DOI:
10.1039/C5RA14396A
(Paper)
RSC Adv., 2015,
5, 73363-73372
Odd–even effect on the thermal properties of Schiff base functionalized dicyanate esters and thermo-mechanical properties of their blends with epoxy resins†
Received
21st July 2015
, Accepted 24th August 2015
First published on 24th August 2015
Abstract
An odd–even effect was observed in the case of dicyanate esters described in this work. The diamines were converted into their corresponding bisphenols by reacting with 2-hydroxybenzaldehyde and followed by treatment with cyanogen bromide which resulted in the dicyanate ester (CE). The synthesised dicyanate esters were confirmed by FT-IR and NMR spectral studies. The curing studies of the dicyanate esters were measured by using differential scanning calorimetry (DSC). The thermal properties depend on the length of the alkyl spacer. The maximum curing temperatures (Tmax) of these cyanate esters were in the range of 229–278 °C. The 5% weight loss temperature (T5%) was found to be in the range of 415 to 467 °C and the 10% weight loss temperature (T10%) ranged from 509 to 530 °C. The char yield of these polycyanurate networks is found to be in the range of 70.78 to 73.12 at 700 °C. Scanning electron microscopy (SEM) analysis reveals brittle behavior for the neat cured dicyanates while that of CE/epoxy blends show uniform dispersion of the cyanate ester. The storage modulus and glass transition temperature of cyanate/epoxy ester blends were found to decrease slightly with increasing cyanate ester content (1–10%). From the polarized optical microscope studies, all the uncured dicyanate esters show crystalline properties.
1. Introduction
Thermosetting resins are generally used to make fiber reinforced composites (FRC) which are being used in electronics, aeronautical and automobile industries for their superior strength. Among the thermosetting resins, epoxy resins have occupied the majority of the commercial market due to their high-performance. Epoxy resins are usually used as matrix resins for structural composites because of their processability, ease of handling, moderate chemical resistance and mechanical properties and low shrinkage on curing. Subsequently, many approaches have been explored in an effort to improve the properties of epoxy resins.
The fracture toughness of the epoxy resins can be improved by using fillers such as rubber1 and nano cellulose.2 The elastic modulus can be improved by adding nanoclay,3 or blending with other thermosetting resins such as bismaleimides4 and polybenzoxazines.5 The epoxy backbone may be modified to improve their thermal stability and flame retardancy. Modification of epoxy resins have been attempted, for example halogenated epoxy resins are widely exploited for circuit board applications for their excellent flame retardancy.6 But, they offer low thermal stability and results in release of toxic, corrosive and halogenated gases as well as endocrine disrupting chemicals during their decomposition or incineration. Phosphorus-containing epoxy polymers were studied extensively by Wang and Liu et al.7 They exhibit high flame retardancy, however the presence of phosphorus cause debatable environmental issues. The reaction between the cyano groups and the epoxy ring leads to the formation of oxazolidinone structure which results in enhanced thermal and mechanical properties of cyanate/epoxy blends.8
Among the thermosetting resins, cyanate esters are widely used in electronics and aerospace applications. Cyanate esters are versatile materials due to the high glass transition temperature,9 high thermal stability,10 low dielectric constant9 and good adhesion. In particular, cyanate esters are used in the preparation of electrical9 and structural laminates, potting formulations, molding formulations, etc. Cyanate esters show excellent relationship between their structural designing and processing. On curing, cyanate esters undergo cyclotrimerization reaction resulting in the formation of a cross-linked resin. Previously we have reported that, incorporation of carbon nano tube in the cyanate ester gels further improved the cure behavior and thermal stability.11 They blend easily with other thermosetting resins like epoxy resins,5,12 bismaleimides13,14 and polybenzoxazines,5 thermoplastic resins15 (toughening with thermoplastic resins leads to phase separation). Most of the blends were prepared using the commercially available BADCY and epoxy resin. The resultant material is insoluble in solvent and also shows high glass transition temperature. The chemistry and kinetics of curing reaction of cyanate esters have been studied in detail in the presence of catalyst.16 The degree of conversion can be calculated by DiBenedetto equation.17
Earlier, azomethine functionalized cyanate ester and its BMI blends were prepared and characterized. On blending Schiff base functionalized cyanate ester into with resin, the mechanical and physico-chemical properties were improved without significant sacrifice in the thermal properties of the BMI.18
In this paper, the effect of placing the cyanate group in the ortho position and increasing the cyanate ester content in epoxy/cyanate ester blend system are reported. Three different dicyanate esters with ether and azomethine linkages were prepared and then blended with epoxy resin at various compositions. The dicyanate esters were cured by heating in the absence of catalyst and diamino diphenyl methane as the curing agent for the epoxy resin. Thermal properties and morphology of the CE/epoxy blends are also discussed in this paper.
2. Experimental procedure
2.1. Materials
Potassium carbonate (K2CO3), cyanogen bromide (CNBr), acetone and 2-hydroxy benzaldehyde were purchased from Sisco Research Laboratories (SRL-India). Ethanol and triethylamine (Et3N) were purchased from E-Merck (India). Triethylamine and acetone were distilled prior to use. Epoxy resin (DGEBA) was purchased from Huntsman, India. Diamino diphenyl methane was purchased from Alfa Aesar, Germany.
