DOI:
10.1039/C5RA13861B
(Paper)
RSC Adv., 2015,
5, 73601-73611
In vivo biodistribution and toxicity of Gd2O3:Eu3+ nanotubes in mice after intraperitoneal injection
Received
14th July 2015
, Accepted 23rd August 2015
First published on 24th August 2015
Abstract
To better understand the potential impact of Eu3+ doped gadolinium oxide nanotubes (Gd2O3:Eu3+ nanotubes) on human health, we investigated their biodistribution, subacute toxicity, and hepatic injury in mice under different dosages (4.0, 40.0, and 400.0 mg kg−1). The results showed that the gadolinium element was mainly accumulated in the spleen, liver, lung, kidney, and bone. The relative organ weight of spleen in the middle-dose group and high-dose group was significant higher than that of the control group. However, the relative organ weight of liver and kidney had no obvious difference from the control group. Besides, the change of toxicity on the hematological system was not noticeable under the tested doses. The high-dose Gd2O3:Eu3+ nanotubes increased the alanine aminotransferase (ALT), aspartate aminotransferase (AST), and alkaline phosphatase (ALP) levels, but no significant difference was observed in the low-dose group and middle-dose group compared with the control group. These changes demonstrated that high-dose Gd2O3:Eu3+ nanotubes induced liver injury. Based on the changes of superoxide dismutase (SOD), glutathione peroxidase (GSH-Px), catalase (CAT), glutathione S-transferase (GST), reactive oxygen species (ROS), malondialdehyde (MDA), and protein carbonylation levels, it can be deduced that high-dose Gd2O3:Eu3+ nanotubes could induce liver injury by oxidative stress. Furthermore, the levels of inflammatory cytokines, such as tumor necrosis factor alpha (TNF-α), interleukin-1β (IL-1β), and interleukin-8 (IL-8), increased upon high-dose Gd2O3:Eu3+ nanotube treatment. The results may benefit the applications of Gd2O3:Eu3+ nanotubes.
Introduction
Rare earth nanomaterials, which possess excellent characteristic features, have been widely used in electronic, chemical, pharmaceutical, and many other fields.1 For example, Gd2O3 nanoparticles have found potential applications in biomedical fields. They can be used as contrast agents in MRI (magnetic resonance imaging), X-ray tomography, and ultrasound scanners due to their good paramagnetic characteristics.2,3 Gd2O3 nanotubes were employed as site-specific drug carriers for cancer therapy,4,5 which can improve the killing efficiency and decrease the adverse effects.6,7 The inner surface of nanotubes can be modified for better encapsulation of drugs, and the outer surface can be tailored for targeted delivery.8 In addition, the magnetic capability of Gd2O3:Eu3+ nanotubes under magnetic field can facilitate targeted drug delivery.9,10 Recently, Zhou et al. synthesized a single-phase mesoporous Gd2O3:Eu3+ nanorods, which showed a mesoporous structure and multimodal imaging properties.11
It is well known that nanomaterials have potential hazardous bioeffects. Setyawati et al. reported that Gd2O3:Tb3+ nanoparticles showed cytotoxicity against human neonatal foreskin fibroblast cells in a dose-dependent way, and induced genotoxicity via DNA damage.1 It was also reported that ligand-free monoclinic Gd2O3 nanocrystals displayed lower toxicity for S18 cells (CNE-2 clone 18) and RAW264.7 cells (mouse macrophage cells).12,13 Gd2O3 nanoparticles have been synthesized and encapsulated in phospholipid micelles to improve their biocompatibility and resolution, which demonstrated that these micelle-coated nanoparticles could be taken up by human embryonic kidney 293 cells and exhibited less cytotoxicity.14 Besides, the toxicity assessments showed that Gd2O3:Yb3+,Er3+ nanostructures had no cytotoxic effect on B-cell hybridomas, but induced significant cell death in macrophages.15
As mentioned above, although Gd2O3:Eu3+ nanotubes may be promising in biomedical fields, more wide-scale biomedical applications have been limited seriously due to the absence of a thorough evaluation on their toxicity. Therefore, the risk level of Gd2O3:Eu3+ nanotubes to the general public must be taken into consideration. In this study, the biodistribution and toxicity of the Gd2O3:Eu3+ nanotubes in mice after intraperitoneal injection have been investigated in detail. The results showed that gadolinium element was mainly accumulated in the spleen, liver, lung, kidney, and bone. The significant lesion of liver was induced by high-dose Gd2O3:Eu3+ nanotubes, and the oxidative stress may be the mechanism of liver lesions. The results may benefit the applications of Gd2O3:Eu3+ nanotubes in biomedical fields.
