DOI:
10.1039/C5RA15373E
(Paper)
RSC Adv., 2015,
5, 79519-79524
A simple and reversible fluorescent probe based on NBD for rapid detection of hypochlorite and its application for bioimaging†
Received
2nd August 2015
, Accepted 10th September 2015
First published on 14th September 2015
Abstract
A simple and reversible fluorescent probe bearing 7-nitrobenz-2-oxa-1,3-diazole and a selenomorpholine fragment was designed and synthesized. The probe showed highly selective, sensitive and fast (<10 s) recognition to hypochlorite in aqueous solutions. The relative results demonstrated that the linear response range of the probe was between 5.0 × 10−8 M and 1.2 × 10−4 M, with a low detection limit of 3.3 nM (S/N = 3). The probe was capable of monitoring hypochlorite reversibly in the presence of glutathione. In addition, the biological applications in living cells have been described.
1 Introduction
Hypochlorite (ClO−) is one of the most significant reactive oxygen species (ROS) in biological systems and plays a critical role in many biological processes.1 It is produced from the reaction of H2O2 with chloride ions catalyzed by a heme enzyme myeloperoxidase (MPO) in living systems.2 However, a high concentration of hypochlorite can cause tissue damage and a variety of pathological diseases including neuron degeneration,3 inflammatory disease,4 atherosclerosis,5 cystic fibrosis,6 arthritis,7 cardiovascular disease,8 cancers,9 and liver cirrhosis.10 When oxidative damage occurs in living cells, the antioxidative and repair mechanisms of living organisms start to protect essential components of cells against the hypochlorite harm at once.11 Therefore, it is worth developing a highly sensitive, good selective and fast technique to monitor the redox changes between hypochlorite and antioxidants in living organisms.
Fluorescence sensing are efficient ways for detecting species both in solution and living organism due to their excellent selectivity, high sensitivity, ability for real-time capability and fast response times. So far, fluorescence sensors for specific detection of hypochlorite have been constructed based on the strong oxidation properties of hypochlorite. These probes were generally designed on the basis of the conjugation of a fluorophore with a hypochlorite reactive group, such as p-methoxyphenol, p-alkoxyaniline, dibenzoylhydrazine, hydroxamic acid, thiol, and oxim derivatives.12 These oxidation-reaction based probes were very useful for sensing for hypochlorite, however, most of them have not realized to detect hypochlorite based on the redox cycle. The complex redox biology of the cell governs many essential biological processes and has broad implications in human health and diseases.13 Thus, it is eagerly desirable to design and develop reversible fluorescent probes.
Selenium (Se) is a compound with various oxidations and it is applicable to the design of reversible ROS probes via the oxidation–reduction cycles.14 In the past few years, Se containing fluorophores have been used for designing reversible fluorescent probes for detecting hypochlorite.15 For example, Liu et al. reported a BODIPY-based fluorescent probe which can reversible recognize hypochlorite.15b Lou et al. developed a reversible fluorescent probe for hypochlorite acid based on 1,8-naphthalimide.15d The probes could respond reversibly for the redox changes mediated by hypochlorite acid and thiols. The reversibly fluorescent probes are suitable for visualizing states of redox stress and continuous monitoring of real-time hypochlorite. However, some of them still encounter some problems, such as low sensitivity, complicated synthetic procedures and long response time. These limitations seriously retarded the application of the probe in real biological. In this regard, it is worth designing and developing new reversible fluorescent probes being able to overcome these limitations in detecting hypochlorite.
The present study about fluorescent probes for detection of hypochlorite was mainly focused on the selection of dyes and recognition fragment. Heptamethine cyanine dye, 1,8-naphthalimide dye, BODIPY dye and other dyes have been widely utilized to explore chemosensors for hypochlorite.16,17 However, to the best of our knowledge, there are few studies and applications of visual and fluorometric sensor based on NBD for highly sensitive and reversible detection of hypochlorite up to now.
