DOI:
10.1039/C5RA15328J
(Paper)
RSC Adv., 2015,
5, 82153-82158
Naturally occurring Batatasins and their derivatives as α-glucosidase inhibitors
Received
1st August 2015
, Accepted 22nd September 2015
First published on 22nd September 2015
Abstract
Batatasins are endogenous plant hormones found in yams and other related plant species. These plants are widely consumed as botanical dietary supplements in many parts of the world. This study investigated the inhibitory effects and mechanisms of Batatasin I, III, IV, V against α-glucosidase regarding their antidiabetic activities. The results revealed that Batatasin I, III, IV inhibited α-glucosidase in a reversible and noncompetitive manner, with IC50 values of 2.55, 1.89 and 2.52 mM respectively, while Batatasin V completely abolished its inhibitory activity even at the highest concentrations tested. Furthermore, a class of Batatasin-derived compounds with different substitution patterns was synthesized and subjected to the assay to clarify the structure–activity relationships, which suggested that the hydroxyl at the C-2′ position may play a significant role in improving the inhibitory activities. Their probable binding modes and the specificity of the binding sites were studied using molecule docking simulation. It was concluded that Batatasins, especially Batatasin III and IV, are promising α-glucosidase inhibitors, which therefore could be used as functional food to alleviate postprandial hyperglycemia and as potential candidates for the development of antidiabetic agents.
Introduction
Type-2 diabetes, as a health-threatening concomitant disease all over the world, is a chronic metabolic disease characterized by major imbalances in glucose metabolism and abnormalities in fat.1,2 Therapies focusing on achieving normal glycemia are proven to be effective in improving postprandial glycemic control and delaying the progression of long-term diabetic complications.3,4 One of the approaches currently used is the reversible inhibition of α-glucosidase involved in the digestion of carbohydrates, which delays the absorption of glucose from the small intestine and thus leads to a lowering effect on postprandial blood glucose and insulin levels.5,6 Beyond that, α-glucosidase is also involved in biosynthesis of glycoproteins, including promotion of protein folding and stabilisation of cell-surface glycoproteins.7,8 Hence, α-glucosidase inhibitors have also been well appreciated as therapeutic agents for the treatment of such diseases as viral diseases, cancer, and chronic heart failure.9–11
To date, only three antidiabetic agents, acarbose, miglitol, and voglibose belonging to this category are clinically used to retard carbohydrate digestion to a moderate extent. However, they are all structurally confined to glycosidic derivatives and required multiple steps from the carbohydrates.12 Recent warnings about adverse effects of those drugs, such as flatulence, diarrhea and hepatotoxicity in chronic therapy, highlight the urgent of alternative and safer treatments to control postprandial glucose levels.13 In the course of looking for novel, easily accessible and chemically stable α-glucosidase inhibitors, plant-based foods and dietary bioactives are more acceptable sources for the screening of practicable candidates due to their diversity and relative safety.14,15
Dioscorea opposita Thunb, commonly known as “yam”, is widely consumed as botanical dietary supplements in many areas of tropical America, Africa and Asia. This species, alone or in combination with other food, has a long dietary therapy history for the diabetes and widely cultivated in China. However, there is a limited understanding of its antidiabetic constituents and corresponding mechanisms. Batatasins are endogenous plant hormones isolated from yams and further related plant species like Dioscorea cayenesis Lam, D. dumetorum Pax, and D. composite Hemsl,16–18 which have been recognised as an important factor in the dormancy of yams bulbils related to gibberellic acid and abscisic acid metabolism. Numerous pharmacological studies had indicated that Batatasins possessed variously beneficial health effects, such as anti-inflammatory, antifungal, radical scavenging and immune system modulator.19–21 In the previous studies, we had shown that Batatasin I led to a remarkable inhibitory effect against α-glucosidase.22 However, the inhibitory effects of other Batatasins on α-glucosidase have not been assessed yet and their pharmacological mechanisms remain to be clarified. In response to these issues, we synthesized Batatasin I, III, IV, V and evaluated their inhibitory effects on α-glucosidase. Lineweaver–Burk plots were further employed in the kinetic enzymatic assays to determine the mechanism of enzymatic inhibition. The probable binding modes and the specificity of the binding sites were studied by using molecule docking simulation. To gain further insight into their structure–activity correlations, a class of Batatasin derivatives with hydroxyl and/or methoxyl substitutions was also synthesized and subjected to the enzymatic assay, which provide the possible guidelines for future rational design of potent Batatasin-derived α-glucosidase inhibitors.