2.2. Characterization
Fourier transform infrared (FT-IR) spectra were recorded in an ABB Bomem MB series FT-IR spectrometer and spectroscopy grade KBr was used to make pellets for analysis. Nuclear magnetic resonance (1H and 13C) measurements were carried out with a Bruker AV III spectrometer (500 MHz) (samples are slightly heated for complete soluble in deuterated solvents). DSC was performed on a Perkin Elmer DSC 7 model under air atmosphere. The samples were heated to 300 °C, then cooled to 25 °C and reheated to 300 °C at a heating rate of 5 °C min−1. Glass transition temperature was determined by Perkin Elmer DSC 7 in the temperature range of 30 to 300 °C. The mid-point between onset and endset of the inflectional tangent on the measured curve is denoted as glass transition temperature (Tg). The morphology of the cured cyanate ester and cyanate ester/epoxy blends were studied using a JEOL JSM-6460 scanning electron microscope and the samples were coated with gold (80%) and palladium (20%) alloy layer by Hummer VII sputtering system (Anatech Ltd, Alexandria, VA) under argon atmosphere to make them conductive. The dynamic mechanical properties of the cyanate ester/epoxy blends were obtained using a NETZSCH DMA 242 dynamic mechanical analyser in the tensile mode. Rectangular specimens (made up of stainless steel) of 55 mm length, 10 mm width and 3 mm thickness were used. Hot stage polarized optical microscopy (HOPM) studies were carried out by using Euromax polarizing optical microscope equipped with a Linkem HFS-91 heating stage and a TP-93 temperature programmer and the images were recorded using Nikon DSLR camera. Thermo gravimetric analysis (TGA) was done by using a Perkin Elmer Diamond series model in the range of 25–700 °C at a heating rate of 10 °C min−1 under flowing nitrogen or air.
2.3. Synthesis of diamine(1,4-bis(4-aminophenoxy)butane)
The procedure for the synthesis of 1,4-bis(4-acetamidophenoxy)butane and all the diamines were reported in our previous paper.12,19
2.4. Synthesis of bisphenols
2.4.1. Synthesis of 1,4-bis[4(2-hydroxyphenylazomethyl)phenoxy]butane (But-OH). 1,4-Bis(4-aminophenoxy)butane (2.750 g, 0.01 mol) and 100 mL of ethanol were taken in a 250 mL round bottomed flask and refluxed. To this 2.1 mL of 2-hydroxy benzaldehyde (0.0201 mol) was added drop wise with constant stirring. The reaction was initiated by adding trace amounts of trifluroacetic acid and refluxed for a further period of 6 h. Yellow crystals formed were separated by filtration. The bisphenol was washed with distilled water followed by cold ethanol and recrystallized using hot ethanol. Yield: 90%, M.P.: 145 °C. FT-IR (KBr, cm−1): 3392 (stretching vibrations of –OH), 2951 (stretching vibrations of –OCH2), 1688 (stretching vibrations of –HC
N–), 835 (bending vibrations of p-substituted aromatic). 1H-NMR (DMSO-d6, ppm): 9.4 (s, 2H, a), 7.0 (d, 2H, b), 7.5 (d, 2H, c), 7.1 (d, 2H, d), 7.7 (d, 2H, e), 8.9 (s, 4H, f), 7.2 (d, 4H, g), 6.9 (d, 4H, h), 4.1 (t, 4H, i), 1.9 (m, 4H, j). 13C-NMR (500 MHz, DMSO-d6, ppm): C1-161.0, C2-117.4, C3-132.9, C4-121.9, C5-132.3, C6-120.7, C7-159.9, C8-145.8, C9-122.3, C10-116.3, C11-155.8, C12-67.3, C13-27.8.
2.4.2. 1,5-Bis[4(2-hydroxyphenylazomethyl)phenoxy]pentane (Pen-OH). Yield: 86%, M.P.: 113 °C. FT-IR (KBr, cm−1): 3405 (stretching vibrations of –OH), 2949 (stretching vibrations of –OCH2–), 1680 (stretching vibrations of –HC
N–), 836 (bending vibrations of p-substituted aromatic). 1H-NMR (DMSO-d6, ppm): 9.3 (s, 2H, a), 7.1 (d, 2H, b), 7.4 (d, 2H, c), 7.0 (d, 2H, d), 7.6 (d, 2H, e), 8.9 (s, 4H, f), 7.2 (d, 4H, g), 6.9 (d, 4H, h), 4.1 (t, 4H, i), 1.9 (m, 4H, j), 1.8 (m, 2H, k). 13C-NMR (500 MHz, DMSO-d6, ppm): C1-161.0, C2-117.4, C3-132.9, C4-121.7, C5-132.1, C6-120.3, C7-159.9, C8-145.8, C9-122.3, C10-116.3, C11-155.8, C12-67.3, C13-27.8, C14-22.8.
2.4.3. 1,6-Bis[4(2-hydroxyphenylazomethyl)phenoxy]hexane (Hex-OH). Yield: 93%, M.P.: 141 °C. FT-IR (KBr, cm−1): 3341 (stretching vibrations of –OH), 2934 (stretching vibrations of –OCH2–), 1679 (stretching vibrations of –HC
N–) 839 (bending vibrations of p-substituted aromatic). 1H-NMR (DMSO-d6, ppm): 9.3 (s, 2H, a), 7.1 (d, 2H, b), 7.4 (d, 2H, c), 7.0 (d, 2H, d), 7.6 (d, 2H, e), 8.9 (s, 4H, f), 7.2 (d, 4H, g), 6.9 (d, 4H, h), 4.1 (t, 4H, i), 1.9 (m, 4H, j), 1.8 (m, 2H, k). 13C-NMR (500 MHz, DMSO-d6, ppm): C1-161.0, C2-117.4, C3-132.9, C4-121.6, C5-132.3, C6-120.6, C7-159.9, C8-145.8, C9-122.3, C10-116.3, C11-155.8, C12-67.3, C13-27.8, C14-25.8.
