DOI:
10.1039/C5RA11144G
(Paper)
RSC Adv., 2015,
5, 73373-73380
Stereoselective synthesis of functionalized 1,2,3,4-tetrahydroisoquinolines (THIQs) via highly diastereoselective Ugi three-component reactions (U3CRs) with chiral 3,4-dihydroisoquinolines (DHIQs)†
Received
11th June 2015
, Accepted 19th August 2015
First published on 20th August 2015
Abstract
A highly diastereoselective Ugi three-component reaction (U3CR) involving chiral 3,4-dihydroisoquinolines (DHIQs), isocyanides and carboxylic acids has been developed to synthesize enantiopure 1,2,3,4-tetrahydroisoquinolines (THIQs). The inherent chirality of DHIQ at C-3 and C-4 controls the addition of isocyanide at C-1 to induce excellent diastereoselectivity. The method was applied to a variety of aromatic and aliphatic acids, and in most cases the reaction proceeded smoothly without formation of any side products. The experimental and computational studies demonstrated the role of internal chirality in the conformational stability of the intermediate in order to control the facial selectivity and strong solvent effect on the reaction. Cytotoxicity of selected compounds was also evaluated against four types of human cancer cell lines, MCF-7 (breast cancer), MDA MB-231 (breast cancer), DU-145 (prostate cancer), A549 (lung cancer) and HepG2 (liver cancer), where few compounds exhibited GI50 values in the submicromolar range.
Introduction
Multicomponent reactions (MCRs) allow for the rapid construction of small molecule libraries from common starting materials by combining economic and environmental aspects in a single step.1 The Ugi reaction is one of the most widely explored MCRs where an isocyanide reacts with in situ generated iminium ion to produce interesting peptidomimetic compounds.2 Various internal imine-containing substrates such as dihydroquinoline, dihydroisoquinoline and dihydrobenzoazepines have been successfully employed by Ugi three component reactions (U3CRs) to obtain functionalized heterocycles.3,4 Aromatic imines such as quinolines, isoquinolines or pyridines are not reactive towards isocyanide based MCRs, except for 1,3-dipolar cycloadditions with aldehydes, malononitrile, and isocyanides.5 One of the major drawbacks of the Ugi reaction is poor stereoselectivity during the isocyanide addition to iminium ion. Although many efforts have been made to develop enantio-/diastereoselective Ugi reactions, excellent selectivity was observed only in a few cases.6 While good diastereoselectivity remains challenging in open systems, excellent selectivity was observed when chiral cyclic imines were subjected to U3CRs reflecting strong stereo induction from inherent chirality.7–9 Particularly in the case of chiral pyrrolines, a strong stereoinduction was observed in the U3CR.10 In a computational study, Codee et al. found that the conformation of the iminium intermediate is determined by its substitution pattern, controlling the selectivity in the isocyanide addition to dictate the chirality at the new center.11 A combination of biocatalytic desymmetrization of chiral cyclic imines using the MCR has been developed by several groups for the stereoselective synthesis of substituted pyrrolidines.12 Furman et al. synthesized a series of polyhydroxylated piperidines and pyrrolidines by employing sequential lactam reduction/Joullie–Ugi reactions of their lactam counterpart.13 The use of chiral acids to attain the diastereoselectivity did not show encouraging results.14 Though the U3CR with various chiral cyclic imines have been studied, a diastereoselective U3CR with chiral 3,4-dihydroisoquinoline has not been reported to the best our knowledge.