Materials and methods
Chemicals and materials
All solvents used in this research were of analytical grade, and the chemicals used for synthesizing the derivatives were of reagent grade and commercially available. The baker's yeast α-glucosidase (EC 3.2.1.20), pNPG (4-nitrophenyl-α-D-glucopyranoside), and acarbose were purchased from Sigma-Aldrich Chemical (St. Louis, MO, USA). All other chemicals, including material and reagents, were purchased from Aladdin Chemical Ltd (Shanghai, China).
General experimental procedures
The NMR spectra were recorded at 400 MHz on a Bruker Avance 400 spectrometer with TMS as an internal standard. J values were reported in Hertz. High-resolution TOF-MS experiments were carried with a Qstar Elite mass spectrometer (AB Sciex, America). The IR spectra were measured with the AVATAR 360 infrared spectrometer (Nicolet, America). Measurement of α-glucosidase activities were performed with the DNM-9606 microplate reader (Beijing, China).
Synthesis of Batatasins and their derivatives
Batatasins derivatives were prepared according to the method described for Batatasins with modification.23 A generalized synthetic approach for the proposed compounds was illustrated in Fig. 1, which was efficiently synthesized via Wittig reaction and stilbene reduction procedure as key steps. Briefly, functionally-substituted benzyl alcohol 2d–10d, which were synthesized according to the procedures described in literatures,24–26 were reacted with phosphorus tribromide respectively to obtain the Br-substituted intermediates 2c–10c. These intermediates were then used by adding stoichiometric triethyl phosphate to form the stilbene derivatives 2b–10b, which underwent the intramolecular ring-closure via the Wittig olefination reaction at room temperature. The intermediates 2b–10b were submitted to the catalytic hydrogenolysis under a hydrogen atmosphere to give the target compounds 2–10. Compound 1 with a phenanthrene skeleton was prepared by the following strategy, where the intermediate 1b (4-benzyloxy-3,3′,5-trimethoxystibene) was irradiated with UV light in the presence of catalytic iodine and then adopted to the reduction procedure under the same condition as described above to obtain Batatasin I.
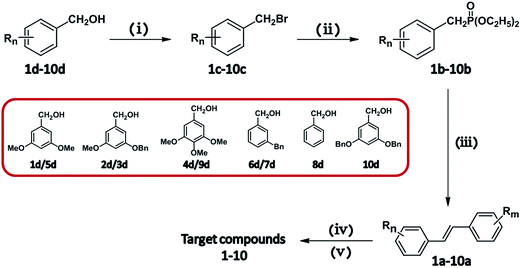 |
| Fig. 1 Synthesis of Batatasins and their derivatives. Reagents and conditions: (i) PBr3, CH2Cl2, rt, 2 h; (ii) P(OEt)3, tetrabutylammonium bromide, 125 °C, 4 h; (iii) NaH, THF, rt, 3 h; (iv) cyclohexane, I2, hν, 8 h; (v) Pd–C, H2, MeOH, room temperature, 12 h. | |
Inhibitory activity against α-glucosidase
The α-glucosidase enzymatic assay was performed according to the methods described by Chen and Wang27,28 with some minor modifications. This assay used pNPG as the substrate, which was hydrolyzed by α-glucosidase to release p-nitrophenol, a color agent that can be monitored at 405 nm. Briefly, 20 μL of a sample solution was mixed with 20 μL of the enzyme solution (0.45 U/mL) in 0.1 M phosphate buffer (pH 6.8) and incubated at 37 °C for 5 min under shaking. After that, 40 μL of 2.5 mM pNPG solution in the above buffer was added to initiate the colorimetric reaction. After incubation at 37 °C for 10 min, 80 μL of 0.2 M sodium carbonate solution was added to stop the reaction, the total volume was 160 μL, and the released p-nitrophenol was monitored at 405 nm by the microplate reader. Acarbose was used as a positive control. The inhibitory activity was calculated by the following equation:
Inhibition (%) = (Acontrol − Asample)/Acontrol × 100 |
where Acontrol is the p-nitrophenol resulting from the enzymatic hydrolysis without inhibitor, and Asample is the p-nitrophenol resulting from the enzymatic hydrolysis in the presence of the inhibitor. All of the analyses were performed in triplicate.