Experimental section
Animal and materials
ICR mice were obtained from Beijing Vitalriver Experimental Animal Technology Co. Ltd China. All procedures used in this animal experiment were compliant with the local ethics committee. Gd2O3 and Eu2O3 (99.99%) were supplied by Wuxi Yiteng Rare-Earth Co., Ltd China, and Gd2O3 and Eu2O3 were added into dilute HNO3 solution to obtain Gd(NO3)3 and Eu(NO3)3 aqueous solutions under heating with stirring. The assay kits of total bilirubin levels (TBIL), alanine aminotransferase (ALT), alkaline phosphatase (ALP), and aspartate aminotransferase (AST) were purchased from Beijing Capital Medical University Clinical Science Center, China. The assay kits of uric acid (UA), creatinine (Cr), blood urea nitrogen (BUN) creatine kinase (CK), lactate dehydrogenase (LDH), and alpha-hydroxybutyrate dehydrogenase (HBDH) were purchased from (Randox Laboratories Ltd UK). The assay kits of blood-element were purchased from Randox Laboratories Ltd UK. The assay kits of glutathione peroxidase (GSH-Px), glutathione S-transferase (GST), superoxide dismutase (SOD), catalase (CAT), reactive oxygen species (ROS), and malondialdehyde (MDA) were purchased from Nanjing Jiancheng Bioengineering Institute, China. The enzyme linked immunosorbent assay (ELISA) kits of tumor necrosis factor alpha (TNF-α), interleukin-1β (IL-1β), interleukin-8 (IL-8) were provided by R&D Systems, Oxford, UK.
Synthesis and characterizations of Gd2O3:Eu3+ nanotubes
The Gd2O3:Eu3+ nanotubes were synthesized by a wet-chemical route at ambient pressure according to our previous report.16 In a typical synthesis, 1.9 mL of Gd(NO3)3 (1 M) and 1 mL of Eu(NO3)3 (0.1 M) aqueous solution was added to 25 mL of deionized water. Subsequently, ammonia solution was dropped to the stirred solution until pH 10. The mixing solution was then heated to 75 °C for 18 h with stirring. The as-obtained precipitate was centrifuged, washed with deionized water, and dried in air. The sample was annealed at 650 °C for 2 h in air to obtain the final product.
The crystal phase of the sample was characterized by powder X-ray diffraction (XRD) (Bruker, D8 Advance, Germany). The morphology was inspected using SEM (scanning electron microscope) (JEOL, JSM-7500F, Japan) and transmission electron microscopy (TEM) (FEI Tecnai G2 S-Twin, America). Photoluminescence (PL) excitation and emission spectra were recorded with a spectrophotometer equipped with a 150 W xenon lamp as the excitation source (F-7000, Hitachi, Japan). All measurements were performed at room temperature.
Preparation of Gd2O3:Eu3+ nanotubes suspension
Briefly, 4.0, 40.0, and 400.0 mg of Gd2O3:Eu3+ nanotubes were suspended in 10 mL saline solution and dispersed by ultrasonic vibration for 30 min. The concentrations of Gd2O3:Eu3+ nanotubes in the suspension were 0.4, 4.0, and 40.0 mg mL−1, respectively. In order to avoid the aggregation of the nanotubes, a few glass beads were added to the suspension and then stirred on vortex agitator before every use.