1,2,3,4-Tetrahydroisoquinoline (THIQ) fused piperazines are widely found in many classes of alkaloids such as ecteinascidin (I),15 saframycin (II),16 naphthyridinomycin (III), 17 and quinocarcin (IV) 18 (Fig. 1). Though the 1,3-cis configuration is the most common in naturally occurring THIQ-containing alkaloids,19 epimers of naturally occurring counterparts such as 3-epi-jorumycin (V) and 3-epi-renieramycin G (VI) have been of significant synthetic interest to explore the role of stereochemistry in relation to their pharmaceutical properties.20
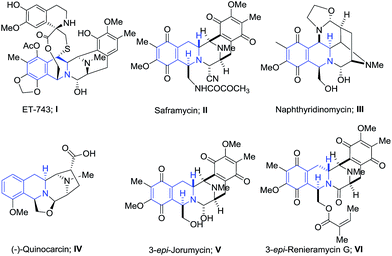 |
| Fig. 1 Important alkaloids with a chiral THIQ skeleton. | |
Generally the advanced synthetic intermediate of these complex alkaloids consists of a suitably substituted 1,2,3,4-tetrahydroisoquinoline (THIQ) skeleton with the requisite chirality.21 Therefore the asymmetric synthesis of functionalized THIQ has attracted synthetic chemists to develop newer methods.22 Recently, we have developed a novel method to synthesize 1,2,3,4-THIQ 3 from 3,4-dihydroisoquinoline 1 via a bridged oxazolidine intermediate 2 by employing Lewis acid catalyzed diastereoselective nucleophilic substitution reactions (eqn (1)).23 This offered an excellent method to obtain C-1 functionalized THIQs, however substitutions at N-2 were limited and required protection–deprotection operations for further applications for the total synthesis.23
|
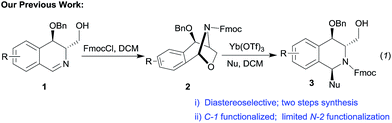 | (1) |
|
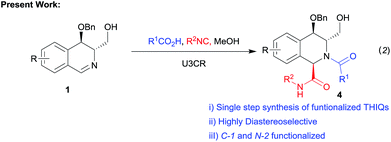 | (2) |
In order to develop an efficient synthesis of THIQ containing alkaloids, various functionalizations at N-2 are required with diastereoselective derivatization at C-1. We envisioned that the inherent chirality of C-3 and C-4 may significantly induce chirality at C-1 leading to the desired diastereoselectivity under U3CR conditions (eqn (2)). Herein, we report highly diastereoselective Ugi three-component reactions of DHIQ 1 to synthesise a variety of enantiopure THIQs 4 and to introduce a wide range of substitutions at N-2. Detailed mechanistic investigations including computational studies were performed and the results are summarised. In addition, functionalization of one Ugi product and the anti-proliferative properties of selected Ugi products have been evaluated.
Results and discussion
DHIQ 1 was easily obtained from Garner’s aldehyde in three steps by employing our reported procedure.23 A trial U3CR involving DHIQ 1, tert-butyl isocyanide and p-nitrobenzoic acid in methanol did not show any progress at lower temperatures (−40–0 °C), however a clean formation of product 5a was observed at room temperature (Table 1, entry 1–4). It was interesting to note that the reaction was highly dependent on the solvents and in toluene no characterizable product was observed following consumption of all the starting material. Formation of desired product was confirmed by 1H NMR analysis where the C-1-H at the newly generated stereogenic center was visible at δ 5.28 ppm and the C-1-H (imine proton) signal of DHIQ 1 disappeared from δ 8.32 ppm. The 1H NMR of purified 5a showed two signals for the C-1-H at δ 5.28 (major) and 5.17 (minor) corresponding to the two rotamers that was further confirmed by NOE experiments, while the two signals for the C-1-H at δ 5.28 (major) and 5.79 (minor) in the 1H NMR of the post aqueous work-up sample (crude sample) corresponds to the two diastereomers (C-1 epimers) [see the ESI†].
Table 1 Reaction optimization
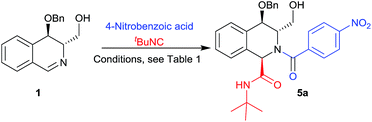
|
Entry |
Solvent |
Temp |
Time |
Yielda (%) |
Isolated yield of the major diastereomer 5a. Progress of the reaction was monitored by TLC. Starting material (SM) consumed but no characterizable product obtained. NR – no reaction, SM recovered. |
1 |
MeOH |
−40 °C |
20 h |
NR |
2 |
MeOH |
−20 °C |
20 h |
NR |
3 |
MeOH |
0 °C |
20 h |
NR |
4 |
MeOH |
rt |
15 min |
89 |
5 |
EtOAc |
rt |
15 min |
5 |
6 |
MeCN |
rt |
15 min |
27 |
7 |
THF |
rt |
15 min |
11 |
8 |
DMF |
rt |
15 min |
26 |
9 |
Toluene |
rt |
20 h |
0b |
Stereochemistry of 5a at the C-1 position was established by performing 2D-COSY and 2D-NOESY experiments which confirmed it to be 1 R, possibly generated through the addition of tert-butyl isocyanide from the Si face of C-1 (β-attack) (Fig. 2). The 1H NMR spectrum of 5a displayed two sets of resonances with a 16
:
1 ratio that indicates the presence of a minor isomer which was confirmed to be a rotamer due to the amide bond present at piperidine ring that could lead to two sets of resonances in the 1H NMR spectrum. These resonances showed exchange cross peaks between the minor and major peaks in the NOESY spectrum which confirms that the second set of resonances are appearing because of a rotational isomerization.24
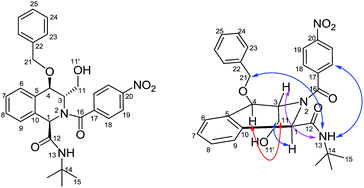 |
| Fig. 2 NOE correlations of compound 5a. | |
The appearance of strong NOE cross peaks between H-1/H-11 and H-1/H-11′ along with the small coupling constant between H-3 and H-4 (3J H-3–H-4 = 2.8 Hz) indicate that the piperidine ring adopts a half chair conformation having H-1, H-11(H-11′) and H-4 protons on one side of the ring as pictorially represented in Fig. 2.