Kinetics of α-glucosidase inhibitors
In this study, the type of the inhibitive mode of the enzyme inhibitors were characterised by the kinetic method. Typically, different concentrations (0–5 mM) of each inhibitor around the IC50 values were chosen. Under each concentration, α-glucosidase activity was assayed by varying the concentration of PNP glycoside (0.25, 0.5, 0.75, 1.0 and 1.5 mM), respectively. The enzymatic assay was performed according to the above-mentioned reaction conditions. The inhibitory kinetics of the investigated compounds on α-glucosidase was analysed using the Lineweaver–Burk plots 1/v versus 1/[S].
Molecular modeling and docking
AutoDock Tools were employed to assign Gasteiger partial charges and merge non-polar hydrogen atoms. The rigid protein structure of α-glucosidase (ID: 4J5T) was downloaded from the Protein Data Bank as a receptor for the docking calculations. It was suggested that the C-domain residues of Asp568 and Glu771 affect substrate orientation and metabolism that play critical roles in substrate recognition and binding processes.29 Mutations of these residues often led to dramatic changes in the kinetics of the catalytic reaction. The docking simulations were performed with the Lamarckian genetic algorithm for at least 80 docking runs for each ligand. An extended model was employed to estimate the energetics of the unbound states of the receptor and the ligands when evaluating their binding free energies (ΔG), which mainly involved two steps: (i) the intramolecular energetics was estimated for the transition from unbound states to the conformation of the ligand and protein in the bound state; (ii) evaluation of the intermolecular energetics of combining the ligand and protein in their bound conformations.
Statistical analysis
All of the samples were run at least in triplicate. The data obtained in this study were dealt with using GraphPad Prism 5 and Origin 8 software. Values were expressed as means ± standard deviations and the difference was considered significant at P ≤ 0.05.
Results and discussion
α-Glucosidase inhibitory activity and structure–activity relationships
The enzymatic inhibitory activities of compounds 1–10 against α-glucosidase were assayed in the experimental section. The IC50 values for all samples were calculated and listed in Fig. 2. Since the smaller IC50 value means the stronger inhibitory activity, Batatasin I, III, and IV exhibited potent inhibitory activities against α-glucosidase, more than acarbose (IC50 = 3.52 mM). The results showed that even 2.55 mM of Batatasin I, 1.89 mM of Batatasin III and 2.52 mM of Batatasin IV had been enough to inhibit 50% of the α-glucosidase activity, which demonstrated the potential of Batatasins, especially Batatasin III and IV, as a new type of natural α-glucosidase inhibitors.
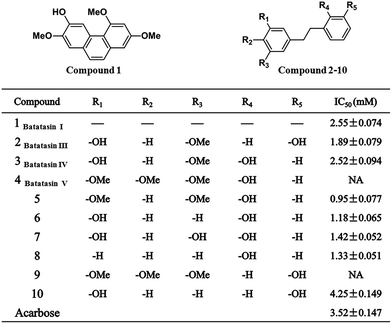 |
| Fig. 2 Inhibitory activities on α-glucosidase (values are the mean of at least three independent determinations. NA means no inhibition). | |
It is interesting to note that in the enzymatic activity assay, Batatasin V completely abolished its inhibitory actions, which encouraged us to explore the structure–activity relationships of Batatasins and their derivatives. As an evident from the Fig. 3, Compound 5 was the most potent α-glucosidase inhibitor with an IC50 value of 0.95 mM, being about 4 times more potent than acarbose. The remaining compounds, apart from compound 9, had significantly reduced the enzymatic activities with IC50 in the range of 0.95–4.25 mM. As the main skeleton for all compounds is same, the differences in their inhibitory potential would be due to the different substitution patterns on bibenzyl. It is worthy to note that compound 3, 6, 7 and 8 bearing hydroxyl at the C-2′ position exhibited considerably enhanced inhibitory activities, while compounds 2 and 10, with the same substitution at the C-3′ position, led to a large decrease in their inhibition effects suggesting that introduction of hydroxyl group at the C-2′ position plays a more important role in improving the inhibitory activities against α-glucosidase.