2.5. Synthesis of cyanate ester monomers
A 250 mL, three-necked round bottomed flask (maintained at −15 °C until completion of entire reaction), equipped with a magnetic stirring device and a nitrogen inlet was charged with a solution of 2.4 g (0.0228 mol) of CNBr in acetone (50 mL) and 3.5 g (0.0114 mol) of the bisphenol, 1,4-bis[4(2-hydroxyphenylazomethyl)phenoxy]butane (bisphenol was separately refluxed with acetone until it dissolved completely, cooled and added to CNBr in drop wise). To this mixture, 3.2 mL (0.0228 mol) of triethylamine was added in drops with continuous stirring. After complete addition of triethylamine, the reaction mixture was stirred for a further period of 2 h. The temperature of the system was then raised to room temperature. The mixture was poured into crushed ice when a brown precipitate (yield: 91%) was separated out. All other prepared bisphenols were converted into their corresponding dicyanate ester monomers in a similar way.
2.5.1. 1,4-Bis[4(2-cyanatophenylazomethyl)phenoxy]butane (But-CE). Yield: 92%, M.P.: 202 °C. FT-IR (KBr, cm−1): 2218, 2232 (stretching vibrations of –OCN), 2951 (stretching vibrations of OCH2), 1684 (stretching vibrations of –HC
N–), 834 (bending vibrations of p-substituted aromatic). 1H-NMR (DMSO-d6, ppm): 7.0 (d, 2H, a), 7.6 (d, 2H, b), 7.1 (d, 2H, c), 7.7 (d, 2H, d), 8.7 (s, 2H, e), 7.1 (d, 4H, f), 7.0 (d, 4H, g), 4.0 (t, 4H, h), 1.8 (m, 4H, i). 13C-NMR (500 MHz, DMSO-d6, ppm): C1-109.2, C2-154.7, C3-115.9, C4-133.4, C5-127.7, C6-133.4, C7-118.7, C8-160.1, C9-143.4, C10-122.5, C11-116.7, C12-156.2, C13-67.8, C14-28.6.
2.5.2. 1,5-Bis[4(2-cyanatophenylazomethyl)phenoxy]pentane (Pen-CE). Yield: 87%, M.P.: 159 °C. FT-IR (KBr, cm−1): 2192, 2228 (stretching vibrations of –OCN), 2945 (stretching vibrations of OCH2), 1682 (stretching vibrations of –HC
N–), 838 (bending vibrations of p-substituted aromatic). 1H-NMR (DMSO-d6, ppm): 7.1 (d, 2H, a), 7.6 (d, 2H, b), 7.0 (d, 2H, c), 7.7 (d, 2H, d), 8.9 (s, 2H, e), 7.1 (d, 4H, f), 7.0 (d, 4H, g), 4.1 (t, 4H, h), 1.9 (m, 4H, i), 1.7 (m, 2H, j). 13C-NMR (500 MHz, DMSO-d6, ppm): C1-109.1, C2-154.6, C3-115.8, C4-133.6, C5-127.6, C6-133.1, C7-118.7, C8-160.2, C9-143.4, C10-122.5, C11-116.7, C12-156.3, C13-66.9, C14-28.6, C15-22.1.
2.5.3. 1,6-Bis[4(2-cyanatophenylazomethyl)phenoxy]hexane (Hex-CE). Yield: 90%, M.P.: 183 °C. FT-IR (KBr, cm−1): 2209, 2222 (stretching vibrations of –OCN), 2938 (stretching vibrations of OCH2), 1686 (stretching vibrations of –HC
N–), 837 (bending vibrations of p-substituted aromatic). 1H-NMR (DMSO-d6, ppm): 7.1 (d, 2H, a), 7.6 (d, 2H, b), 7.1 (d, 2H, c), 7.7 (d, 2H, d), 8.6 (s, 2H, e), 7.1 (d, 4H, f), 7.0 (d, 4H, g), 4.1 (t, 4H, h), 1.9 (m, 4H, i), 1.7 (m, 2H, j). 13C-NMR (500 MHz, DMSO-d6, ppm): C1-109.3, C2-154.9, C3-116.0, C4-133.5, C5-127.6, C6-133.1, C7-118.7, C8-159.9, C9-143.4, C10-122.5, C11-116.7, C12-156.3, C13-67.3, C14-28.4, C15-22.3.
2.6. Preparation of cyanate ester/epoxy blends
Three different weight ratios of cyanate ester/epoxy blends were prepared in order to study the effect of increasing cyanate ester contents in epoxy blends. 2 g of dicyanate ester was taken in a 100 mL beaker, 18 g of epoxy resin was added and the mixture was heated on a hot plate at 175 °C with continuous stirring until it turned into a homogeneous solution. The temperature of the mixture was reduced to 75 °C once it became homogeneous. To this solution, 4.86 g of DDM20 (27 g for 100 g of epoxy resin) was added, stirred until it dissolved completely and then poured into a mold (made up of stainless steel and a dimension of 55 mm length, 10 mm width and 3 mm thickness). Cyanate/epoxy systems were cured by following the cure cycle: 120 °C/1 h + 150 °C/1 h + 180 °C/2 h + 220 °C/2 h. After the curing was complete, the samples were allowed to cool slowly in order to prevent cracking. The same procedure was adopted for the preparation of cyanate ester + epoxy blends with 5% and 10% of cyanate ester.