To further check the scope of this reaction a series of aromatic, aliphatic and amino acid derivatives were subjected to the Ugi reaction with DHIQ 1 (Fig. 4). The diastereoisomeric ratios were confirmed by 1H NMR analysis of post aqueous work-up crude samples. All the products were easily purified by column chromatography and were characterized further by 1H and 13C NMR, mass, IR and HRMS analysis. Relatively poor yields were observed when cinnamic acid and aliphatic acids were used for the U3CR (5f and 5k, Fig. 3). Reactions with DHIQs 6, 7 and 8, bearing electron withdrawing or electron donating groups on the aromatic portion of DHIQ, yielded 5q, 5r and 5s respectively in good yields and high diastereoselectivity (Fig. 3 and 4).23
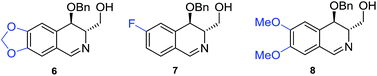 |
| Fig. 3 DHIQs used in the study with substitutions at the aromatic portion. | |
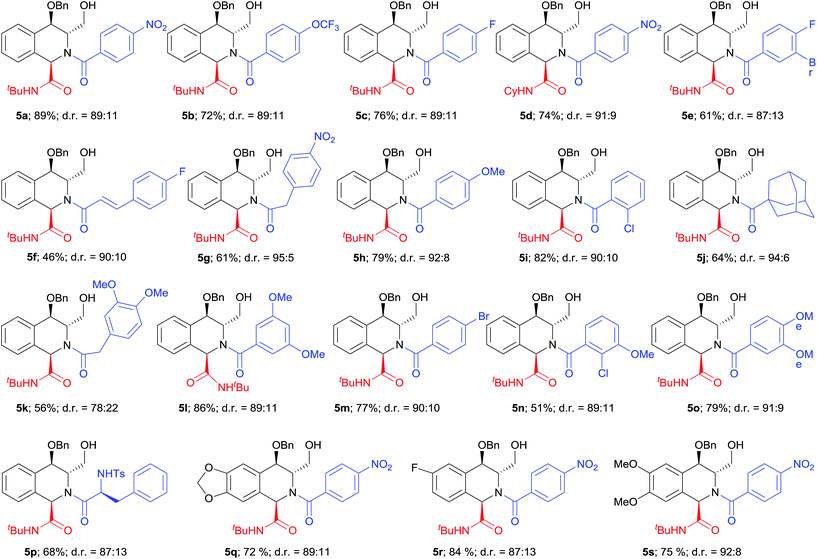 |
| Fig. 4 Substrate scope of the reaction [diastereomeric ratios were determined by 1H NMR analysis of post aqueous work-up crude reaction mixtures. The C-1-H at the newly generated stereogenic carbon was used to calculate the ratio as it was clearly visible in the region of δ 5.00–6.00 ppm without any overlap of peaks from excess reactants (see ESI†)]. | |
According to our hypothesis, it was important to understand the role of C-3-CH2OH which might be to induce the diastereoselectivity by providing anchimeric assistance. To examine the role of C-3-CH2OH on the stereochemical outcome, the U3CR with substrate 9, where OH was protected as a silyl ether, was carried out with similar reagents and conditions (Scheme 1). The protection of the alcohol functionality was difficult with other protecting groups, such as benzyl, benzoyl, etc., as DHIQ 1 gets converted to bridged oxazolidines.23 The Ugi reaction of substrate 9 yielded 10 with equally good diastereoselectivity as unprotected DHIQ 1 confirming that C-3-CH2OH did not participate in the reaction through anchimeric assistance by forming a bridged oxazolidine.23
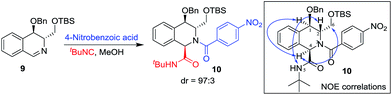 |
| Scheme 1 U3CR with a C3-hydroxymethyl protected DHIQ 9. | |
Another effort to extend this reaction to acyclic substrates with a chiral appendage at the C-2 position did not give encouraging results (Scheme 2). The Ugi reaction with an acyclic imine 12 which was obtained by the LAH reduction of L-phenylalanine 11 followed by condensation with p-toualdehyde gave equal mixtures of diastereomers 13, limiting the scope of the present methodology to cyclic substrates (Scheme 2, data not shown).