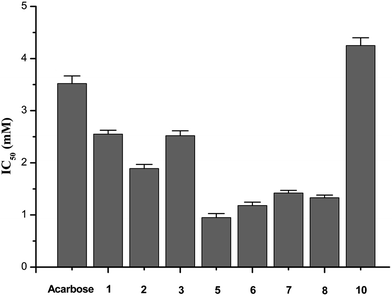 |
| Fig. 3 The comparison of IC50 values between the inhibitors and the control. | |
Moreover, Batatasin V did not show any inhibitory activity even at the highest concentrations tested, illustrating that 3,4,5-trimethoxy substitutions on the A ring might be a crucial factor for their ineffective inhibition effects, which was confirmed further by the similar phenomenon observed in compound 9. Contrarily, when substituted with a 3,5-dimethoxy moiety, compound 5 drastically increased its inhibitory activity, suggesting the moderately deactivating groups at the C-4 position could enhance the affinity between inhibitors and enzyme. These results indicated that the relatively small structural changes in the skeleton of bibenzyl resulted in the significant differences in their abilities to inhibit α-glucosidase.
The inhibition kinetics of α-glucosidase
In order to clarify the inhibitory characters of these compounds, the mechanisms of these inhibitors binding to the α-glucosidase were further studied by analyzing their inhibitory kinetics. The inhibition of α-glucosidase by compound 3 (Batatasin IV) and compound 5 (the most effective specie) were illustrated in Fig. 4 representatively. It showed that plots of the initial velocity versus enzyme concentrations in the absence and presence of compounds gave a family of straight lines, all of which passed through the origin. Increasing the inhibitor concentrations resulted in lowering of the slope of the line, which suggested these compounds were reversible inhibitors.30
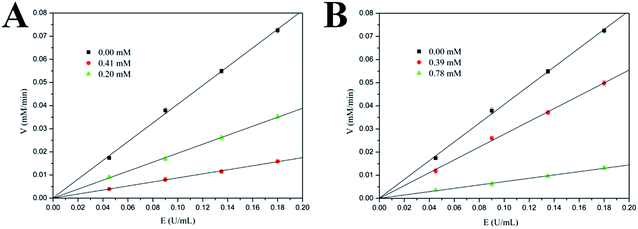 |
| Fig. 4 The inhibitory mechanism of α-glucosidase with different concentrations of compound 3 and 5. | |
Furthermore, the inhibition types of the representative α-glucosidase inhibitors were explored by Lineweaver–Burk plots, a linear double-reciprocal plot of the substrate concentration and reaction velocity.31 As shown in Fig. 5, the double-reciprocal plots for all of the inhibitors and the control differed in slopes, and the intercepts on the x-axis remained nearly constant, indicating that the type of inhibition belonged to the noncompetitive mode. Additionally, similar trends were observed in the remaining compounds. The inhibition mode of Batatasins and their derivatives belonging to the noncompetitive type was different from that of acarbose, which behaved as a typical competitive inhibitor.32 It is suggested that competitive inhibition is usually reversible and an excessive amount of substrate would displace the inhibitor, while noncompetitive inhibition like that of Batatasins will not be influenced by the substrate concentrations. These above findings supported the possibility that Batatasins and their derivatives bind to the noncompetitive domain of yeast's α-glucosidase rather than to its active site.
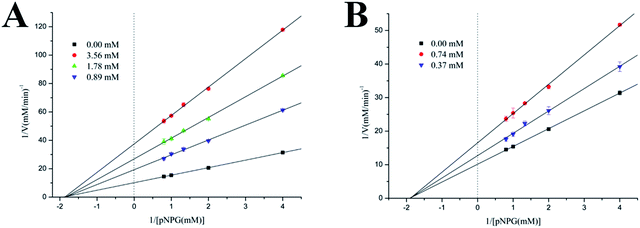 |
| Fig. 5 Lineweaver–Burk plot of PNP release by α-glucosidase in the presence of compound 3 and 5 in phosphate buffer (pH 6.8) at 37 °C. | |
Thermodynamics of interaction between ligands and α-glucosidase
To provide further insight into the binding model of inhibitors toward α-glucosidase as well as into their impacts on the activity of the enzyme at the noncompetitive domain, a molecular docking study was performed using the program AutoDock4. Docking of Batatasins and their derivatives to α-glucosidase resulted in a position for the ligand coinciding with that of the resolved crystal structure (4J5T; 2.04 Å) and confirmed the validity of the docking procedure. As shown in Fig. 6, all these inhibitors were situated appropriately for enzymatic interaction with Asp568 and Glu771.