3. Results and discussion
Scheme 1 shows the preparation of cyanate ester. The aromatic diols were prepared from aromatic diamines and aldehyde by condensation reaction. The reaction was initiated by trace amounts of trifluroacetic acid. The bisphenols were converted into their corresponding dicyanate esters by reacting with cyanogen bromide in the presence of triethylamine. The structures of the bisphenols and dicyanate esters were confirmed by FT-IR and NMR (1H and 13C) spectral studies. The FT-IR spectra of all the three dicyanate esters are given in Fig. 1. The formation of azomethine linkages is confirmed by the appearance of new bands around 1680 cm−1 and disappearance of amine bands around 3375–3405 cm−1. The formation of –C–O–C linkage is confirmed by the presence of band at 1051 to 1075 cm−1 in the FT-IR spectrum. In the FT-IR spectra, the disappearance of amide band at 3336 cm−1 and appearance of the new band in the range of 3375–3405 cm−1 confirms the conversion of amide in to amine was succeed.19 The appearance of new signal at 8.9 ppm due to azomethine proton and disappearance of amine protons signal at 3.7 ppm indicate the successful conversion of the amino group to azomethine group.12,18 The appearance of new bands at 2192–2232 cm−1 and disappearance of hydroxyl band at 3392–3440 cm−1 in the FT-IR spectrum confirm the conversion of hydroxyl group into dicyanate ester. The conversion was further confirmed by the disappearance of the hydroxyl proton signal at 9.4 ppm and appearance of the signal due to cyanate ester carbon at 109.1 ppm in the 1H and 13C NMR spectra respectively.21 The chemical shifts of all the aromatic protons appear in the range of 7.0 to 7.6 ppm. The chemical shifts at 4.0 and 1.9 ppm are assigned to the methylene protons attached to aromatic ring through oxygen atom and the methylene protons of spacer group respectively. In the 13C-NMR spectrum, the peak in the range of 159–161 ppm may be assigned to carbon atom present in the azomethine linkage.18 Aromatic carbons appear in the range of 115.8–156.3 ppm. Fig. S1 and S2† represent the 1H-NMR and 13C-NMR spectra of the dicyanate ester (But-CE). Curing of cyanate ester and cyanate ester/epoxy blend was given in Scheme 2. In FT-IR spectra, the absorption peaks at 1371 cm−1 and 1748 cm−1 assigned to the presence of triazine and oxazolidinone rings respectively.5 Hence, the proposed structures of all the synthesised dicyanate esters were confirmed.
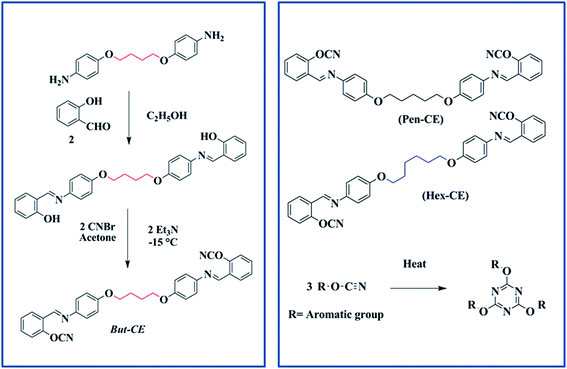 |
| Scheme 1 Synthesis of dicyanate ester monomer. | |
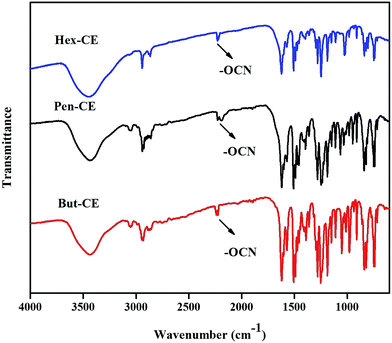 |
| Fig. 1 FT-IR spectrum of the dicyanate esters But-CE, Pen-CE and Hex-CE. | |
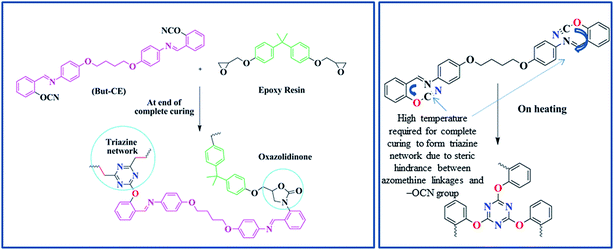 |
| Scheme 2 Curing of dicyanate ester monomer and cyanate ester/epoxy blend system. | |
3.1. Differential scanning calorimetry
The cure behavior of the dicyanate esters were studied using differential scanning calorimetry and FT-IR spectral studies. The DSC curves showing the melting and crystallization of the dicyanate esters But-CE, Pen-CE and Hex-CE are shown in Fig. 2 and the data are given in Table 1. The crystallization temperatures and melting points of the dicyanate esters were found to be in the range of 81.7 to 168 °C and 159 to 201.7 °C respectively. The melting points of these dicyanate esters are higher than that of commercially available dicyanate ester (BADCY, M.P.: 80 °C).22 The dicyanate esters But-CE and Hex-CE show sharp crystallization peaks compared to the dicyanate ester Pen-CE. The Pen-CE shows a broad crystallization peak and lowest crystallization temperature (81.7 °C) compared to other two dicyanate esters. The difference in temperature between melting point and crystallization temperature was found to be high for Pen-CE. The dicyanate ester monomers But-CE, Pen-CE and Hex-CE were found to cure in the temperature range of 255.7 to 293, 207.1 to 267.9 and 245.2 to 280 °C respectively. The dicyanate esters (But-CE and Hex-CE) having even number of carbon atoms in the alkyl spacer were found to have the highest Tmax (exothermic peak maximum temperature) at 277.7 and 252.48 °C respectively. The Pen-CE with odd number of carbon atoms in the alkyl spacer was found to have lowest Tmax (exothermic peak maximum temperature) at 229 °C. The odd and even number of carbon atom in alkyl spacer plays a major role in the curing temperature of dicyanate esters. Such an odd–even effect is well-known in the case of liquid crystalline polymers and was also seen in a recent study on the crystallization of biscarbamates.23 The sharp endotherm confirms that all the dicyanate ester is crystalline and it is further confirmed in polarized optical microscope. The crystallization DSC of all the three dicyanate esters was given in ESI.†