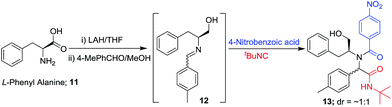 |
| Scheme 2 U3CR with an acyclic imine 12. | |
The effect of the C-4 chirality was also investigated by performing the reaction with syn-DHIQ; syn-1. The reaction gave good diastereoselectivity towards the β-product 14 (Scheme 3). The 1,3-trans configuration was confirmed by 2D-NOESY correlations of 14.
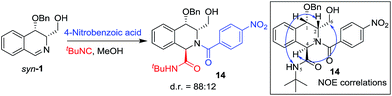 |
| Scheme 3 U3CR with syn-DHIQ (syn-1). | |
Computational studies
To understand the diastereoselective preference of the β (1R) isomer over the α (1S) isomer quantum chemical calculations were performed and the reaction profiles were generated by employing gas as well as solvent (methanol) phases using a PCM model at the B3LYP/6-31+G(d) level of theory for both α and β attacks. All the calculations were performed using the Gaussian 09 program package.25
Previous reports show that π-facial selectivity involves various factors such as steric, electrostatic and electronic which work in concordance and discordance in determining the final product.26 The bulky groups were substituted with methyl groups to reduce the computational cost. A huge effect of the solvent was observed on the reaction path (Fig. S1, see ESI†). Hence the discussion is carried out by analysing the solvent phase results (Fig. 5). The mechanism of the Ugi reaction has been well established previously, therefore we aim to look at the preferential formation of one isomer over the other. The initial trials for generating a N–C bond forming TS, an O–C bond breaking TS and H-migration TSs were futile. Hence we considered two explicit solvent molecules to generate these TSs. This method was previously employed by Fleurat-Lessard and co-workers.27 In3 complexes with the two methanol molecules and forms In4. All the trials for generating the C–N bond forming TS and the corresponding intermediate from In4 were futile which led either to In4 or In5. Therefore bond critical points (AIM2000 software)28 were generated for In4 to understand the nature of bonding (Fig. 6). The AIM calculations on the In4 suggest a bond critical point (BCP) between C⋯N, with ρ and its Laplacian values being 0.050 and 0.023 respectively, thereby suggesting that strong interactions exist between C⋯N that in turn yield a barrierless TS. This is true for the β attack while for the α attack In4 does not show a BCP between C⋯N. Thus In4 should generate In5 via a barrierless N–C bond forming TS and TS3 which is a proton migration of TS.
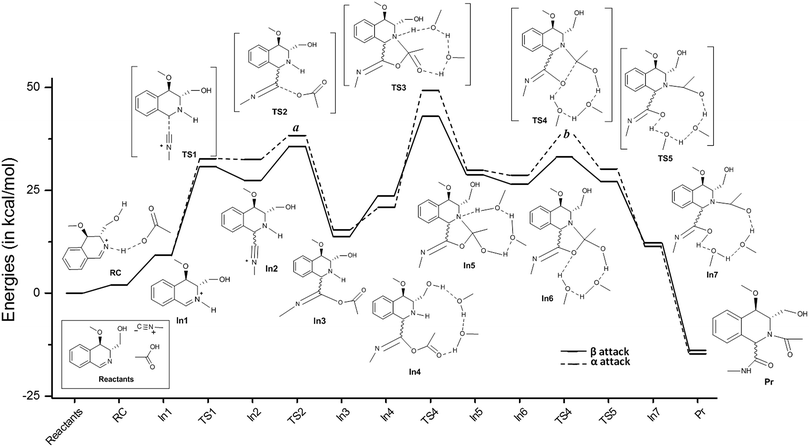 |
| Fig. 5 Reaction energy profiles generated at the B3LYP/6-31+G(d) level of theory (obtained by single point calculations using (a): gas phase optimized geometries, and (b): could not be obtained). | |
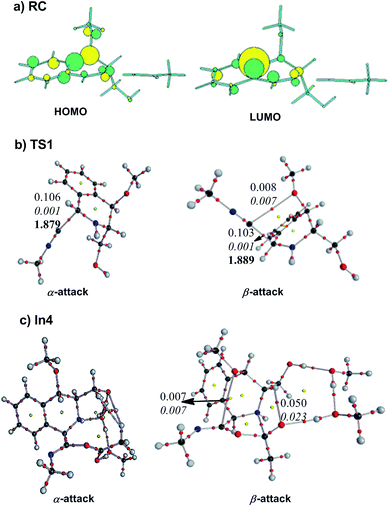 |
| Fig. 6 HOMO and LUMO orbital diagrams for RC along with bond critical points generated for TS1 and In4 considering α and β attacks (normal: ρ, italics: Laplacian of ρ, bold: bond lengths in Å). | |
After generating the reaction profiles for α and β attacks, we aimed to comprehend the reason for the experimentally formed β isomer as a major product. Throughout the reaction profile the structures generated for a β attack are stable compared to an α attack by ∼1 to 6 kcal mol−1 except for In4 and In8. Assuming that the initial attack of the isocyanide must be a deciding factor for the reaction to proceed forward and in turn generate the final product we focussed on the RC and TS1 structures. TS1 for a β attack is ∼2.0 kcal mol−1 more preferred than an α attack. This however does not give a valid reason for the preferential formation of the β product. Hence the HOMO and LUMO orbital diagrams of the RC were studied (Fig. 6). In both HOMO and LUMO the electron density on the orbitals is more concentrated towards the β phase suggesting that the isocyanide must attack from the β side. Further bond critical points were generated for TS1 considering both α and β attacks (Fig. 5). TS1 generated by a β attack shows a BCP between CH3O⋯CNCH3, with ρ and its Laplacian values being 0.008 and 0.007 respectively, suggesting weak interactions which stabilize the TS while the TS1 for an α attack is devoid of these interactions. Also the ρ and its Laplacian values, and the bond lengths suggest that the TS1 that is considering an α attack is a tight TS while for a β attack is a loose TS which in turn suggests the preferred attack of β over α.