7-Nitrobenz-2-oxa-1,3-diazole (NBD) derivatives have been extensively used in fluorescent labeling reagents because of their good spectral properties, excellent biocompatibility, cellular membrane-penetrating capacity, commercial availability and easy functionalization.18 Currently, based on the photoinduced electron transfer (PET) mechanism, NBD derivatives have been used as fluorescent chemosensors for detecting heavy metal ions.19 According to the PET mechanism, we begin to consider a fluorometric sensing integrated with NBD as signaling unit and selenomorpholin as a recognition group for detection of hypochlorite. We envisage when NBD is conjugated with selenide, the fluorescence of the probe is weak. NBD-selenide is oxidized to selenoxide by hypochlorite, the fluorescence of NBD-selenide will increase because of the electron-withdrawing effect of NBD-selenoxide. Once NBD-selenoxide is reduced to NBD-selenide by antioxidants, the fluorescence of NBD-selenide recovers its original weak fluorescence again. In this way, the NBD-selenide is expected to acquire positive results for reversible detection of hypochlorite.
With these considerations in mind, we decided to incorporate the selenomorpholin moiety into the NBD core via a simple substitution reaction, obtaining a novel and reversible probe for visualizing the redox changes mediated by hypochlorite and glutathione (GSH). As shown in Fig. 1, compound 1 contains a NBD dye and selenomorpholin moiety for hypochlorite. As expected, compound 1 itself is weak fluorescent, whereas it showed fluorescence intensity change response between hypochlorite and GSH in aqueous media. Moreover, compound 1 can serve as a naked-eye indicator for hypochlorite by color change. In addition, compound 1 could be successfully applied for hypochlorite detection in living cells. Notably, this novel and simple NBD-selenide based visual and turn on fluorescent probe for highly selective, sensitive and fast (<10 s) detection of hypochlorite with a low detection limit (3.3 nM) will fit the preference of further application.
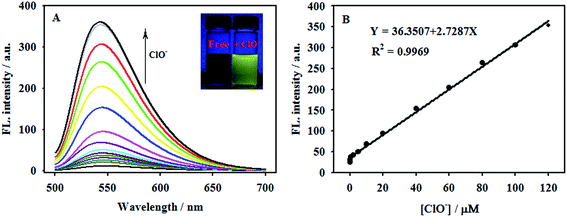 |
| Fig. 1 (A) Fluorescence spectra of compound 1 (10 μM) in acetonitrile–water solution (1 : 1 v/v, 50 mM PBS buffer solution at pH 7.4) at various concentrations of ClO− (0–130 μM); (B) the right figure displays the fluorescent intensity vs. the ClO− concentration. | |
2 Experimental
2.1 Reagents and chemicals
4-Chloro-7-nitro-benzoxadiazole (NBD-Cl) was purchased from Sigma-Aldrich Company. All other chemicals used in this work were of analytical grade, purchased from Sinopharm chemical reagent company and used without further purification. The synthesis of selenomorpholine was similar to the procedures reported by Hu Liming et al.20 The thin-layer chromatography (TLC) was carried out on silica gel plates (60F-254) using UV-light to monitor the reaction, and silica gel (200–300 mesh) was used for column chromatography. Milli-Q ultrapure water (Millipore, ≥18 M cm) was used throughout. Various ROS and RNS including ˙OH, NO3−, NO2−, ONOO−, 1O2, O22−, NO and t-BuOOH were prepared according to published methods.21
2.2 Instrumentation
Fluorescence spectra were carried out using a Hitachi F-7000 spectrophotometer. UV-vis spectra were measured on a Shimadzu UV2450 spectrophotometer. Fluorescence imaging of Hela cells was carried out using fluorescence microscopy. 1H NMR and 13C NMR spectra were recorded using a Bruker AVB-500 MHz NMR spectrometer (Bruker biospin, Switzerland). Mass spectra were obtained using a Bruker Daltonics BioTOF-Q mass spectrometer in the positive mode. The pH measurements were performed with a Sartorius basic pH-meter PB-10.