Animals and treatment
Forty female ICR mice (18–20 g) were housed and maintained by commercial pellet diet, given deionized water ad libitum, kept in plastic cages in a 20 ± 2 °C, 60 ± 10% relative humidity room with a 12 h light/dark cycle. After a week acclimation, the mice were randomly divided into four groups: control group (physiological saline), low-dose group (4.0 mg kg−1), middle-dose group (40.0 mg kg−1), and high-dose group (400.0 mg kg−1). The dosages were designed according to “long-term toxicity test of the chemical technical guiding principles (written by China food and chemical administration)”. The guiding principles required that the subacute toxicity tests must be set up three doses at least, named as low-dose group, middle-dose group and high-dose group. High dose should make animals produce obvious toxic effects even individual animals are dead in principle. Low dose should not make obvious toxic effects. In order to examine dose-effect relationship, middle-dose group should be set up. Moreover, high dose should be 1/20–1/5 LD50. Preliminary tests indicated that the LD50 of Gd2O3:Eu3+ nanotubes was greater than 2000 mg kg−1. All animals were administered via IP at dose of 0.1 mL/10 g every day over a period of 35 days.
After administration, the symptoms including changes in skin, fur, eyes membranes, respiratory, circulatory, autonomic and central nervous systems, and behavior pattern were observed daily. The body weight (g), food (g) and water (mL) consumption were also recorded. After 35 days administration, all animals were anaesthetized with ether and then sacrificed by exsanguination. Blood was collected from ophthalmic veins. The organic tissues of heart, liver, spleen, lung, and kidney were collected. The tissues were kept in 10% formalin for histopathological examination.17
Relative organ weight of liver, kidney, and spleen
After weighing the body and organs, the relative organ weight of liver, kidney, and spleen to body weight was calculated as the ratio of organs (wet weight, mg) to body weight (g).
Biochemical analysis of serum
Serum was obtained by centrifuging blood at 3000 rpm for 15 min, and the biochemical markers in serum were assayed using an automatic biochemical analyzer (7600-110, Hitachi, Japan). Liver function was evaluated by the levels of TBIL, ALT, ALP, and AST. The nephrotoxicity was determined by the levels of UA, Cr, and BUN. The enzymes of CK, LDH, and HBDH were assayed for evaluating cardiac damage. All the biochemical markers were determined using assay kits.
Blood-element test
The blood-element: white blood cell (WBC), blood platelet (PLT), red blood cell (RBC), hemoglobin (HGB), mean cell hemoglobin concentration (MCHC), haematocrit (HCT), mean corpuscular volume (MCV), and red cell distribution width corpuscular volume (RDW-CV) were determined using assay kits within 2 h by an automatic hematology analyzer (COULTER LH 750, Beckman, American).
Analysis of gadolinium biodistribution
Tissues were weighed, digested and analyzed for gadolinium concentration. Briefly, 0.1–0.2 g tissues were digested in nitric acid (ultrapure grade) overnight. After that, mixed acid (nitric acid
:
perchloric acid, 6
:
1) was added. In order to completely digest the samples, the solution was heated at 120 °C until the solution was colorless and clear. Finally, the remaining solution was diluted to 3 mL with 2% nitric acid. The gadolinium concentration in the samples was analyzed using ICP-MS (inductively coupled plasmamass spectrometry) (Elan9000/DRC-e, Perkin-Elmer). Indium of 20 ng mL−1 was chosen as an internal standard. The detection limit of gadolinium was 0.092 ng mL−1.18
Histopathological examination
For pathological studies, all histopathological tests were performed according to standard laboratory procedures. The vital organs (heart, liver, kidney, spleen, and lung) were fixed with 10% formalin, embedded in paraffin blocks, then sliced into 5 μm in thickness and placed onto glass slides. After hematoxylin–eosin (HE) staining, the slides were observed using optical microscopy (BX53, Olympus, Japan). The identity and analysis of pathology sections were blind to the pathologist.
Preparation of liver tissue homogenates
0.1–0.3 g liver tissues were weighed, washed several times with cold physiological saline, minced and transferred into glass homogenizer. After adding 1
:
9 (w/v) volume of ice-cold physiological saline, and the mixtures were homogenized in an ice bath. Finally, the homogenates were centrifuged at 3000 rpm for 10 min at 4 °C, and the supernatant was used to assay oxidative biomarkers.
