DOI:
10.1039/C5RA15853B
(Paper)
RSC Adv., 2015,
5, 86927-86933
Performance and mechanisms for removal of perfluorooctanoate (PFOA) from aqueous solution by activated carbon fiber†
Received
7th August 2015
, Accepted 5th October 2015
First published on 7th October 2015
Abstract
The sorption behavior of perfluorooctanoate (PFOA) by activated carbon fiber (ACF) in aqueous solution was investigated. The sorption of PFOA on ACF followed pseudo-second-order kinetics and the sorption equilibrium was reached at approximately 6 h. The sorption of PFOA on ACF fitted the Freundlich model well. The sorption capacity of PFOA by ACF decreased with increasing pH, indicating that electrostatic attraction (pH < 7.4) and repulsion (pH > 7.4) existed in the sorption process. Dissolved organic matter (DOM) inhibited the sorption of PFOA on ACF and no obvious sorption was observed when the concentration of DOM was increased to 500 mg L−1. This was primarily because of the competitive sorption between DOM and PFOA, and the blockage of ACF pores by DOM. The results indicated that electrostatic interactions, hydrogen bonding interactions, and hydrophobic interactions were responsible for the fast sorption, and the formation of hemi-micelles and micelles of PFOA further promoted the sorption of PFOA on ACF.
1. Introduction
Perfluorinated compounds (PFCs) are environmentally refractory organic pollutants that have been widely used in different products, i.e., fire-fighting foams, polymer additives, surfactants and cleaning agents, for nearly sixty years.1–3 Perfluorooctanoate (PFOA, C7F15COO−) is one of the most frequently used PFCs in these products. Due to its toxic effect, bioaccumulation and global distribution, PFOA has been detected in various different environmental matrices including sludge,4 dust,5 water,6,7 wildlife,8 air,9 and humans.10 For example, the concentration of PFOA collected from groundwater at a fire-training area at Wurtsmith Air Force Base (WAFB) was 105 μg L−1.11 For drinking water, however, the US Environmental Protection Agency (USEPA) set 0.4 μg L−1 as the PFOA provisional health advisory limit.12 Industrial wastewater is one of the main sources for PFOA entering into natural waters.13 Previous research has reported that the PFOA concentration in natural waters was at the ng L−1 level.14 Especially, close to the source point of PFCs emissions, the concentration of PFOA was up to almost several hundred mg L−1.15 Therefore, it is imperative to develop effective technologies to remove PFOA from these industrial wastewaters before being discharged into the natural environment.
To date, several treatment technologies including membrane filtration,16 ultrasonic irradiation,17 electrochemical oxidation,18 sorption19 and ultraviolet irradiation20 have been applied to remove PFOA from water. Among these methods, sorption has been demonstrated to be one of effective and versatile methods due to its low cost and highly efficiency.21 Previous studies have demonstrated that PFOA could be effectively removed by boehmite,22 alumina,23 zeolite,24 sludge,25 carbon nanotube,26 granular activated carbon (GAC),27 and powder activated carbon (PAC).21 However, limited data are available on the adsorption of PFOA on activated carbon fiber (ACF).
ACF is made from different natural and synthetic fibrous precursors by carbonization and activation. The raw materials of ACFs are viscose, phenolic resin fiber, polyacrylonitrile fiber or pitch fiber. Compared with PAC and GAC, ACF has many advantages including higher specific surface area (700–2500 m2 g−1), more porous structure, more concentrated pore size distribution and smaller fiber diameter.28 These advantages resulted in many favorable characteristics such as high mass transfer rate and high sorption capacity even at low concentration of adsorbate.29 Especially, this fibrous adsorbent can be prepared in the forms of cloth and felt. The recycle and reusage of ACF is easier than that of conventional activated carbon. Many organic pollutants could be effectively removed by ACF due to its good performance, such as pentachlorophenol,30 p-nitrophenol,31 tetracycline,32 phenol,33 dye,34 phenolic compounds,35 and metal ions.36 According to the sorption principle of ACF, ACF may also exhibit effective adsorption capacity for PFOA. However, the role of solution chemistry on PFOA adsorption mechanisms by ACF has not been fully understood.
The main objective of the present study was to evaluate the removal of PFOA from aqueous solution by using ACF as the adsorbent. The sorption kinetics, sorption isotherms, the effects of initial ACF concentrations, solution pH, and dissolved organic matter (DOM) on sorption of PFOA by ACF were carried out to elucidate the removal performance of PFOA. Furthermore, the underlying mechanisms and the possible interactions between the adsorbent and adsorbate were discussed.