2.3 Synthesis of compound 1
As illustrated in Scheme 1, compound 1 was readily prepared in one step method. Briefly, to a solution of 4-chloro-7-nitro-benzoxadiazole (NBD-Cl) (0.2 g, 1 mmol) in 5 mL CH2Cl2, selenomorpholine (0.15 g, 1 mmol) and Et3N (0.12 g, 1.2 mmol) were added. The mixture was stirred at room temperature for 4 h under N2 atmosphere. Then, a rude mixture was concentrated under reduced pressure. The residue was purified by silica gel column chromatography (petroleum ether/ethyl acetate, 4
:
1, v/v) to give compound 1 in 85% yield (0.27 g). 1H NMR (CDCl3, 500 MHz) δ (ppm): 8.46 (d, J = 9.0 Hz, 1H), 6.28 (d, J = 9.0 Hz, 1H), 4.57 (t, J = 5.0 Hz, 4H), 2.93 (t, J = 5.0 Hz, 4H); 13C NMR (CDCl3, 125 MHz) δ (ppm): 145.0, 144.8, 145.8, 144.0, 135.1, 123.6, 102.6, 53.3, 16.8; ESI-MS m/z [M + Na]+ calc. 336.1612, obs. 336.9810.
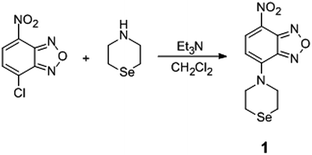 |
| Scheme 1 Synthetic route to compound 1. | |
2.4 Measurement procedure
A stock solution of compound 1 was prepared by dissolving compound 1 in acetonitrile. Fluorescence and absorption spectra were recorded in acetonitrile–water solution (1
:
1 v/v, 50 mM PBS buffer solution at pH 7.4). The fluorescence intensity was recorded with a fluorescence spectrometer (λex = 468 nm, λem = 544 nm, slit: 10 nm/10 nm).
2.5 Cell culture
The Hela cells were incubated on a 96-well plate and allowed to adhere for 24 h at 37 °C, and then washed three times with PBS and incubated at 37 °C in the presence of compound 1 (10 μM, 1
:
99 DMSO-PBS, v/v, pH 7.4) for 30 minute. Then the cells were washed three times with PBS buffer and incubated at 37 °C in the presence of NaClO for 10 minute. The fluorescence images were recorded using an inverted fluorescence microscope (NIKON Eclipse Ti–S, Japan) with a 20× objective lens.
2.6 Determination of fluorescence quantum yield
The fluorescence quantum yield was determined using the solutions of fluorescein (ΦF = 0.90 in 0.1 N NaOH22) as reference. The quantum yield was calculated using the following equation:
ΦF(s) = ΦF(r) (FsAr/FrAs) (ns/nr)2 |
where ΦF is the fluorescence quantum yield, F is the area under the corrected emission curve, A is the absorbance at the excitation wavelength and n is the refractive index of the solvents used. Subscripts refer to the reference (r) or sample (s) compound.
3 Results and discussion
3.1 Spectra properties of compound 1
The fluorescence properties of the compound 1 were studied in CH3CN–H2O solution (1
:
1 v/v, 50 mM PBS buffer solution at pH 7.4). The compound 1 itself displays weak fluorescence (Φ = 0.002), which suggested the PET process from Se atom to NBD fluorophore occurred. Upon addition of ClO−, the fluorescence intensity of the compound 1 at 544 nm increases steadily (Fig. 1a). After 120 μM of ClO− was added, the fluorescence intensity enhanced up to 32-fold, and the final quantum yield was 0.048. The results implied that the PET process in compound 1 was inhibited upon the addition of ClO−, in good agreement with our design. Moreover, the fluorescence intensity of compound 1 at 544 nm showed a good linear response to ClO− in the range of 5 × 10−8 M to 1.20 × 10−4 M (Fig. 1B). The detection limit for ClO− is estimated to be 3.3 nM (S/N = 3). The low detection limit shown that compound 1 is highly sensitive to ClO−.
To further get insight into the reaction of ClO− with compound 1, the UV spectrum of compound 1 in the absence and in the presence of ClO− were investigated. As depicted in Fig. 2, compound 1 itself displayed absorption centered at 344 nm and 483 nm. However, upon addition of 12 equiv. of ClO− to a solution of compound 1, the absorbance bands of compound 1 were recorded with a blue-shift to 294 nm and 468 nm respectively. Blue-shift in absorption spectra further demonstrated that compound 1 can react with ClO−. Concurrently, an obvious color change from orange to yellow was observed (Fig. 2, inset). This phenomenon indicates that compound 1 can be employed conveniently for ClO− detection by simple visual inspection.