Plausible mechanism
Considering the findings from computational studies and related experiments, a plausible mechanism has been elucidated in Fig. 7. The activation of imine followed by a favourable attack of isocyanide from the Si face to yield the transient reactive intermediate A which reacts with acid to form more a stable reactive intermediate C which then rearranges to the desired product. The formation of a bridged oxazolidine intermediate23 was ruled out on the basis of observations from experiments shown in Scheme 1.
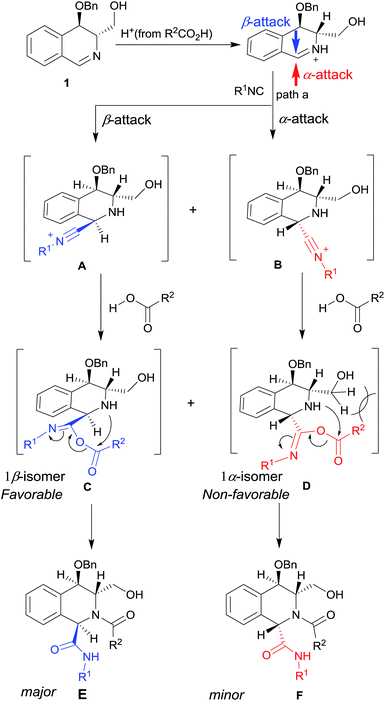 |
| Fig. 7 Plausible mechanism. | |
In order to explore the application of the present methodology, an intramolecular Mitsunobu reaction was performed with compound 5p to obtain THIQ fused piperazinone 15 in good yield (Scheme 4). THIQ fused piperazinones are highly abundant in natural products and have been widely used in total syntheses.29
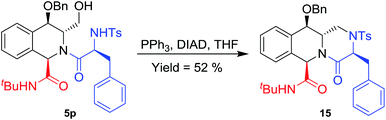 |
| Scheme 4 Preparation of hexahydropyrazinoisoquinolinone. | |
Anticancer activity
To reflect the pharmaceutical significance of synthesized THIQs, the cytotoxicity profile of selected compounds was evaluated against four types of human cancer cell lines such as MCF-7 (breast cancer), MDA-MB-231 (breast cancer), DU-145 (prostate cancer), A549 (lung cancer) and HepG2 (liver cancer) [Table 2].30 The compounds 5a, 5d, 5g, 5i and 5p exhibited a good anticancer profile against most of the cancer cell lines. The GI50 values were encouraging and infer that a suitable modification of the substituent may lead to some potential anticancer hits.