2. Materials and methods
2.1. Materials
Perfluorohexanoic acid (PFHxA, 98%), perfluoheptanoic acid (PFHpA, 98%), PFOA (98%), perfluorononanoic acid (PFNA, 98%), perfluorodecanoic acid (PFDA, 98%) and HPLC-grade acetonitrile (99.9%) were purchased from Sigma-Aldrich Chemical Co., Ltd. (St. Louis, MO, USA). ACF was supplied by Shanghai Union Soldiers Environmental Protection Technology Co., Ltd. (Shanghai, China). Sodium hydroxide (NaOH), disodium hydrogen phosphate (Na2HPO4), sodium dihydrogen phosphate (NaH2PO4), phosphorus acid (H3PO4), and fulvic acid (CAS: 479-66-3, 99%) were obtained from Sinopharm Chemical Reagent Co., Ltd. (Beijing, China). All chemicals and reagents were of analytical grade or higher. Prior to use in the sorption experiments, ACF was first washed repeatedly with deionized water and then washed in 80 °C deionized water for 2 h to remove the impurities. After being dried in an oven at 105 °C for 48 h, ACF was cooled and stored in a desiccator.
2.2. Characterization of ACF
The specific surface area of ACF was measured with a surface area analyzer (Quadrasorb SI, Quantachrome, USA), and N2 was used as the flow gas. The point of zero charge (pHpzc) for ACF was measured according to the reported method.37 Fifty mL of 0.01 M NaCl solution was placed into each conical flask, and the pH was adjusted to a value from 2 to 12 by adding 0.1 M HCl or 0.1 M NaOH stock solutions. Then 0.15 g of ACF was added into each flask, and the solutions were stirred continuously at 25 °C for 48 h. Finally, the pH value of each sample was measured. The point of pHinitial = pHfinal was taken as the pHpzc of ACF.
2.3. Sorption experiments
Sorption experiments were conducted in 250 mL conical flasks, containing 100 mL PFOA solution. ACF was placed into each flask and 2 mM NaH2PO4 (pH 7.0) was added as a pH buffer during the sorption. Then the mixture was immediately stirred at the speed of 120 rpm on an orbital shaker. The pH values were adjusted by 0.1 M HCl or 0.1 M NaOH stock solutions. All of the experiments were carried out in triplicate and the average value was adopted. Blank experiments demonstrated that the total PFOA loss was less than 1% of the initial concentration (Table S1†). In the adsorbent effect experiments, the dosages of ACF ranged from 50 to 2500 mg L−1. In the sorption kinetic experiments, sorption of 100 mg L−1 of PFOA solution at initial pH 7.0 by 800 mg L−1 of ACF was carried out. The samples were taken at 0.08, 0.25, 0.5, 1, 1.5, 2, 4, 6, 8, 10, 12 and 16 h. After the sorption experiments, the final solution pH was determined. The sorption isotherm experiments were conducted at initial PFOA concentrations ranging from 50 to 600 mg L−1 for 6 h. The effect of pH on sorption was conducted with 800 mg L−1 of ACF at initial pH values of 3, 4, 6, 8, 10, and 12. The concentration of fulvic acid varying from 0–500 mg L−1 was selected to investigate the effect of fulvic acid on PFOA sorption by 800 mg L−1 of ACF.
2.4. PFOA determination
The concentrations of PFOA were measured using a high performance liquid chromatography (HPLC, DionexU3000, USA) equipped with an Athena C18-WP HPLC column (4.6 mm × 250 mm, 5 μm). The mixture of acetonitrile and 20 mM Na2HPO4 (50/50, v/v) was used as the mobile phase. The flow rate was set at 1.0 mL min−1. The injection volume was 10 μL and the column temperature was maintained at 30 °C. The correlation coefficients (R2) of standard curves for PFOA were above 0.999 (Fig. S1†). In this study, the detection limit of PFOA was 1.0 mg L−1, the sorption capacity was calculated according to the differences between the initial and equilibrium PFOA concentrations using the following equation: |
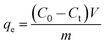 | (1) |
where qe is the sorption amount of PFOA (mg g−1), C0 is the initial PFOA concentration (mg L−1), Ct is the equilibrium concentration of PFOA (mg L−1), V is the solution volume (L), and m is the mass of ACF (g).
3. Results and discussion
3.1. Effect of adsorbent dosage
The effect of adsorbent dosage on the adsorption of PFOA on ACF was investigated. As shown in Fig. 1, the removal ratio of PFOA increased from 19.3 ± 0.8% to 93.4 ± 0.6% as the initial ACF concentration increased from 50 mg L−1 to 1000 mg L−1. The number of active sites for PFOA sorption on ACF surface increased with the increasing concentrations of ACF. This could improve the contact probability between PFOA and ACF, thus resulting in a higher PFOA removal efficiency. However, as the initial ACF concentration increased to 2500 mg L−1, almost all of PFOA was adsorbed on the surface of ACF and the PFOA concentration remaining in the solution was below the detection limits.