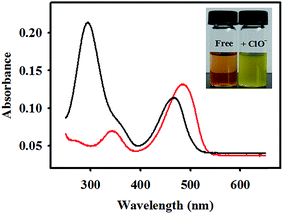 |
| Fig. 2 UV-vis spectra of 30 μM compound 1 in the absence of ClO− (red line) and in the presence of ClO− (black line). Inset: the photographic images of compound 1 in the presence of and in the absence ClO− under ultraviolet lamp irradiation. | |
3.2 Effects of reaction time on sensing ClO−
Reaction time is an important factor for reaction-based probes, and the time-dependent fluorescence spectra of compound 1 in the presence of ClO− were examined. As shown in Fig. 3, the fluorescence intensity of compound 1 at 544 nm shows no obvious variations in the absence of ClO−, which proves that compound 1 is very stable in such a sensing system. When 50 μM and 120 μM of ClO− was added respectively, the fluorescence intensities enhanced within just a few seconds (<10 s) at 544 nm, and the fluorescence intensity remained nearly unchanged over time. Therefore, compound 1 is a good candidate for real-time detection of ClO−.
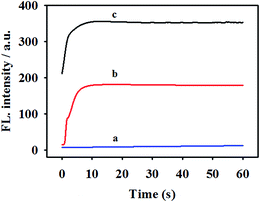 |
| Fig. 3 Time-dependent fluorescence intensity changes of compound 1 (10 μM) in the absence (a), in the presence of 50 μM (b) and the presence of 120 μM ClO− (c) in acetonitrile–water solution (1 : 1 v/v, 50 mM PBS buffer solution at pH 7.4). | |
3.3 Influence of pH value
To test the application extent of compound 1 as a ClO− probe, the fluorescence properties of the compound 1 was investigated both in the absence and presence of ClO− in different pH values ranging from 1 to 12.0 (Fig. 4). As can be seen in Fig. 4, in the absence of ClO−, compound 1 did not exhibit any fluorescence changes in the pH range of 1.0–12.0, and showed weak fluorescence at 544 nm. However, the solution pH is between 4.0 and 12.0, a fluorescence increase was induced in the presence of 12 equiv. ClO−. When the pH value was between 7.0 and 8.0, the strong fluorescence was detectable at 544 nm. This indicated that compound 1 is pH-dependence in the detection of ClO−, and it could be used to detect ClO− at physiological conditions.
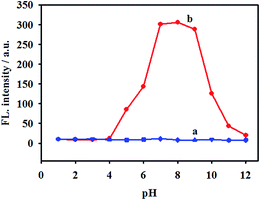 |
| Fig. 4 Fluorescence intensities of 10 μM compound 1 in the absence (a) and the presence of 120 μM (b) in acetonitrile–water solution (1 : 1 v/v, 50 mM PBS buffer) at various pH with 120 μM ClO−. | |
3.4 Selectivity of compound 1
To evaluate the compound 1 as a highly selective chemosensor for ClO− under simulated physiological conditions (pH = 7.4), we investigated the responses of compound 1 to various analytes including a series of ROS/RNS (˙OH, ONOO−, 1O2, O2−, NO, H2O2, t-BuOOH, NO2−, ClO− and NO3−) and metal ions (Fe3+, Cu2+ and Hg2+). As displayed in Fig. 5, the fluorescence of compound 1 at 544 nm was a dramatic increase with ClO−, no obvious fluorescence enhancement was observed for any analyte. The excellent selectivity toward ClO− over other interfering species exhibits that compound 1 has the potential to detect ClO− in biological environment.