Isolation of hepatocytes
Hepatocytes were isolated from liver tissue by the perfusion technique.19 Briefly, liver tissues were extensively perfused in situ with PBS, cut up into small pieces, and digested by trypsin for about 30 min at 37 °C. Then they were centrifuged at 1000 rpm for 10 min at 4 °C, and resuspended in PBS. The cells were washed with PBS three times, the solution was filtered, centrifuged and the pellet was suspended in DMEM containing 10% FBS.
Measurement of the activities of antioxidant enzymes
The activities of GSH-Px, GST, SOD, and CAT in liver tissues were measured according to the method reported by Wang et al.20 The protein contents in liver tissue were measured according to Bradford's method.21
Measurement of intracellular ROS
Intracellular ROS production was quantified by using 2,7-dichlorofluorescein diacetate (DCF-DA). Briefly, hepatocytes were cultured with DMEM including 6 μM DCFH-DA for 30 min at 37 °C in 5% CO2 atmosphere. Then, cells were washed twice with PBS and centrifuged at 1000g for 5 min. The pellets were suspended in PBS, and 1 × 104 events per sample were assayed by a FACScalibur flow cytometer (FACSCalibur™, Becton Dickinson, American). The ROS level was expressed as the ratio of mean intensity of sample/mean intensity of control cells. The data were analyzed by CELLQuest 6.0 software.22,23
Measurement of MDA and protein carbonyl content
Cell membrane damage and modification of proteins were measured by lipid peroxidation and protein carbonyl content assays, respectively. The lipid peroxidation product MDA was measured using thiobarbituric acid (TBA). Protein carbonyl content in the homogenate was determined by the routine method illustrated in specification of kits.
Measurement of inflammatory cytokines
TNF-α, IL-1β, and IL-8 could induce liver damage, in which TNF-α is the strongest cytokine for liver damage, and IL-1β can strengthen the liver damage induced by TNF-α. To further assess the inflammatory response by Gd2O3:Eu3+ nanotubes treatment, inflammatory cytokines such as TNF-α, IL-1β, and IL-8 in the liver tissues were measured by enzyme linked immunosorbent assay (ELISA) kits. The assays were performed according to the manufacturer's instructions. Finally, the optical density (OD) were measured under 450 nm using a microplate spectrophotometer (Molecular Devices, VersaMax, USA).
Determination of mitochondrial membrane potential (MMP)
Rh123 staining was used to determine the alteration of MMP.23 Briefly, hepatocytes were washed twice with PBS, digested by trypsin solution, and stained with Rh123 (10 mM) for 30 min at 37 °C in the dark. The uptake of RH123 was analyzed using a FACSCalibur flow cytometer (FACSCalibur™, Becton Dickinson, American) in the FL1 channel (530 ± 15 nm) with the excitation wavelength at 488 nm. MMP was expressed as the ratio of mean intensity of sample/mean intensity of control cells. Ten thousand cells per sample were acquired in histograms and the data were analyzed with CELLQuest 6.0 software.
Statistical analysis
All data were expressed as mean ± standard deviation (SD), and the statistical differences between the experimental groups and the control group were analyzed using the “Student–Newman–Keuls Multiple Comparisons Test” (ANOVA). p < 0.05 was considered to indicate statistical significance.