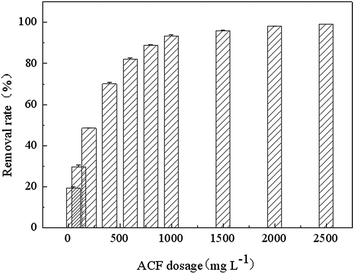 |
| Fig. 1 Effect of ACF dosage on the sorption behavior of PFOA (each point represents the average of three replicates). | |
3.2. Sorption kinetics
Fig. 2 displays the sorption kinetics of PFOA on ACF at pH 7.0. It can be seen that the sorption equilibrium time of PFOA by ACF is approximately 6 h. The sorption rate was fast in the first stage of 2 h, reaching 67.5% of equilibrium sorption capacity of ACF. The rapid sorption equilibrium of microcystin LR38 and uranium39 by ACF was also observed, where only 0.08–0.5 h was required to reach sorption equilibrium. The adsorption of PFOA on GAC and resin AI400 were equilibrated after at least 168 h.21 These results suggested that the size of the adsorbent influenced the sorption rate of PFOA.
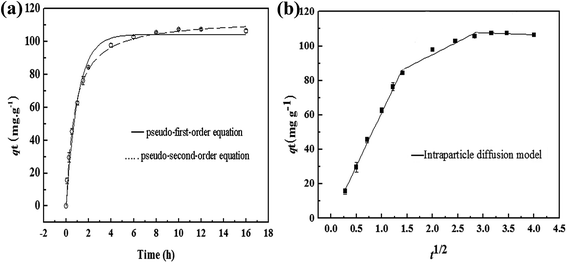 |
| Fig. 2 Sorption kinetics of PFOA on ACF fitted by (a) pseudo-first-order equation (──) and pseudo-second-order equation (……), and (b) intraparticle diffusion model (each point represents the average of three replicates). | |
Three models were applied to investigate the sorption kinetics of PFOA on ACF, including the modified pseudo-first-order, pseudo-second-order, and intraparticle diffusion models. The pseudo-first-order model can be expressed as follows:40
where
t is the sorption time (h),
qe and
qt are the amount of PFOA adsorbed at sorption equilibrium and time
t (mg g
−1), respectively, and
kl is the pseudo-first-order rate constant (h
−1).
The pseudo-second-order model is presented as follows:40
|
 | (3) |
where
k*2 =
k2qe (h
−1), and
k2 is the rate constant of pseudo-second-order model (g mg
−1 h
−1).
The intraparticle diffusion model proposed by Weber and Morris41 is expressed as follows:
where
kwm is the intraparticle diffusion rate constant (mg g
−1 h
−1/2) and
C is the intercept (mg g
−1).
As shown in Fig. 2a and Table S2,† the pseudo-second-order model fitted the data better than the pseudo-first-order model according to the relatively higher correlation coefficient (R2 = 0.998). Previous studies have also demonstrated that the sorption of PFOA onto GAC, PAC, and carbon nanotubes fitted the pseudo second-order kinetics well.42–44 These results indicated that the chemical interactions played a major role in the sorption process.45 PFOA existed in the form of anion at pH 7.0 due to its low pKa (2.5) and the surface charge of ACF was positive (pHzpc = 7.4). Therefore the electrostatic interaction between the positively charged ACF and negatively charged PFOA facilitated the sorption of PFOA on ACF.
Because the pseudo-second-order model could not elucidate the sorption mechanism, the intraparticle diffusion model was adopted to fit the sorption kinetics. The intraparticle diffusion model assumes that the intraparticle diffusion is the sole rate-controlling factor in the sorption process. If the regression of qt versus the square root of time (t1/2) is a straight line which passes through the origin, the sorption process is controlled by intraparticle diffusion only.21,46 As shown in Fig. 2b and Table S3,† the intraparticle diffusion model failed to fit the sorption kinetics of PFOA on ACF because of the negative intercepts. This result demonstrated that intraparticle diffusion was not the only rate-controlling step for PFOA sorption on ACF. Yu et al.21 compared the sorption of PFOA on PAC, GAC and resin AI400. The results indicated that the sorption kinetics of PFOA on GAC and resin AI400 followed the intraparticle diffusion-controlled adsorption in the initial phase. In contrast, this model could not fit the sorption kinetics of PFOA by PAC. This was primarily because PAC has a smaller size than GAC and resin AI400. Because of the small size of ACF (Table 1), PFOA can easily diffuse into the inward pores, and thus the external and intraparticle diffusion may be equal in this sorption process or even the external diffusion becomes the rate-limited step.