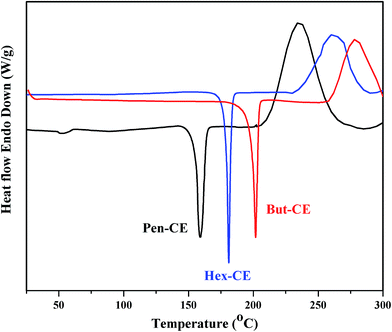 |
| Fig. 2 DSC thermogram of complete curing of the dicyanate esters. | |
Table 1 DSC data of the cyanate ester
Sample code |
M.P. (°C) |
Crystallization (°C) |
Ti (°C) |
Tmax (°C) |
Tend (°C) |
ΔH |
But-CE |
201.7 |
168.0 |
255.7 |
277.7 |
293.0 |
37.3 |
Pen-CE |
159.0 |
81.70 |
207.1 |
229.0 |
267.9 |
60.8 |
Hex-CE |
181.1 |
143.3 |
245.2 |
252.48 |
280.0 |
34.8 |
3.2. Thermogravimetric analysis
The thermal stability of polymeric materials is a very essential characteristic for them to be used for flame retardant applications. It is mainly concerned with the release of decomposition products and the formation of char. The thermal stability of the cured cyanate esters and cyanate ester/epoxy blends were evaluated and expressed in terms of its 5% weight loss temperatures (T5%), 10% weight loss temperatures (T10%) and the char yield at 700 °C. The TGA curves of various cured dicyanate esters are given in Fig. 3 and the data are given in Table 2. On heating, neat cured dicyanate esters show 5% weight loss temperature (T5%) in the range of 415 to 467 °C. T5% was found to be low (415 °C) for cyanate ester (Pen-CE) having alkyl chain length of 5 (odd number) between two aromatic groups. The But-CE having lowest spacer length showed the highest T5% temperature of 467 °C. T5% of commercially available BADCY (420 °C) was found to be higher than that of Pen-CE (414.94 °C). Char yield of 70.78 to 73.12% was obtained at end of the analysis at (700 °C). The prepared dicyanate esters show better thermal stability and char yield compared to commercially available dicyanate ester (char yield of BADCY is found to be 49% at 650 °C).22 The cyanate ester (But-CE) has better char yield compared to other two cyanate esters. The dicyanate esters having even number of carbon atoms in the spacer show better char yield than those having odd number of carbon atoms in the spacer between two aromatic rings.12 The T5% and T10% of cyanate ester/epoxy blends were found to be in the range of 399 to 410 °C and 422 to 445 °C respectively. Char yield of the cyanate ester/epoxy blends were found to be in the range of 34.75 to 42.65 (at 700 °C and the analysis was stopped at 700 °C due to sticking of blends in crucible and its leads to replacement of the crucible). The But-CE and its epoxy blends show better thermal stability and char yield percentage. The prepared cyanate esters and their epoxy blends were found to have a very good thermal stability compared to the terpolymer benzoxazine–cyanate–epoxy system having T10% at 328 °C.5 The char yield and LOI of neat epoxy resin were found to be 28.0% and 28.7% respectively (at 700 °C). The char yield and LOI of the epoxy blends increase with increase in cyanate ester content in epoxy blends, which indicates that the cyanate ester enhances the thermal properties of epoxy resin. As per Krevelen's statement minimum threshold value of 26 is required for the polymer to behave as a self-extinguishing material. It is evident that the neat epoxy blends cannot serve as self-extinguishing materials. But the CE/epoxy blends show better thermal stability compared to neat epoxy resins.
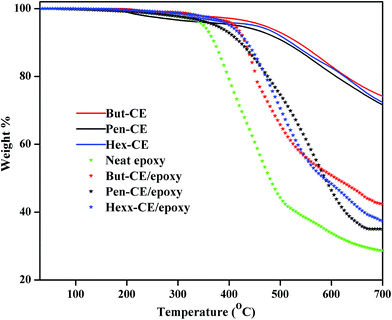 |
| Fig. 3 TGA thermogram data of cyanate ester. | |
Table 2 TGA data of polycyanurates
Sample code |
T5% (°C) |
T10% (°C) |
Char yield% |
LOI |
But-CE |
467.12 |
529.94 |
73.12 |
46.74 |
Pen-CE |
414.94 |
509.14 |
70.78 |
45.81 |
Hex-CE |
449.94 |
519.24 |
72.12 |
46.34 |
Neat epoxy |
363.30 |
369.11 |
28.0 |
28.7 |
10% But-CE + 90% epoxy |
409.82 |
445.21 |
42.65 |
34.56 |
10% Pen-CE + 90% epoxy |
398.77 |
422.39 |
34.75 |
31.40 |
10% Hex-CE + 90% epoxy |
403.22 |
434.67 |
37.43 |
32.47 |
3.3. Morphology studies
SEM analysis was conducted to investigate the dispersion of cyanate ester in the epoxy blends. The fracture surfaces of the samples were sputtered with gold to deionize the electrons on the surface. SEM images of cured But-CE, Pen-CE, Hex-CE, 5% But-CE blend (5% cyanate ester + 95% epoxy) and 10% But-CE blend (10% cyanate ester + 90% epoxy) are given in Fig. 4a–e respectively. The fractured surface of the But-CE (Fig. 4a) shows rose-like layered structure and its higher magnification shows rose-petals like morphology. Fractured surface of cured cyanate esters Pen-CE (Fig. 4b) and Hex-CE (Fig. 4c) were look like rock and layered structure respectively. The SEM images of neat cyanate ester (Hex-CE) resembles that of 2,2′-bis(4-cyanatophenyl)isopropylidene reported by J. T. Hu et al.14 These observations indicate a brittle behavior for all the three cured neat cyanate ester resins as expected a thermosetting resins. SEM images c and d illustrate uniform distribution of the cyanate ester in the epoxy blends without any void.