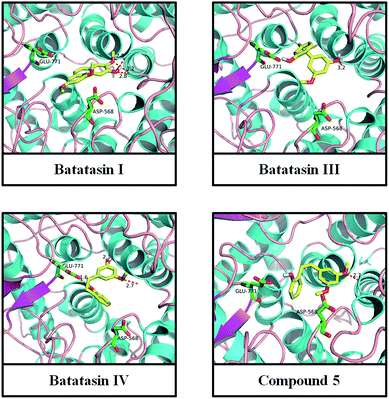 |
| Fig. 6 3D representation of the interaction of Batatasin I, III, IV and compound 5 in the binding site predicted. Dotted red lines represent hydrogen bonds connecting hydroxyl and amino groups with amino acid residues. | |
Interestingly, significant H-bonding interactions of ligands with the amide backbone were observed in these binding sites. For example, the C-5 and C-2′ hydroxyl groups of Batatasin IV form H-bonds with the key residues Asp568 and Glu771, while the H-bonds of C-3′ hydroxyl of Batatasin III was not observed, which verified the aforementioned inference that hydroxyl group at the C-2′ position had more important impact than that of C-3′ position substitution. Similar hydrogen bonds had also been found between the remaining compounds and the amide backbone residues, especially with Asp568, suggesting that H-bonding interaction played a key role in the binding modes of Batatasins and their derivatives. Beyond that, binding free energies in these binding modes were calculated ranging from −4.7 to −6.6 kJ mol−1 with compound 5 being the highest binding affinity, which was in full accordance with the kinetic results. Taken together, the above results indicated that the strong binding interaction of Batatasins and their derivatives to the residues Asp568 and Glu771 of α-glucosidase would probably perturb the protein structure, which may lead to the significant inhibition activities toward α-glucosidase.
Conclusion
In summary, our results revealed that Batatasins, especially Batatasin III and IV are promising α-glucosidase inhibitors, which could be used as potential candidates for the development of antidiabetic agents. Kinetic enzymatic analysis indicated that they inhibit α-glucosidase in a noncompetitive and reversible manner. In order to clarify the structure–activity relationships of Batatasins, a series of derivatives with hydroxyl and/or methoxyl substitutions was synthesized and investigated, which provide the possible guidelines for future rational design of potent Batatasin-derived α-glucosidase inhibitors. In addition, as Batatasins are naturally occurred within the tropical genus Dioscorea including D. opposita Thunb, D. dumetorum Pax and D. composite Hemsl and these vegetables are widely consumed as botanical dietary supplements in many areas of the world, this might be important for the role of these vegetables in dietary recommendations to inhibit or retard carbohydrate digestion to a moderate extent.
Acknowledgements
This work is supported by the grants from the National Technology R&D Program for the 12th Five-year Plan (No. 2011BAI06B04), the National Natural Science Foundation of China and Henan province (No. U1204304), the Natural Science Research Program of Henan Province of China (No. 13A150053). College Science and technology innovation team program of Henan Province (No. 14IRTSTHN030).
References
- J. C. Pickup and M. A. Crook, Diabetologia, 1998, 41, 1241–1248 CrossRef CAS PubMed.
- A. S. Boath, D. Stewart and G. J. McDougall, Food Chem., 2012, 135, 929–936 CrossRef CAS PubMed.
- K. R. Rengasamy, M. A. Aderogba, S. O. Amoo, W. A. Stirk and J. van Staden, Food Chem., 2013, 141, 1412–1415 CrossRef CAS PubMed.
- S. Mohan, R. Eskandari and B. M. Pinto, Acc. Chem. Res., 2013, 47, 211–225 CrossRef PubMed.
- S. Lordan, T. J. Smyth, A. Soler-Vila, C. Stanton and R. P. Ross, Food Chem., 2013, 141, 2170–2176 CrossRef CAS PubMed.
- F. A. van de Laar, P. L. Lucassen, R. P. Akkermans, E. H. van de Lisdonk, G. E. Rutten and C. van Weel, Diabetes Care, 2005, 28, 154–163 CrossRef CAS.
- K. Takada, T. Uehara, Y. Nakao, S. Matsunaga, R. W. van Soest, N. Fusetani and A. C. Schulzeines, J. Am. Chem. Soc., 2004, 126, 187–193 CrossRef CAS PubMed.
- C. M. de Praeter, G. J. Gerwig, E. Bause, L. K. Nuytinck, J. F. Vliegenthart, W. Breuer and R. N. van Coster, Am. J. Hum. Genet., 2000, 66, 1744–1756 CrossRef CAS PubMed.