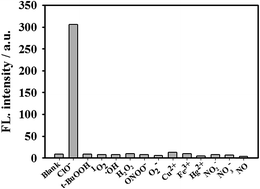 |
| Fig. 5 Fluorescence emission spectra of 10 μM compound 1 in acetonitrile–water solution (1 : 1 v/v, 50 mM PBS buffer solution at pH 7.4) with various analyte (120 μM). | |
3.5 Reversibility of compound 1
To examine reversibility of compound 1, the fluorescence intensity of compound 1 were mediated by ClO− and GSH in CH3CN–H2O solution (1
:
1 v/v, 50 mM PBS buffer solution at pH 7.4). As shown in Fig. 6, when upon addition of ClO− to a solution of compound 1, fluorescence intensity reached a maximum value. Then GSH was added to the above solution, the fluorescence intensity showed decrease and recovered its weak fluorescence. This reversible redox cycle can be repeated at least four times under the same conditions. These results showed that compound 1 could be used to detect ClO− continuously.
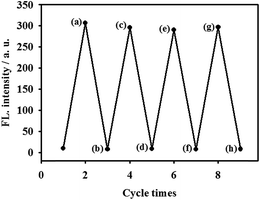 |
| Fig. 6 Fluorescence response monitored at 544 nm after each addition (a), (c), (e) and (g) After addition of 12 equiv. ClO−. (b), (d), (f) and (h) After addition of GSH. | |
3.6 Investigation of sensing mechanism
In our hypothesis, compound 1 exhibited faint fluorescence due to the PET process from the selenide to NBD in the excited state. After the reaction with ClO−, the fluorescence of compound 1 would boost because of the resulting the hampered PET process induced by the formation of selenoxide. Once reduction of selenoxide to selenide was triggered by GSH, the fluorescence switch was turned off, and the compound 1 recovered its weak fluorescence. To verify the proposed mechanism, a Job plot of compound 1 reaction with ClO− was examined, and the result displayed compound 1 reacted ClO− in a 1
:
1 molar ratio (Fig. 7). The mass spectrum of the reaction of compound 1 with NaClO showed one new peaks with m/z of 330.9940, which can be assigned as [1 − O + 1]+. This result indicated that compound 1 with m/z of 336.9814 for [1 + Na]+ can be converted into 1 − O in the presence of NaClO (Fig. S5 in the ESI†). Thus, the experiment results confirmed the proposed reaction mechanism, a possible mechanism was proposed as shown in Scheme 2.
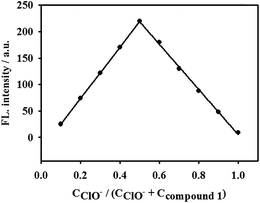 |
| Fig. 7 Job's plot diagram of compound 1 for ClO−. | |
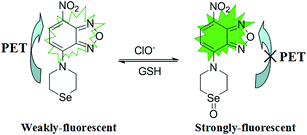 |
| Scheme 2 Proposed mechanism for the response of compound 1 to ClO−. | |
3.7 Biological imaging studies
In order to further explore the potential biological application of this sensor, the real-time imaging of cellular redox cycles was evaluated in Hela cells (Fig. 8). The Hela cells was incubated with compound 1 (10 μM) for 30 minute at 37 °C, and a dark fluorescence was recorded. Then a strong fluorescence was appeared after the addition of 30 μM NaClO into the medium and incubation for another 10 minute at 37 °C, which indicating a good cell-membrane permeability of compound 1. When stimulating compound 1-loaded cells with 100 μM GSH for 10 minute after removal of the remaining NaClO, no fluorescence was observed. This indicates that the NBD-selenoxide was reduced to the nonfluorescent NBD-selenide by GSH. Those experiment results suggested that compound 1 had a good reversibility and could monitor the redox status in cells.