Table 1 Characteristics of ACF used in this study
BET surface area (m2 g−1) |
Pore diameter (Å) |
Micropore volume (cm3 g−1) |
Micropore area (m2 g−1) |
Total pore volume (cm3 g−1) |
pHzpc |
1226.16 |
5.63 |
0.392 |
991.85 |
0.563 |
7.4 |
3.3. Sorption isotherm
To further understand the interactions between adsorbent and adsorbate, the Langmuir and Freundlich equations were applied to describe the sorption data.
The Langmuir equation assumes that the sorption occurs in the monolayer of adsorbent and the equation is expressed as follows:
|
 | (5) |
where
qe is the equilibrium sorption amount (mg g
−1),
Ce is the equilibrium concentration of PFOA in solution (mg L
−1),
kl represents the sorption affinity coefficient (mg L
−1), and
Q0 is the maximum sorption capacity (mg g
−1).
The Freundlich model is an empirical equation assuming heterogeneous adsorptive energies on the adsorbent surface. The isotherm is defined by:
where
KF is the Freundlich constant related to sorption capacity (mg
1−1/n L
1/n g
−1), and
n is a constant depicting the sorption intensity.
As shown in Fig. 3 and Table S4,† the sorption isotherms of PFOA on ACF could be fitted better with Freundlich equation than Langmuir equation. The results demonstrated that the energy distribution for the sorption “sites” was an exponential type, implying that there was multilayer sorption in the sorption of PFOA on ACF.47 According to the fitting results of Freundlich model, n−1 value was 0.43 as expected for a non-linear fitting. Nonlinearity could occur due to the heterogeneity of sorption site and the interactions among sorbates such as electrostatic repulsion.21,48
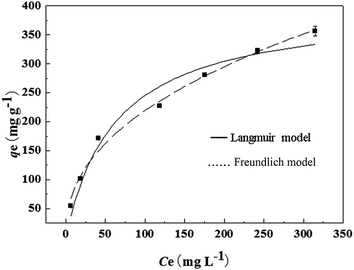 |
| Fig. 3 Sorption isotherms of PFOA on ACF fitted by Langmuir and Freundlich equations. | |
3.4. Effect of pH
Solution pH plays an important role in the sorption of PFOA by ACF because pH not only influences the surface charge of ACF, but also affects the speciation of PFOA in aqueous solution. The effect of pH on the sorption of PFOA by ACF is illustrated in Fig. 4a. It can be seen that the sorption capacities of PFOA on ACF decreased from 112.3 mg L−1 to 103.5 mg L−1 as the pH values increased from 7.4 to 12.0. Meantime, the sorption capacity of PFOA on ACF decreased by 8.1% as the pH value increased from 3.0 to 7.4.
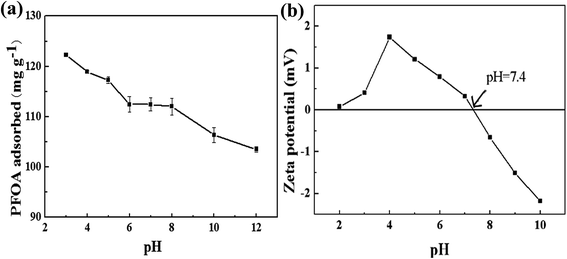 |
| Fig. 4 Effect of pH on the sorption behavior of PFOA on ACF (a), and the zeta potential of ACF (b). | |
As the pKa value of PFOA (2.5) was lower than the pH values (3.0–12.0) of PFOA solution in this study, PFOA mainly existed in anionic form within the pH range. Fig. 4b presents the zeta potentials of ACF at different pH values. The pHpzc of ACF is approximately 7.4. When solution pH was higher than 7.4, the surface charge of ACF was negative. Therefore the electrostatic repulsion played an important role in this sorption process. Furthermore, as the ACF surface had more negative surface charges with the increasing solution pH, the increasing electrostatic repulsion resulted in the decreasing sorption capacity. However, the surface charge of ACF was positive at solution pH below 7.4, implying that electrostatic interaction between PFOA and ACF was attractive.