Table 2 Cytotoxicity data of selected compoundsa
Entry |
Compound |
GI50 (μM) |
MCF-7 |
MDA MB-231 |
DU-145 |
A549 |
HepG2 |
MCF-7 (breast cancer), MDA MB-231 (breast cancer), DU-145 (prostate cancer), A549 (lung cancer) and HepG2 (liver cancer). |
1 |
5a |
4.148 |
6.883 |
5.813 |
12.48 |
7.690 |
2 |
5b |
9.419 |
11.34 |
20.16 |
18.00 |
13.42 |
3 |
5c |
8.941 |
12.81 |
18.29 |
21.09 |
12.90 |
4 |
5d |
2.467 |
2.629 |
4.500 |
7.358 |
5.417 |
5 |
5e |
4.031 |
9.167 |
12.21 |
28.16 |
11.91 |
6 |
5f |
4.644 |
7.755 |
7.971 |
25.41 |
11.26 |
7 |
5g |
2.000 |
2.558 |
2.273 |
4.964 |
4.898 |
8 |
5i |
3.257 |
4.902 |
7.370 |
27.31 |
8.038 |
9 |
5p |
2.364 |
3.615 |
5.939 |
9.677 |
6.320 |
10 |
11 |
5.500 |
1.622 |
10.42 |
54.33 |
9.114 |
11 |
Doxorubicin |
1.026 |
2.122 |
0.955 |
1.951 |
1.487 |
12 |
Tamoxifen |
8.083 |
11.73 |
19.33 |
24.42 |
21.78 |
Conclusions
A concise and highly stereoselective synthetic route for the construction of densely functionalized enantiopure 1,2,3,4-tetrahydroisoquinolines (THIQs) from 3,4-dihydroisoquinoline has been explored by employing Ugi three-component reactions. This methodology is efficient and robust enough to produce a diverse collection of biologically relevant 1,2,3,4-tetrasubstituted THIQs. Detailed experimental as well as computational studies were performed to understand the required elements causing the high diastereoselectivity. Considering the challenges in attaining excellent diastereoselectivity in one of the most widely used Ugi reactions, we believe that the outcomes of the present methodology will render solutions to synthesize complex alkaloids. We utilized the inherent chirality of Garner’s aldehyde through 1,2- and 1,3-/1,4-asymmetric inductions to obtain fully functionalized THIQs without any external chiral sources. This novel method can be useful for the synthesis of THIQ-containing chiral bioactive natural products and their derivatives. The cytotoxicity data of selected compounds reflected the pharmaceutical potential of these THIQs.
Acknowledgements
This work was founded by the Council of Scientific and Industrial Research (CSIR)-New Delhi under the 12th Five year plan project ‘Affordable Cancer Therapeutics – ACT’ (CSC0301). TR thanks CSIR, New Delhi for providing fellowships. GG thanks DST for funding in the form of Fast-track Young Scientist Scheme. Dr G. Narahari Sastry is thanked for his suggestions and guidance during molecular modelling studies.
Notes and references
-
(a) B. H. Rotstein, S. Zaretsky, V. A. Rai and K. Yudin, Chem. Rev., 2014, 114, 8323–8359 CrossRef CAS PubMed;
(b) A. Domling, W. Wang and K. Wang, Chem. Rev., 2012, 112, 3083–3135 CrossRef CAS PubMed;
(c) I. Ugi, B. Werner and A. Dömling, Molecules, 2003, 8, 53–66 CrossRef PubMed.
- A. Dömling and I. Ugi, Angew. Chem., Int. Ed., 2000, 39, 3168–3210 CrossRef.
-
(a) S. S. van Berkel, B. G. M. Bögels, M. A. Wijdeven, B. Westermann and F. P. J. T. Rutjes, Eur. J. Org. Chem., 2012, 3543–3559 CrossRef CAS PubMed;
(b) M. C. Pirrung and K. D. Sarma, J. Am. Chem. Soc., 2004, 126, 444–445 CrossRef CAS PubMed;
(c) R. W. Armstrong, A. P. Combs, P. A. Tempest, S. D. Brown and T. A. Keating, Acc. Chem. Res., 1996, 29, 123–131 CrossRef CAS;
(d) S. Zaretsky, S. Adachi, B. H. Rotstein, J. L. Hickey, C. C. G. Scully, J. D. St. Denis, R. Courtemanche, J. C. Y. Yu, B. K. W. Chung and A. K. Yudin, J. Org. Chem., 2014, 79, 9948–9957 CrossRef CAS PubMed.
-
(a) T. Ngouansavanh and J. Zhu, Angew. Chem., Int. Ed., 2007, 46, 5775–5778 CrossRef CAS PubMed;
(b) I. Schuster, L. Lázár and F. Fülöp, Synth. Commun., 2010, 40, 2488–2498 CrossRef CAS PubMed.
-
(a) M. J. Arévalo, N. Kielland, C. Masdeu, M. Miguel, N. Isambert and R. Lavilla, Eur. J. Org. Chem., 2009, 617–625 CrossRef PubMed;
(b) M. Li, X.-L. Lv, L.-R. Wen and Z.-Q. Hu, Org. Lett., 2013, 15, 1262–1265 CrossRef CAS PubMed;
(c) A. Bhunia, D. Porwal, R. G. Gonnade and A. T. Biju, Org. Lett., 2013, 15, 4620–4623 CrossRef CAS PubMed.