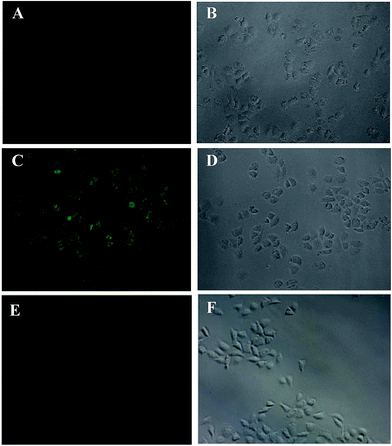 |
| Fig. 8 Fluorescence microscopy images of Hela cells. (A) Images of cells pre-treated with compound 1 (10 μM) for 30 min at 37 °C. (C) Images of cells incubated subsequent with NaClO (30 μM) solution for 30 min at 37 °C. (E) Images of cells further incubated with compound 1 with GSH (100 μM) for 30 min at 37 °C. (B), (D) and (F) Represent the bright-field images of (A), (C) and (E) respectively. | |
4 Conclusion
In summary, we have developed a simple and novel probe for ClO− by combining NBD moiety as the fluorophore and selenide as the recognition unit. Compared with some reported fluorescent probes for hypochlorite determination (Table 1), the proposed fluorescent probe for ClO− displayed several advantages in terms of high selectivity, reversibility, speedy response and a low detection limit. In addition, fluorescent imaging of intracellular showed that compound 1 could be used to evaluate ClO− in biological systems. This strategy may provide a simple method to fabricate a chemical sensor in relevant biological processes of redox biology.
Table 1 A comparison of various approaches to ClO− detection
Detection method |
Detection limit (M) |
Testing media |
Reaction time |
Linearly range (μM) |
Ref. |
Irreversible fluorometry |
1.98 × 10−8 |
DMF/phosphate buffer (20 mM, pH 7.4; 0.5 : 100, v/v) |
6 min |
0–20 |
12a |
Irreversible fluorometry |
4.48 × 10−9 |
HEPES buffer (20 mM, pH 7.4) |
30 min |
0.5–5 |
12m |
Irreversible fluorometry |
10 × 10−9 |
PBS buffer (10 mM, pH 7.4) |
30 min |
0–6.4 |
12p |
Reversible fluorometry |
41.3 × 10−9 |
CH2OH/PBS (100 mM, pH 7.4; 1 : 99, v/v) |
200 s |
0–10 |
21 |
Reversible fluorometry |
5.86 × 10−7 |
PBS buffer (20 mM, pH 7.40) |
15 min |
0–15 |
15d |
Reversible fluorometry |
9.8 × 10−7 |
CH3CN/PBS (20 mM, pH 7.4; 3 : 7, v/v) |
5 min |
0–70 |
15c |
Reversible fluorometry |
3.3 × 10−9 |
CH3CN/PBS (50 mM, pH 7.4; 1 : 1, v/v) |
10 s |
0.05–120 |
This work |
Acknowledgements
This work was supported by the National Natural Science Foundation of China (21342012, 21375037, 21507028), Hunan Provincial Natural Science Foundation of China (11JJ3023, 15JJ3094), Science and Technology Department (13JJ2020), State Key Laboratory of Chemo/Biosensing and Chemometrics Foundation (KLCBTC MR 2011-05), and Scientific Research Fund of Hunan Provincial Education Department (13C635).
References
- C. C. Winterbourn, M. B. Hampton, J. H. Livesey and A. J. Kettle, J. Biol. Chem., 2006, 281, 39860–39869 CrossRef CAS PubMed.
-
(a) J. E. Harrison and J. Schultz, J. Biol. Chem., 1976, 251, 1371–1374 CAS;
(b) Z. M. Prokopowicz, F. Arce, R. Biedron, C. L. Chiang, M. Ciszek, D. R. Katz, M. Nowakowska, S. Zapotoczny, J. Marcinkiewicz and B. M. Chain, J. Immunol., 2010, 184, 824–835 CrossRef CAS PubMed.
- D. I. Pattison and M. J. Davies, Chem. Res. Toxicol., 2001, 14, 1453–1464 CrossRef CAS PubMed.
- S. Sugiyama, K. Kugiyama, M. Aikawa, S. Nakamura, H. Ogawa and P. Libby, Arterioscler., Thromb., Vasc. Biol., 2004, 24, 1309–1314 CrossRef CAS PubMed.
- A. Daugherty, J. L. Dunn, D. L. Rateri and J. W. Heinecke, J. Clin. Invest., 1994, 94, 437–444 CrossRef CAS PubMed.
