, J. Krstina, J. Jeffery, T. P. T. le, R. T. A. Mayadunne, G. F. Meijs, C. L. Moad, G. Moad, E. Rizzardo and S. H. Thang, Macromolecules, 1998, 31, 5559–5562 CrossRef CAS.
- G. Moad, E. Rizzardo and S. H. Thang, Aust. J. Chem., 2012, 65, 985 CrossRef CAS.
- G. Moad, E. Rizzardo and S. H. Thang, Aust. J. Chem., 2006, 59, 669–692 CrossRef CAS.
- D. J. Phillips and M. I. Gibson, Chem. Commun., 2012, 48, 1054–1056 RSC.
- K. Uchida, H. Otsuka, M. Kaneko, K. Kataoka and Y. Nagasaki, Anal. Chem., 2005, 77, 1075–1080 CrossRef CAS.
- K. N. Plunkett, X. Zhu, J. S. Moore and D. E. Leckband, Langmuir, 2006, 22, 4259–4266 CrossRef CAS PubMed.
- X. Zhu, C. Yan, F. M. Winnik and D. Leckband, Langmuir, 2007, 23, 162–169 CrossRef CAS PubMed.
Footnote |
† Electronic supplementary information (ESI) available: Temperature dependent retention factors on HPLC column, 1H NMR spectra. See DOI: 10.1039/c5ra15906g |
|
This journal is © The Royal Society of Chemistry 2015 |