-
(a) G. Lesma, F. Meneghetti, A. Sacchetti, M. Stucchi and A. Silvani, Beilstein J. Org. Chem., 2014, 10, 1383–1389 CrossRef PubMed;
(b) D. Zou, L. Xia, L. Pan, S. Li, R. Chen, Y. Mou and X. Chen, J. Org. Chem., 2012, 77, 1386–1395 CrossRef PubMed;
(c) G. Dyker, K. Breitenstein and G. Henkel, Tetrahedron: Asymmetry, 2002, 13, 1929–1936 CrossRef CAS;
(d) K. Oertel, G. Zech and H. Kunz, Angew. Chem., Int. Ed., 2000, 39, 1431–1433 CrossRef CAS;
(e) G. F. Ross, E. Herdtweck and I. Ugi, Tetrahedron, 2002, 58, 6127–6133 CrossRef CAS;
(f) M. Jida, C. Betti, Z. Urbanczyk-Lipkowska, D. Tourwé and S. Ballet, Org. Lett., 2013, 15, 5866–5869 CrossRef CAS PubMed.
-
(a) A. Basso, L. Banfi, R. Riva and G. Guanti, J. Org. Chem., 2005, 70, 575–579 CrossRef CAS PubMed;
(b) L. Banfi, A. Basso, V. Cerulli, V. Rocca and R. Riva, Beilstein J. Org. Chem., 2011, 7, 976–979 CrossRef CAS PubMed.
- K. Katayama, T. Okamura, T. Sunadome, K. Nakagawa, H. Takeda, M. Shiro, A. Matsuda and S. Ichikawa, J. Org. Chem., 2014, 79, 2580–2590 CrossRef CAS PubMed.
- D. Zhu, L. Xia, L. Pan, S. Li, R. Chen, Y. Mou and X. Chen, J. Org. Chem., 2012, 77, 1386–1395 CrossRef CAS PubMed.
-
(a) K. M. Bongera, T. Wennekesa, S. V. P. de Lavoir, D. Esposito, R. J. B. H. N. van den Berg, R. E. J. N. Litjens, G. A. van der Marel and H. S. Overkleeft, QSAR Comb. Sci., 2006, 25, 491–503 CrossRef PubMed;
(b) K. M. Bonger, T. Wennekes, D. V. Filippov, G. Lodder, G. A. van der Marel and H. S. Overkleeft, Eur. J. Org. Chem., 2008, 3678–3688 CrossRef CAS PubMed.
- E. R. vanRijssel, T. P. M. Goumans, G. Lodder, H. S. Overkleeft, G. A. van der Marel and J. D. C. Codee, Org. Lett., 2013, 15, 3026–3029 CrossRef CAS PubMed.
-
(a) A. Znabet, E. Ruijter, F. J. J. de Kanter, V. Köhler, M. Helliwell, N. J. Turner and R. V. A. Orru, Angew. Chem., Int. Ed., 2010, 49, 5289–5292 CrossRef CAS PubMed;
(b) V. Cerulli, L. Banfi, A. Basso, V. Rocca and R. Riva, Org. Biomol. Chem., 2012, 10, 1255–1274 RSC;
(c) L. Moni, L. Banfi, A. Basso, L. Carcone, M. Rasparini and R. Riva, J. Org. Chem., 2015, 80, 3411–3428 CrossRef CAS PubMed.
- P. Szcześniak, E. Maziarz, S. Stecko and B. Furman, J. Org. Chem., 2015, 80, 3621–3633 CrossRef PubMed.
- I. Schuster, A. Sztojkov-Ivanov, L. Lázár and F. Fülöp, Lett. Org. Chem., 2007, 4, 102–108 CrossRef CAS.
-
(a) A. Endo, A. Yanagisawa, M. Abe, S. Tohma, T. Kan and T. Fukuyama, J. Am. Chem. Soc., 2002, 124, 6552–6554 CrossRef CAS PubMed;
(b) S. Zheng, C. Chan, T. Furuuchi, B. J. D. Wright, B. Zhou, J. Guo and S. J. Danishefsky, Angew. Chem., Int. Ed., 2006, 45, 1754–1759 CrossRef CAS PubMed.
-
(a) T. Fukuyama, L. Yang, K. L. Ajeck and R. A. Sachleben, J. Am. Chem. Soc., 1990, 112, 3712–3713 CrossRef CAS;
(b) J. W. Lown, A. V. Joshua and J. S. Lee, Biochemistry, 1982, 21, 419–428 CrossRef CAS.
-
(a) P. Siengalewicz, U. Rinner and J. Mulzer, Chem. Soc. Rev., 2008, 37, 2676–2690 RSC;
(b) P. Garner, K. Sunitha, W. B. Ho, W. J. Youngs, V. O. Kennedy and A. Djebli, J. Org. Chem., 1989, 54, 2041–2042 CrossRef CAS.