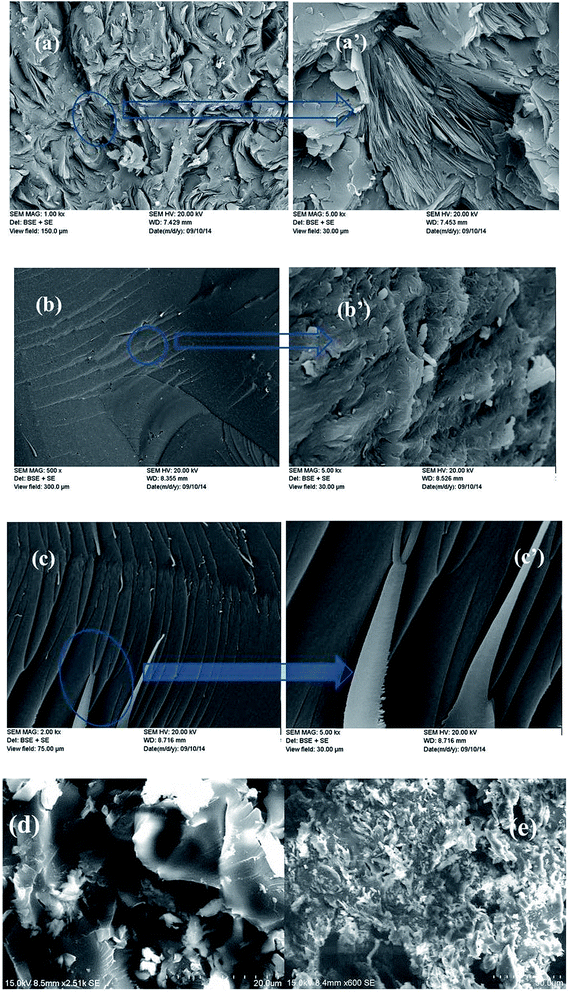 |
| Fig. 4 SEM images of cured (a) neat But-CE, (b) neat Pen-CE and (c) neat Hex-CE (d) 5% But-CE + 95% epoxy system, (e) 10% But-CE + 90% epoxy system. | |
3.4. Dynamic mechanical analysis (DMA)
The effects of increase of cyanate ester content on the stress relaxation behavior of the CE/epoxy blends have been studied. The storage modulus and tan delta of the blend with 10% cyanate ester (But-CE, Pen-CE and Hex-CE) are given in Fig. 5 and 6 respectively. The data obtained from DMA scans are given in Table 3. Both the storage modulus and glass transition temperature of the CE/epoxy blends decrease with increase in the cyanate ester content (from 1 to 10% of cyanate ester).
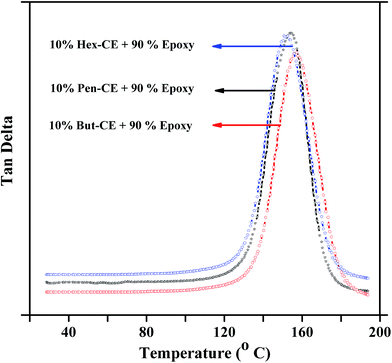 |
| Fig. 5 DMA curve (tan delta vs. temperature) of the CE/epoxy blends. | |
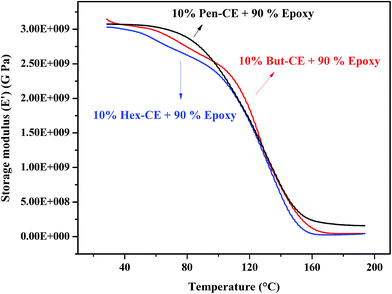 |
| Fig. 6 DMA analysis showing storage modulus of the CE/epoxy blends. | |
Table 3 Values of crosslink density of CE + epoxy blends (from DMA values)
Sample code |
CE% |
E′ (GPa) |
Temp. k (273 + 75) |
Cross link density νe × 105 (mol m−3) |
But-CE + epoxy |
1 |
2.96 |
348 |
3.41 |
Pen-CE + epoxy |
1 |
3.01 |
348 |
3.46 |
Hex-CE + epoxy |
1 |
2.91 |
348 |
3.35 |
But-CE + epoxy |
10 |
2.75 |
348 |
3.16 |
Pen-CE + epoxy |
10 |
2.92 |
348 |
3.36 |
Hex-CE + epoxy |
10 |
2.68 |
348 |
3.08 |
The But-CE/blend with 10 weight% cyanate ester, displays a storage modulus of 2.75 GPa at 75 °C. The glass transition temperature can be determined as the temperature at which E′ exhibits a steep decrease and as well as the temperature of peak maximum of the tan
δ curve.
The neat cured epoxy has a remarkable Tg (161 °C)21 compared to other epoxies reported. The incorporation of 1 wt% Hex-CE into epoxy blends diminishes the Tg from 161 to 160 °C. But, on addition of 5% Hex-CE to the epoxy resin, the glass transition temperature was decreased to 156 °C from 161 °C) and in the case of 10% Hex-CE in the epoxy resin, the glass transition temperature was decreased to 151 °C from 161 °C.
Increasing the amount of cyanate ester in the blend decreases the storage modulus due to aliphatic chains as bridging groups. Also the presence of cyanate ester functionality in the ortho position to the azomethine leads to unsymmetrical arrangement of the polymer chain segments leading to a decrease in the glass transition temperature of CE/epoxy blend. The lower cross link density of the blends in comparison to neat epoxy resins can also be another cause for the decrease in glass transition temperature. The glass transition temperature of the epoxy resins decrease with increase in cyanate ester content.21
3.5. Crosslink density
The number of moles of network chains per unit volume of the cured polymers is described as cross link density. Crosslink density of highly crosslinked polymers can be measured by substituting modulus in the rubbery plateau, in the equation of state for rubber elasticity24 as
where, E′ = tensile storage modulus in the rubbery plateau, T = temperature in K corresponding to the storage modulus value, R = gas constant.