The removal ratio of PFOA decreased by 15.6% when the solution pH increased from 3.0 to 12.0, suggesting that other interactions existed in this process, such as hydrophobic interaction and hydrogen bonding interaction. These interactions may be weaken electrostatic repulsion between PFOA and ACF, leading to only 15.6% decrease in this sorption process. In contrast, it was reported that pH had a significant effect on the sorption of PFOA on alumina. The adsorption capacity of PFOA decreased with the increase of the solution pH, and no measurable amount of PFOA was adsorbed onto alumina at pH 7.5.23 The results suggested that the sorption of PFOA on alumina was mainly attributed to the electrostatic interaction since the electrostatic interaction was the only pH-sensitive force. The results indicated that ACF had a high sorption performance within a wide pH range.
3.5. Effect of DOM
DOM is ubiquitous in natural waters, and it can interact with organic contaminants by a variety of binding and adsorption interactions.49 Many researches have demonstrated that these interactions could affect the migration and transformation of organic pollutants.50,51 In this study, fulvic acid was selected as the model DOM to investigate the effect of DOM on the sorption of PFOA by ACF.
As shown in Fig. 5, the sorption capacity of PFOA on ACF was obviously decreased with the increasing fulvic acid concentration from 0 to 500 mg L−1. Blank experiments (the concentration of fulvic acid was 0 mg L−1) demonstrated that the amount of PFOA adsorbed on ACF was 103.91 mg g−1. As the concentration of fulvic acid increased to 100 mg L−1, the sorption capacity of PFOA decreased by 75.4%. Especially, when the concentration of fulvic acid was 500 mg L−1, no obvious sorption reaction was observed in the experiments. The above results demonstrated that competitive sorption existed in this sorption process.
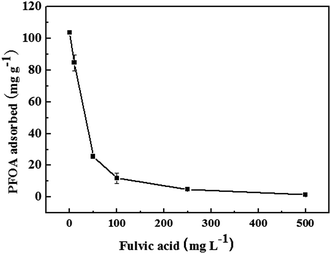 |
| Fig. 5 Effect of fulvic acid on the sorption behavior of PFOA (each point represents the average of three replicates). | |
It is known that DOM inhibited the sorption of trace organic compounds through two major mechanisms: direct site competition and pore blockage.52 DOM would compete for sorption site of activated carbon with the target organic compounds. The molecular weight (MW) of DOM is a critical factor that affects the competition between DOM and trace organic compounds.51 Different MW of DOM compounds have been demonstrated to have diverse effects on the sorption of trace organic compounds on activated carbon. It has been reported that the effluent organic matter (EfOM) fraction with MW lower than 1 kDa had the greatest effect on adsorption capacity for PFCs, while adsorption was almost unaffected by the EfOM fraction with MW greater than 30 kDa.51 Similar trend has also been reported by Newcombe et al.,53 who reported that larger MW fraction of DOM compounds (>30
000) had much less effect on 2-methylisoborneol (MIB) adsorption capacity while lower MW compounds (<500) exhibited higher uptake and exerted a greater competitive effect on the adsorption of MIB.53 In the present study, fulvic acid belongs to the low MW fraction of DOM. As a consequence, the sorption capacity of PFOA could be significantly affected by fulvic acid and decreased with the increasing concentration of fulvic acid. Furthermore, previous studies have demonstrated that pore blockage was the major mechanism for inhibited sorption of target organic compounds by DOM.54,55 Kilduff et al.56 carried out the experiments to verify theoretical predictions of competitive effects between trichloroethylene and activated carbon in the presence of humic acid. The results indicated that the changes of site-energy heterogeneity were small at high loadings of humic acid, suggesting that the possibility of pore blockage or pore filling mechanism existed. The preloaded DOM could block some adsorption sites of sorbent, thus reducing the sorption capacity of trace organic compounds, especially when the DOM loading was high. They may also narrow the existing transport pores, resulting in the decreased sorption rate for trace organic compounds. The phenomena have also been verified by other researchers.57,58 Therefore, the adsorbed fulvic acid could block the micropores and surface active sites of ACF and subsequently decreased their sorption capacity for PFOA.
3.6. Sorption mechanisms
As discussed above, the electrostatic interactions existed between ACF and PFOA, which was justified by the decreased sorption capacity of PFOA on ACF as the pH values increased from 3.0 to 12.0. However, the sorption capacity of PFOA on ACF decreased by only 15.6%, indicating that the hydrophobic interaction and hydrogen bonding interaction between ACF and PFOA were much stronger than the electrostatic repulsion.