-
(a) J. D. Scott and R. M. Williams, J. Am. Chem. Soc., 2002, 124, 2951–2956 CrossRef CAS PubMed;
(b) Y.-C. Wu, M. Liron and J. Zhu, J. Am. Chem. Soc., 2008, 130, 7148–7152 CrossRef CAS PubMed;
(c) H. Saito, T. Hirata, M. Kasai, K. Fujimoto, T. Ashizawa, M. Morimoto and A. Sato, J. Med. Chem., 1991, 34, 1959–1966 CrossRef CAS.
- J. D. Scott and R. M. Williams, Chem. Rev., 2002, 102, 1669–1730 CrossRef CAS PubMed.
- J. W. Lane, Y. Chen and R. W. Williams, J. Am. Chem. Soc., 2005, 127, 12684–12690 CrossRef CAS PubMed.
-
(a) P. Magnus and K. S. Matthews, J. Am. Chem. Soc., 2005, 127, 12476–12477 CrossRef CAS PubMed;
(b) X. Li and I. Coldham, J. Am. Chem. Soc., 2014, 136, 5551–5554 CrossRef CAS PubMed;
(c) S. Zhou, V. Wang, D. Lin, F. Zhao and H. Liu, J. Org. Chem., 2013, 78, 11204–11212 CrossRef CAS PubMed.
- E. Mons, M. J. Wanner, S. Ingemann, J. H. Van Maarseveen and H. Hiemstra, J. Org. Chem., 2014, 79, 7380–7390 CrossRef CAS PubMed.
- A. K. Srivastava, M. Koh and S. B. Park, Chem.–Eur. J., 2011, 17, 4905–4913 CrossRef CAS PubMed.
- D. X. Hu, P. Grice and S. V. Ley, J. Org. Chem., 2012, 77, 5198–5202 CrossRef CAS PubMed.
- M. J. Frisch, G. W. Trucks, H. B. Schlegel, G. E. Scuseria, M. A. Robb, J. R. Cheeseman, G. Scalmani, V. Barone, B. Mennucci, G. A. Petersson, H. Nakatsuji, M. Caricato, X. Li, H. P. Hratchian, A. F. Izmaylov, J. Bloino, G. Zheng, J. L. Sonnenberg, M. Hada, M. Ehara, K. Toyota, R. Fukuda, J. Hasegawa, M. Ishida, T. Nakajima, Y. Honda, O. Kitao, H. Nakai, T. Vreven, J. A. Montgomery Jr, J. E. Peralta, F. Ogliaro, M. Bearpark, J. J. Heyd, E. Brothers, K. N. Kudin, V. N. Staroverov, R. Kobayashi, J. Normand, K. Raghavachari, A. Rendell, J. C. Burant, S. S. Iyengar, J. Tomasi, M. Cossi, N. Rega, J. M. Millam, M. Klene, J. E. Knox, J. B. Cross, V. Bakken, C. Adamo, J. Jaramillo, R. Gomperts, R. E. Stratmann, O. Yazyev, A. J. Austin, R. Cammi, C. Pomelli, J. W. Ochterski, R. L. Martin, K. Morokuma, V. G. Zakrzewski, G. A. Voth, P. Salvador, J. J. Dannenberg, S. Dapprich, A. D. Daniels, Ö. Farkas, J. B. Foresman, J. V. Ortiz, J. Cioslowski and D. J. Fox, Gaussian 09, Revision C.01, Gaussian, Inc., Wallingford CT, 2009 Search PubMed.
- U. D. Priyakumar, G. N. Sastry and G. Mehta, Tetrahedron, 2004, 60, 3465–3472 CrossRef CAS PubMed.
- N. Cheron, R. Ramozzi, L. El Kaïm, L. Grimaud and P. Fleurat-Lessard, J. Org. Chem., 2012, 77, 1361–1366 CrossRef CAS PubMed.
- F. Biegler-König and J. Schönbohm, AIM 2000 Version 2.0, University of Applied Science, Bielefeld, Germany, 2002 Search PubMed.
- D. Fishlock and R. M. Williams, J. Org. Chem., 2008, 73, 9594–9600 CrossRef CAS PubMed.
- V. Vichai and K. Kirtikara, Nat. Protoc., 2006, 1, 1112–1116 CrossRef CAS PubMed.
Footnote |
† Electronic supplementary information (ESI) available: 1H and 13C NMR and HRMS spectra of all the new compounds, COSY and NOESY spectra of compounds 5a, 7 and 11, details of computational studies (Cartesian coordinates and absolute energies). See DOI: 10.1039/c5ra11144g |
|
This journal is © The Royal Society of Chemistry 2015 |
Click here to see how this site uses Cookies. View our privacy policy here.