- V. Rudolph, R. P. Andrie, T. K. Rudolph, K. Friedrichs, A. Klinke, B. Hirsch-Hoffmann, A. P. Schwoerer, D. Lau, X. M. Fu, K. Klingel, K. Sydow, M. Didie, A. Seniuk, E. C. von Leitner, K. Szoecs, J. W. Schrickel, H. Treede, U. Wenzel, T. Lewalter, G. Nickenig, W. H. Zimmermann, T. Meinertz, R. H. Boger, H. Reichen-spurner, B. A. Freeman, T. Eschenhagen, H. Ehmke, S. L. Hazen, S. Willems and S. Baldus, Nat. Med., 2010, 16, 470–474 CrossRef CAS PubMed.
- M. J. Steinbeck, L. J. Nesti, P. F. Sharkey and J. Parvizi, J. Orthop. Res., 2007, 25, 1128–1135 CrossRef CAS PubMed.
- W. Y. Lin and L. L. Long, Chem.–Eur. J., 2009, 15, 2305–2309 CrossRef CAS PubMed.
- M. Benhar, D. Engelberg and A. Levitzki, EMBO Rep., 2002, 3, 420–425 CrossRef CAS PubMed.
- M. Whiteman, P. Rose, J. L. Siau, N. S. Cheung, G. S. Tan, B. Halliwell and J. S. Arm-strong, Free Radical Biol. Med., 2005, 38, 1571–1584 CrossRef CAS PubMed.
-
(a) A. Maritim, R. Sanders and J. Watkins III, J. Biochem. Mol. Toxicol., 2003, 17, 24–38 CrossRef CAS PubMed;
(b) I. Juránek and S. Bezek, Gen. Physiol. Biophys., 2005, 24, 263–278 Search PubMed.
-
(a) Z. R Lou, P. Li, P. Song and K. L Han, Analyst, 2013, 138, 6291–6295 RSC;
(b) J. J. Hu, N. K. Wong, Q. Gu, X. Bai, S. Ye and D. Yang, Org. Lett., 2014, 16, 3544–3547 CrossRef CAS PubMed;
(c) L. Yuan, W. Lin, J. Song and Y. Yang, Chem. Commun., 2011, 47, 12691–12693 RSC;
(d) S. Yu, C. Hsu, W. Chen, L. Wei and S. Wu, Sens. Actuators, B, 2014, 196, 203–207 CrossRef CAS PubMed;
(e) Y. Koide, Y. Urano, K. Hanaoka, T. Terai and T. Nagano, J. Am. Chem. Soc., 2011, 133, 5680–5682 CrossRef CAS PubMed;
(f) S. R. Liu and S. P. Wu, Org. Lett., 2013, 15, 878–881 CrossRef CAS PubMed;
(g) S. R. Liu, M. Vedamalai and S. P. Wu, Anal. Chim. Acta, 2013, 800, 71–76 CrossRef CAS PubMed;
(h) Q. Xu, K. A. Lee, S. Lee, K. M. Lee, W. J. Lee and J. Yoon, J. Am. Chem. Soc., 2013, 135, 9944–9949 CrossRef CAS PubMed;
(i) M. Emrullahoglu, M. Ucuncu and E. Karakus, Chem. Commun., 2013, 49, 7836–7838 RSC;
(j) G. Cheng, J. Fan, W. Sun, J. Cao, C. Hu and X. Peng, Chem. Commun., 2014, 50, 1018–1020 RSC;
(k) X. Cheng, H. Jia, T. Long, J. Feng, J. Qin and Z. Li, Chem. Commun., 2011, 47, 11978–11980 RSC;
(l) J. Kim and Y. Kim, Analyst, 2014, 139, 2986–2989 RSC;
(m) J. Li, F. Huo and C. Yin, RSC Adv., 2014, 4, 44610–44613 RSC;
(n) Y. Zhou, J. Y. Li, K. H. Chu, K. Liu, C. Yao and J. Y. Li, Chem. Commun., 2012, 48, 4677–4679 RSC;
(o) F. Liu, Y. Gao, J. Wang and S. Sun, Analyst, 2014, 139, 3324–3329 RSC;
(p) G. Li, D. Zhu, Q. Liu, L. Xue and H. Jiang, Org. Lett., 2013, 15, 2002–2005 CrossRef CAS PubMed.