- M. P. Courageot, M. P. Frenkiel, C. D. Dos Santos, V. Deubel and P. Desprès, J. Virol., 2000, 74, 564–572 CrossRef CAS.
- R. Pili, J. Chang, R. A. Partis, R. A. Mueller, F. J. Chrest and A. Passaniti, Cancer Res., 1995, 55, 2920–2926 CAS.
- K. Node, Hypertens. Res., 2006, 29, 741–742 CrossRef CAS PubMed.
- X. J. Hu, X. B. Wang and L. Y. Kong, J. Agric. Food Chem., 2013, 61, 1501–1508 CrossRef CAS PubMed.
- S. E. Inzucchi, Jama, 2002, 287, 360–372 CrossRef CAS PubMed.
- S. Kumar, S. Narwal, V. Kumar and O. Prakash, Pharmacogn. Rev., 2011, 5, 19 CrossRef CAS PubMed.
- S. Liu, D. Li, B. Huang, Y. Chen, X. Lu and Y. Wang, J. Ethnopharmacol., 2013, 149, 263–269 CrossRef CAS PubMed.
- T. Hashimoto, K. Hasegawa and A. Kawarada, Planta, 1972, 108, 369–374 CrossRef CAS PubMed.
- T. Hashimoto and M. Tajima, Phytochemistry, 1978, 17, 1179–1184 CrossRef CAS.
- C. R. Ireland, W. W. Schwabe and D. G. Coursey, Phytochemistry, 1981, 20, 1569–1571 CrossRef CAS.
- M. H. Yang, Y. W. Chin, K. D. Yoon and J. Kim, J. Enzyme Inhib. Med. Chem., 2013, 29, 1–6 CrossRef PubMed.
- S. Boonphong, P. Puangsombat, A. Baramee, C. Mahidol, S. Ruchirawat and P. Kittakoop, J. Nat. Prod., 2007, 70, 795–801 CrossRef CAS PubMed.
- D. C. Rueda, A. Schöffmann, M. de Mieri, M. Raith, E. A. Jähne, S. Hering and M. Hamburger, Bioorg. Med. Chem., 2014, 22, 1276–1284 CrossRef CAS PubMed.
- L. Zhang, B. Bai, X. Liu, Y. Wang, M. Li and D. Zhao, Food Chem., 2011, 126, 203–206 CrossRef CAS PubMed.
- T. Hashimoto, K. Hasegawa, H. Yamaguchi, M. Saito and S. Ishimoto, Phytochemistry, 1974, 13, 2849–2852 CrossRef CAS.
- C. C. Nawrat, L. I. Palmer, A. J. Blake and C. J. Moody, J. Org. Chem., 2013, 78, 5587–5603 CrossRef CAS PubMed.
- S. Venkateswarlu, M. S. Raju and G. V. Subbaraju, Biosci., Biotechnol., Biochem., 2002, 66, 2236–2238 CrossRef CAS PubMed.
- G. Husemoen, R. Olsson, C. M. Andersson, S. C. Harvey and H. C. Hansen, J. Comb. Chem., 2003, 5, 606–609 CrossRef CAS.
- Q. You, F. Chen, X. Wang, P. G. Luo and Y. Jiang, J. Agric. Food Chem., 2011, 59, 9506–9511 CrossRef CAS PubMed.
- S. Y. Wang, M. J. Camp and M. K. Ehlenfeldt, Food Chem., 2012, 132, 1759–1768 CrossRef CAS PubMed.
- M. K. Barker and D. R. Rose, J. Biol. Chem., 2013, 288, 13563–13574 CrossRef CAS PubMed.
- H. W. Ryu, B. W. Lee, M. J. Curtis-Long, S. Jung, Y. B. Ryu, W. S. Lee and K. H. Park, J. Agric. Food Chem., 2009, 58, 202–208 CrossRef PubMed.
- H. He and Y. H. Lu, J. Agric. Food Chem., 2013, 61, 8110–8119 CrossRef CAS PubMed.
- M. Liu, H. Yin, G. Liu, J. Dong, Z. Qian and J. Miao, Xanthohumol, J. Agric. Food Chem., 2014, 62, 5548–5554 CrossRef CAS PubMed.
|
This journal is © The Royal Society of Chemistry 2015 |