Table 3 shows the crosslink density of the CE/epoxy blends. On addition of cyanate ester to the epoxy resin, the cross link density was decreased. The storage modulus of the neat cured epoxy resin was found to be 5.69 GPa at 25 °C25 and the storage modulus was decreased to 3.22 GPa at 25 °C on the addition of 10% But-CE in to epoxy resins (comparison table was given in ESI†). The Hex-CE/epoxy blend shows the lowest cross link density compared to the other two cyanate ester/epoxy blends. The cyanate ester functionality being in the ortho position to the azomethine group, the approach of the –OCN of three such molecules will the very difficult there by decreasing the crosslink density. The presence of C–C single bonds as the bridging groups in the dicyanate monomers makes the approach of the three cyanate ester groups to form symmetric triazine rings, less favorable due to entropy factor because of free rotations about the C–C single bond. Usually commercially available cured epoxy resin (DGEBA) show very high crosslink density compared to other resins. The Tg of the blends decreases with decrease in cross-link density of the blends and similar observations are reported by other authors.26
3.6. Flame-retardant properties of the cured dicyanate ester/epoxy blends
The flame résistance of cyanate ester and cyanate ester/epoxy blends are expressed in terms of limiting oxygen index (LOI). LOI is defined as the minimum fraction of oxygen in a mixture of O2 and N2 during fire. The LOI value should be higher than that of threshold value (value 26) for the polymer to behave as a self-extinguishing material or self-extinguisher. The LOI value is calculated by using van Krevelen's equation.27
where σ is the percentage of char yield. The LOI of the various cured dicyanate ester and cyanate ester/epoxy blends were found to be in the range of 45.81 to 46.74 and 31.40 to 34.56 respectively. The cured dicyanate ester with shorter alkyl spacer shows the highest LOI value and the cured dicyanate ester having five carbon atoms in alkyl chain spacer shows the lowest LOI value. The LOI values of all the cured dicyanate esters indicate that they are very good fire retardant materials. From the LOI values, it was found that the cured cyanate ester But-CE/epoxy blends show the best flame retardance.
3.7. Optical studies
The polarized optical microscope studies were performed for all the neat dicyanate esters. Small quantity of the material was taken between two thin glass cover slips, heating and cooling was carried out at the rate of 5 °C min−1. The polarized optical microscope images of the dicyanate ester monomers (during cooling, taken different positions of sample at various temperatures) are given in Fig. 7. The pictures clearly indicate that on cooling the molten monomers, crystallization takes place proving their crystalline nature.
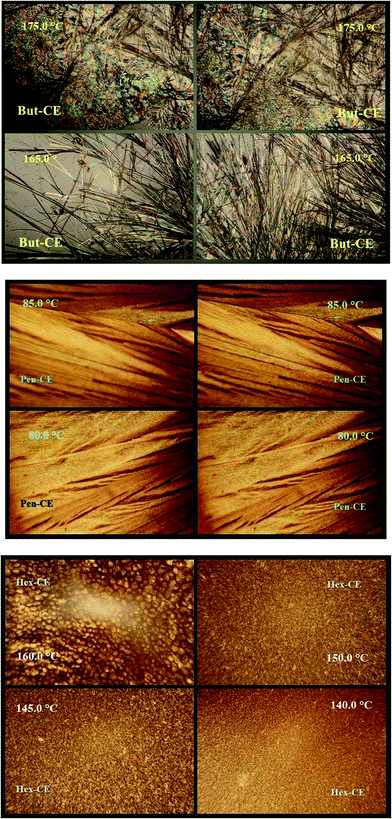 |
| Fig. 7 POM images of cyanate esters But-CE, Pen-CE and Hex-CE. | |
4. Conclusion
The cyanate ester group present in the para position to azomethine linkage show melting point and curing temperature in the range of 91 to 123 and 203 to 242 °C respectively.12 The maximum curing temperature of ortho substituted dicyanate esters were found to be in the range of 229–278 °C. The presence of the cyanate group (–OCN) in ortho position to azomethine linkages show steric hindrance between two groups which leads to increase in curing temperature of the ortho substituted dicyanate ester. All the prepared dicyanate esters were found to be crystalline in nature as indicated by DSC analysis and the crystallization process of dicyanate ester having odd number of carbons in the alkyl chain spacer between two aromatic ring (Pen-CE) is slower compared to dicyanate ester having even number of carbons in the alkyl spacer (Ben-CE and Hex-CE). They lose the crystallinity after trimerization. Due to the crystalline behavior of the dicyanate ester monomer, the cure behavior was affected. The para substituted dicyanate ester does not show the crystalline nature in both DSC and POM studies.12 Similar observations such as thermal properties were found in the thermo tropic polyesters.28
The CE/epoxy blends have uniform distribution of the cyanate ester in the epoxy resin. The DMA study reveals the glass transition temperature of epoxy was decreased from 161 to 151 °C on adding 10% of Hex-CE in the epoxy resin. The crosslink density of the cyanate epoxy blends decreases from 3.35 to 3.08 mol m−3 for Hex-CE with increase in the weight ratio of CE (1–10%) in the blend. The storage modulus of the epoxy resin decreases with increase in cyanate ester content. TGA results show that the char yield of neat cured dicyanate esters are in the range of 70.78 to 73.12. The char yield increases with increase of But-CE content in the CE/epoxy blend from 28% (neat epoxy) to 42.65% (for 10% CE/epoxy) due the formation of the oxazolidinone structure between epoxy resin and dicyanate ester which results in enhance thermal properties of cyanate/epoxy blends.5,12
The char yield of But-CE found to be high for TGA analysis was carried out in nitrogen atmosphere (73.12%) compared to analysis carried out in air atmosphere (64.38%).29 The thermal properties such as Tmax, T10% and Tg were found to be high for But-CE system compared to other two dicyanate ester and their blends. This increase in char yield on the addition of cyanate ester on epoxy blends further suggests that the CE/epoxy blends can be used for high flame retardancy applications, even though they have lower mechanical properties than epoxy resin. The effect of microwave irradiation on morphology, the time and temperature for complete curing between microwave curing and conventional curing shall be studied in the future.