-
(a) Y. Koide, M. Kawaguchi, Y. Urano, K. Hanaoka, T. Komatsu, M. Abo, T. Teraia and T. Nagano, Chem. Commun., 2012, 48, 3091–3093 RSC;
(b) B. C. Dickinson, J. Peltier, D. Stone, D. V. Schaffer and C. J. Chang, Nat. Chem. Biol., 2011, 7, 106–112 CrossRef CAS PubMed;
(c) E. W. Miller, S. X. Bian and C. J. Chang, J. Am. Chem. Soc., 2007, 129, 3458–3459 CrossRef CAS PubMed.
- J. T. Rotruck, A. L. Pope, H. E. Ganther, A. B. Swanson, D. G. Hafeman and W. G. Hoekstra, Science, 1973, 179, 588–590 CAS.
-
(a) B. Wang, P. Li, F. Yu, J. Chen, Z. Qu and K. Han, Chem. Commun., 2013, 49, 5790–5792 RSC;
(b) S. R. Liu and S. P. Wu, Org. Lett., 2013, 15, 878–881 CrossRef CAS PubMed;
(c) B. S. Wang, P. Li, F. B. Yu, P. Song, X. F. Sun, S. Q. Yang, Z. R. Lou and K. L. Han, Chem. Commun., 2013, 49, 1014–1016 RSC;
(d) Z. R. Lou, P. Li, Q. Pan and K. L. Han, Chem. Commun., 2013, 49, 2445–2447 RSC.
- X. Q. Chen, X. Z. Tian, I. Shin and J. Y. Yoon, Chem. Soc. Rev., 2011, 40, 4783–4804 RSC.
- S. T. Manjare, J. Kim, Y. Lee and D. G. Churchill, Org. Lett., 2014, 16, 520–523 CrossRef CAS PubMed.
- L. D. Lavis and R. T. Raines, ACS Chem. Biol., 2008, 3, 142–155 CrossRef CAS PubMed.
-
(a) W. Jiang, Q. Fu, H. Fan and W. Wang, Chem. Commun., 2008, 259–261 RSC;
(b) F. Qian, C. Zhang, Y. Zhang, W. He, X. Gao, P. Hu and Z. Guo, J. Am. Chem. Soc., 2009, 131, 1460–1468 CrossRef CAS PubMed;
(c) S. Banthia and A. Samanta, New J. Chem., 2005, 29, 1007–1010 RSC;
(d) S. H. Kim, J. S. Kim, S. M. Park and S. K. Chang, Org. Lett., 2006, 8, 371–374 CrossRef CAS PubMed;
(e) Y. M. Shen, Y. Y. Zhang, X. Y. Zhang, C. X. Zhang, L. L. Zhang, J. L. Jin, H. T. Li and S. Z. Yao, Anal. Methods, 2014, 6, 4797–4802 RSC;
(f) Z. Xie, K. Wang, C. Zhang, Z. Yang, Y. Chen, Z. Guo, G.-Y. Lu and W. He, New J. Chem., 2011, 35, 607–613 RSC;
(g) H. J. Jeong, Y. Li and M. H. Hyun, Bull. Korean Chem. Soc., 2011, 32, 2809–2812 CrossRef;
(h) Y. B. Ruan, S. Maisonneuve and J. Xie, Dyes Pigm., 2011, 90, 290–296 CrossRef PubMed;
(i) H. Sakamoto, J. Ishikawa, S. Nakao and H. Wada, Chem. Commun., 2000, 2395–2396 RSC.
- L. M. Hu, Z. Y. Chen, S. M. Lu, X. S. Li, Z. J. Liu and H. S. Xu, Phosphorus, Sulfur Silicon Relat. Elem., 2004, 179, 1065–1073 CrossRef CAS PubMed.
- P. Venkatesan and S. P. Wu, Analyst, 2015, 140, 1349–1355 RSC.
- G. A. Crosby and J. N. Demas, J. Phys. Chem., 1971, 75, 991–1024 CrossRef CAS.
Footnote |
† Electronic supplementary information (ESI) available. See DOI: 10.1039/c5ra15373e |
|
This journal is © The Royal Society of Chemistry 2015 |
Click here to see how this site uses Cookies. View our privacy policy here.