Hydrogen bonding interaction could be formed between fluorine atoms of PFOA and hydrogen atoms of ACF because –OH group of ACF could act as hydrogen-bonding donors (Fig. S2†). The protonated groups of ACF can also capture water molecules via hydrogen bonds,59,60 which leads to the competitive sorption of water with PFOA. Therefore the hydrogen bonding interaction played an insignificant role in the adsorption process of PFOA. A schematic diagram of the sorption of PFOA onto ACF is proposed in Fig. 6. Due to the hydrophobicity of ACF and the hydrophobic perfluorinated chain of PFOA, the hydrophobic interaction was involved in the sorption of PFOA on ACF.61 In order to explore this possibility, the competitive sorption of perfluorinated carboxylic acids (PFCAs) on ACF was conducted in the mixed solution containing PFHxA, PFHpA, PFOA, PFNA, and PFDA. As shown in Fig. S3,† ACF had the highest sorption capacity for PFDA and the lowest sorption capacity for PFHxA (approximately zero) in the competitive sorption process. The sorption capacities of PFCAs on ACF increased with the increasing carbon chain length, and the adsorbed amounts of PFCAs on ACF followed the order of PFDA > PFNA > PFOA > PFHpA > PFHxA because PFCAs with longer C–F chain length are more hydrophobic. These results indicated that hydrophobic interaction played an important role on the sorption of PFCAs on ACF.
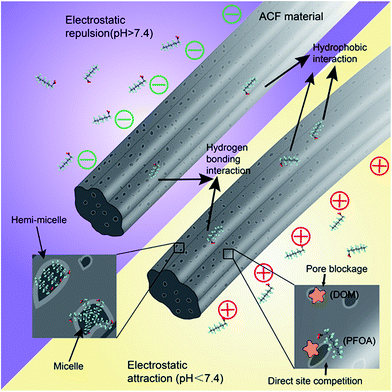 |
| Fig. 6 The schematic diagram of PFOA sorption process on ACF. | |
Although the critical micelle concentration (CMC) value of PFOA is 15
696 mg L−1, the hemi-micelles and micelles are likely to form in the range of 0.01–0.001 of the CMC.62 The accumulation of hemi-micelles and micelles enhanced the adsorbed concentration of PFOA onto ACF.63 The hydrophobic interaction was the main mechanism for the sorption of PFOA on ACF. The electrostatic repulsion and attraction existed between PFOA and ACF when the pH values were greater than and less than 7.4, respectively. Hydrogen bonding interaction also involved in the PFOA sorption by ACF. Moreover, the hemi-micelles and micelles of PFOA formed in the adsorbent pores. Direct site competition and pore blockage were exited between PFOA and ACF in the presence of DOM.
4. Conclusions
The sorption kinetics results showed that the adsorption equilibrium of PFOA on ACF could be reached in 6 h. Adsorbent size governed the sorption rate of PFOA on ACF in aqueous solution. With the increasing pH value from 3.0 to 12.0, the sorption capacities of PFOA on ACF decreased by 15.6%. PFOA sorption was completely inhibited by DOM at 500 mg L−1. Both hydrogen bonding interaction and electrostatic interaction were involved in the sorption of PFOA. The hydrophobic interaction played a dominant role in this sorption process. Furthermore, PFOA might form hemi-micelles and micelles in the adsorbent pores, which significantly enhanced the sorption capacity.
Acknowledgements
This study was financially supported by the Fund for Innovative Research Group of the National Natural Science Foundation of China (No. 51421065), the National Natural Science Foundation of China (No. 51378065), and the Fundamental Research Funds for the Central Universities (No. 2012LZD03).
References
- J. P. Giesy and K. Kannan, Environ. Sci. Technol., 2001, 35, 1339–1342 CrossRef CAS.
- M. M. Schultz, D. F. Barofsky and J. A. Field, Environ. Sci. Technol., 2004, 38, 1828–1835 CrossRef CAS.
- E. Kissa, Fluorinated Surfactants and Repellants, Marcel Dekker, New York, 2nd edn, 2001 Search PubMed.
- O. Sindiku, F. Orata, R. Weber and O. Osibanjo, Chemosphere, 2013, 92, 329–335 CrossRef CAS PubMed.
- M. Nadal and J. L. Domingo, Curr. Org. Chem., 2014, 18, 2200–2208 CrossRef CAS.
- Z. Lu, L. Song, Z. Zhao, Y. Ma, J. Wang, H. Yang, H. Ma, M. Cai, G. Codling, R. Ebinghaus, Z. Xie and J. P. Giesy, Chemosphere, 2015, 119, 820–827 CrossRef CAS PubMed.
- C. Xu, J. J. Zhu, Y. Li, Y. J. Yu and G. L. Duan, RSC Adv., 2015, 5, 13192–13199 RSC.
- A. Rotander, A. Karrman, B. van Bavel, A. Polder, F. Riget, G. A. Auounsson, G. Vikingsson, G. W. Gabrielsen, D. Bloch and M. Dam, Chemosphere, 2012, 86, 278–285 CrossRef CAS PubMed.