Acknowledgements
This work was supported by University Grants Commission (UGC-BRS), India and Natural Sciences and Engineering Research Council of Canada (NSERC). The authors also acknowledge DST (FIST) and UGC (SAP) for the financial support extended to the department to procure instrumental (TGA) facilities.
References
- Y. Zhao, Z. K. Chen, Y. Liu, H. M. Xiao, Q. P. Feng and S. Y. Fu, Composites, Part A, 2013, 55, 178–187 CrossRef CAS PubMed.
- M. H. Gabr, N. T. Phong, K. Okubo, K. Uzawa, I. Kimpara and T. Fujii, Polym. Test., 2014, 37, 51–58 CrossRef CAS PubMed.
- B. Qi, Q. X. Zhang, M. Bannister and Y. W. Mai, Compos. Struct., 2006, 75, 514–519 CrossRef PubMed.
- W. G. Kim and T. Y. Nam, J. Polym. Sci., Part A: Polym. Chem., 1996, 34, 957–962 CrossRef CAS.
- X. Li, Y. Xia, W. Xu, Q. Ran and Y. Gu, Polym. Chem., 2012, 3, 1629–1633 RSC.
- S. Levchik, A. Piotrowski, E. Weil and Q. Yao, Polym. Degrad. Stab., 2005, 88, 57–62 CrossRef CAS PubMed.
- C. S. Wang and C. H. Liu, Polymer, 2000, 41, 8579–8586 CrossRef CAS.
- R. Biju, C. Gouri and C. P. Reghunadhan Nair, Eur. Polym. J., 2012, 48, 499–511 CrossRef CAS PubMed.
- C. H. Lin, C. N. Hsiao, C. H. Li and C. S. Wang, J. Polym. Sci., Part A: Polym. Chem., 2004, 42, 3986–3995 CrossRef CAS PubMed.
- J. J. Cash, M. C. Davis, M. D. Ford, T. J. Groshens, A. J. Guenthner, B. G. Harvey, K. R. Lamison, J. M. Mabry, H. A. Meylemans, J. T. Reams and C. M. Sahagun, Polym. Chem., 2013, 4, 3859–3865 RSC.
- S. Rakesh, C. P. Sakthidharan, M. Sarojadevi and P. R. Sundararajan, Eur. Polym. J., 2015, 68, 161–174 CrossRef CAS PubMed.
- C. P. Sakthidharan, P. R. Sundararajan and M. Sarojadevi, RSC Adv., 2015, 5, 19666–19674 RSC.
- I. Hamerton, B. J. Howlin, S. L. Jewell and P. Patel, React. Funct. Polym., 2012, 72, 279–286 CrossRef CAS PubMed.
- J. T. Hu, A. Gu, G. Liang, D. Zhuo and L. Yuan, J. Appl. Polym. Sci., 2012, 126, 205–215 CrossRef CAS PubMed.
- E. M. Woo, D. A. Shimp and J. C. Seferis, Polymer, 1993, 35, 1658–1665 CrossRef.
- S. Gürşen, S. Yildirim and H. Deligöz, Polym. Eng. Sci., 2013, 53, 662–670 Search PubMed.
- A. T. DiBenedetto, J. Polym. Sci., Part B: Polym. Phys., 1987, 25, 1949–1969 CrossRef CAS PubMed.
- A. Ganesan and S. Muthusamy, Polym. Compos., 2009, 30, 782–790 CrossRef CAS PubMed.
- P. A. Henderson, R. T. Inkster, M. Seddon and C. T. Imrie, J. Mater. Chem., 2001, 11, 2722–2731 RSC.
- M.-D. Shau and T.-S. Wanc, J. Polym. Sci., Part A: Polym. Chem., 1996, 34, 387–396 CrossRef CAS.
- S. Rakesh, C. S. Dharan, M. Selladurai, V. Sudha, P. Sundararajan and M. Sarojadevi, High Perform. Polym., 2012, 25, 87–96 CrossRef PubMed.
- C. H. Lin, Polymer, 2004, 45, 7911–7926 CrossRef CAS PubMed.
- M. K. Khan and P. R. Sundararajan, J. Phys. Chem. B, 2011, 115, 8696–8706 CrossRef CAS PubMed.
- L. W. Hill, Prog. Org. Coat., 1997, 31, 235–243 CrossRef CAS.
- Z. S. Pour and M. Ghaemy, Prog. Org. Coat., 2014, 77, 1316–1324 CrossRef CAS PubMed.
- B. Francis, V. L. Rao, G. Vanden Poel, F. Posada, G. Groeninckx, R. Ramaswamy and S. Thomas, Polymer, 2006, 47, 5411–5419 CrossRef CAS PubMed.
- D. W. van Krevelen, Polymer, 1975, 16, 615–620 CrossRef CAS.
- A. Blumstein and O. Thomas, Macromolecules, 1982, 15, 1264–1267 CrossRef CAS.
- C. S. Wang and M. C. Lee, Polymer, 2000, 41, 3631–3638 CrossRef CAS.
Footnote |
† Electronic supplementary information (ESI) available. See DOI: 10.1039/c5ra14396a |
|
This journal is © The Royal Society of Chemistry 2015 |