- E. Goosey and S. Harrad, Environ. Int., 2012, 45, 86–90 CrossRef CAS PubMed.
- H. Nilsson, A. Karrman, A. Rotander, B. van Bavel, G. Lindstrom and H. Westberg, Environ. Int., 2013, 51, 8–12 CrossRef CAS PubMed.
- C. A. Moody, G. N. Hebert, S. H. Strauss and J. A. Field, J. Environ. Monit., 2003, 5, 341–345 RSC.
- USEPA, Provisional Health Advisories for Perfluorooctanoic Acid (PFOA) and Perfluorooctane Sulfonate (PFOS), 2009 Search PubMed.
- K. Prevedouros, I. T. Cousins, R. C. Buck and S. H. Korzeniowski, Environ. Sci. Technol., 2006, 40, 32–44 CrossRef CAS.
- J. B. N. Mudumbi, S. K. O. Ntwampe, F. M. Muganza and J. O. Okonkwo, Water Sci. Technol., 2014, 69, 185–194 CrossRef CAS PubMed.
- N. Saito, K. Harada, K. Inoue, K. Sasaki, T. Yoshinaga and A. Koizumi, J. Occup. Health, 2004, 46, 49–59 CrossRef CAS.
- T. D. Appleman, E. R. V. Dickenson, C. Bellona and C. P. Higgins, J. Hazard. Mater., 2013, 260, 740–746 CrossRef CAS PubMed.
- H. Zhao, J. Gao, G. Zhao, J. Fan, Y. Wang and Y. Wang, Appl. Catal., B, 2013, 136, 278–286 CrossRef PubMed.
- Q. Zhuo, S. Deng, B. Yang, J. Huang and G. Yu, Environ. Sci. Technol., 2011, 45, 2973–2979 CrossRef CAS PubMed.
- L. Zhao, J. Bian, Y. Zhang, L. Zhu and Z. Liu, Chemosphere, 2014, 114, 51–58 CrossRef CAS PubMed.
- T. Lan-Anh Phan, D. Huu-Tuan, Y. C. Lee and S. L. Lo, Chem. Eng. J., 2013, 221, 258–263 CrossRef PubMed.
- Q. Yu, R. Zhang, S. Deng, J. Huang and G. Yu, Water Res., 2009, 43, 1150–1158 CrossRef CAS PubMed.
- F. Wang, C. Liu and K. Shih, Chemosphere, 2012, 89, 1009–1014 CrossRef CAS PubMed.
- F. Wang and K. Shih, Water Res., 2011, 45, 2925–2930 CrossRef CAS PubMed.
- V. Ochoa-Herrera and R. Sierra-Alvarez, Chemosphere, 2008, 72, 1588–1593 CrossRef CAS PubMed.
- O. S. Arvaniti, H. R. Andersen, N. S. Thomaidis and A. S. Stasinakis, Chemosphere, 2014, 111, 405–411 CrossRef CAS PubMed.
- X. Li, H. Zhao, X. Quan, S. Chen, Y. Zhang and H. Yu, J. Hazard. Mater., 2011, 186, 407–415 CrossRef CAS PubMed.
- Y. Yao, K. Volchek, C. E. Brown, A. Robinson and T. Obal, Water Sci. Technol., 2014, 70, 1983–1991 CrossRef CAS PubMed.
- S. H. Byeon, S. M. Oh, W. S. Kim and C. H. Lee, Ind. Health, 1997, 35, 404–414 CrossRef CAS.
- I. Martin-Gullon and R. Font, Water Res., 2001, 35, 516–520 CrossRef CAS.
- P. E. Diaz-Flores, R. Leyva-Ramos, R. M. Guerrero-Coronado and J. Mendoza-Barron, Ind. Eng. Chem. Res., 2006, 45, 330–336 CrossRef CAS.
- D. Tang, Z. Zheng, K. Lin, J. Luan and J. Zhang, J. Hazard. Mater., 2007, 143, 49–56 CrossRef CAS PubMed.
- L. Huang, C. Shi, B. Zhang, S. Niu and B. Gao, Sep. Sci. Technol., 2013, 48, 1356–1363 CrossRef CAS PubMed.
- M. Li, Y. Luo, T. Xie, M. Liang and S. Wang, Adv. Mater., ed. J. L. Bu, Z. Y. Jiang and S. Jiao, 2012, vol. 415–417, Pts 1-3, pp. 1599–1602 Search PubMed.
- I. A. W. Tan, B. H. Hameed and A. L. Ahmad, Chem. Eng. J., 2007, 127, 111–119 CrossRef CAS PubMed.
- E. Ayranci and O. Duman, J. Hazard. Mater., 2005, 124, 125–132 CrossRef CAS PubMed.
- I. Lee, Environmental Engineering Research, 2014, 19, 157–163 CrossRef.
- Y. Qiu and F. Ling, Chemosphere, 2006, 64, 963–971 CrossRef CAS PubMed.
- D. Pyo and D. Moon, Bull. Korean Chem. Soc., 2005, 26, 2089–2092 CrossRef CAS.
- S. Chen, J. Hong, H. Yang and J. Yang, J. Environ. Radioact., 2013, 126, 253–258 CrossRef CAS PubMed.
- B. Pan, K. Sun and B. Xing, J. Soils Sediments, 2010, 10, 845–854 CrossRef CAS.
- W. J. Weber and J. C. Morris, J. Sanit. Eng. Div., Am. Soc. Civ. Eng., 1963, 89, 31–60 Search PubMed.
- X. Chen, X. Xia, X. Wang, J. Qiao and H. Chen, Chemosphere, 2011, 83, 1313–1319 CrossRef CAS PubMed.
- S. T. M. L. D. Senevirathna, S. Tanaka, S. Fujii, C. Kunacheva, H. Harada, B. R. Shivakoti and R. Okamoto, Chemosphere, 2010, 80, 647–651 CrossRef CAS PubMed.
- Y. Qu, C. Zhang, F. Li, X. Bo, G. Liu and Q. Zhou, J. Hazard. Mater., 2009, 169, 146–152 CrossRef CAS PubMed.
- Y. S. Ho and G. McKay, Process Biochem., 1999, 34, 451–465 CrossRef CAS.
- Z. Cheng, X. Liu, M. Han and W. Ma, J. Hazard. Mater., 2010, 182, 408–415 CrossRef CAS PubMed.
- D. O. Cooney, Adsorption design for wastewater treatment, Lewis Publishers, USA, 1999 Search PubMed.
- C. W. Cheung, J. F. Porter and G. McKay, Water Res., 2001, 35, 605–612 CrossRef CAS.
- N. Senesi and Y. Chen, Toxic Organic Chemicals in Porous Media Ecological Studies, 1989, vol. 73, pp. 37–90 Search PubMed.
- J. Akkanen, A. Tuikka and J. V. K. Kukkonen, Ecotoxicol. Environ. Saf., 2012, 78, 91–98 CrossRef CAS PubMed.
- J. Yu, L. Lv, P. Lan, S. Zhang, B. Pan and W. Zhang, J. Hazard. Mater., 2012, 225, 99–106 CrossRef PubMed.
- Q. L. Li, V. L. Snoeyink, B. J. Mariaas and C. Campos, Water Res., 2003, 37, 773–784 CrossRef CAS.
- G. Newcombe, M. Drikas and R. Hayes, Water Res., 1997, 31, 1065–1073 CrossRef CAS.
- J. E. Kilduff and A. Wigton, Environ. Sci. Technol., 1999, 33, 250–256 CrossRef CAS.
- K. Ebie, F. S. Li, Y. Azuma, A. Yuasa and T. Hagishita, Water Res., 2001, 35, 167–179 CrossRef CAS.
- J. E. Kilduff, T. Karanfil and W. J. Weber, J. Colloid Interface Sci., 1998, 205, 280–289 CrossRef CAS PubMed.
- M. C. Carter and W. J. Weber, Environ. Sci. Technol., 1994, 28, 614–623 CrossRef CAS PubMed.
- G. Newcombe, J. Morrison, C. Hepplewhite and D. R. U. Knappe, Carbon, 2002, 40, 2147–2156 CrossRef CAS.
- F. Ahnert, H. A. Arafat and N. G. Pinto, Adsorption, 2003, 9, 311–319 CrossRef CAS.
- K. Yang and B. Xing, Chem. Rev., 2010, 110, 5989–6008 CrossRef CAS PubMed.
- C. P. Higgins and R. G. Luthy, Environ. Sci. Technol., 2006, 40, 7251–7256 CrossRef CAS.
- R. L. Johnson, A. J. Anschutz, J. M. Smolen, M. F. Simcik and R. L. Penn, J. Chem. Eng. Data, 2007, 52, 1165–1170 CrossRef CAS.
- Q. Zhang, S. Deng, G. Yu and J. Huang, Bioresour. Technol., 2011, 102, 2265–2271 CrossRef CAS PubMed.
Footnote |
† Electronic supplementary information (ESI) available. See DOI: 10.1039/c5ra15853b |
|
This journal is © The Royal Society of Chemistry 2015 |
Click here to see how this site uses Cookies. View our privacy policy here.