Results
Characterizations and properties of Gd2O3:Eu3+ nanotubes
Fig. 1a and b showed the SEM and TEM images of the as-obtained Gd2O3:Eu3+ sample. The typical SEM image (Fig. 1a) indicated that the Gd2O3:Eu3+ sample was composed of a large scale of uniform and well-dispersed nanotubes with open ends. The diameters and lengths were about 50 nm and 300 nm. These nanotubes were non-aggregated with narrow size distribution. From the TEM image (Fig. 1b), the nanotube structure can be confirmed by a contrast between the edge and central part, which agreed well with the SEM result. Fig. 1c showed that the diffraction peaks of the sample were well indexed to cubic Gd2O3 phase (JCPDS 86-2477). No other impurity peaks can be detected, indicating that the Eu3+ ions have been effectively doped into the Gd2O3 host lattice. The PL properties of the Gd2O3:Eu3+ nanotubes were characterized by the PL excitation and emission spectra (Fig. 1d). The excitation spectrum of the Gd2O3:Eu3+ sample was composed of a broad band with a maximum at about 254 nm and some sharp lines from 200 to 450 nm, which can be attributed to the charge-transfer band (CTB) between O2− and Eu3+ and the f–f transitions of Eu3+ ions, respectively. Upon excitation at 254 nm, the emission spectrum consisted of a group of sharp lines, which can be assigned to 5D0–7FJ (J = 0–4) transition lines of the Eu3+ ions. The characteristic red emission of Eu3+ ions with 5D0–7F2 transition (610 nm) was the most prominent compared with other transition lines. The result was in good accordance with those of other Gd2O3:Eu3+ phosphors.16,24
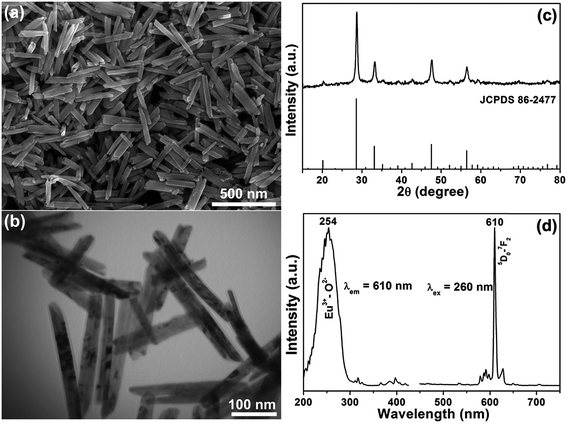 |
| Fig. 1 (a) SEM, (b) TEM images, (c) XRD pattern, and (d) PL excitation and emission spectra of the as-synthesized Gd2O3:Eu3+ nanotubes. | |
Relative organ weight of liver, spleen, and kidney
The obvious abdominal obesity was found in the mice exposed to high-dose Gd2O3:Eu3+ nanotubes after intraperitoneal injection for three weeks, but overt toxic effects and mortality were not observed in all groups. There was statistically significant difference of body weights between the high-dose group and control group after three weeks (Fig. 2), whereas no significant change in food intake was observed between the experimental groups and control group (Fig. 3). When the mice were sacrificed after thirty days, splenomegaly, vast ascites, and some white particulates with tunicary deposited on spleen, liver, intestine and peritoneum in the mice were observed in high-dose group, but no abnormal change was observed in the mice of other groups.
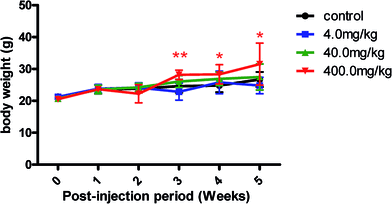 |
| Fig. 2 Effects of repeated administration of Gd2O3:Eu3+ nanotubes on body weight in mice. *p < 0.05, **p < 0.01 versus the control. | |
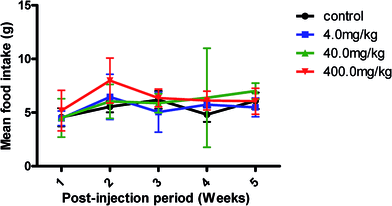 |
| Fig. 3 Effects of repeated administration of Gd2O3:Eu3+ nanotubes on mean food intakes in mice. | |
As shown in Table 1, compared with the control group, the significant difference was not observed in the relative organ weight of liver and kidney in the middle-dose and high-dose group. However, the relative organ weight of spleen in the middle-dose and high-dose group was much higher than that of the control group (p < 0.05).
Table 1 The relative organ weight of liver, spleen and kidney in mice after intraperitoneal injection with Gd2O3:Eu3+ nanotubes (mean ± SD, n = 10)a
Groups |
Body weight (g) |
Liver (mg g−1) |
Spleen (mg g−1) |
Kidney (mg g−1) |
*p < 0.05 versus the control. |
Control |
26.7 ± 2.36 |
57.42 ± 6.94 |
4.64 ± 1.29 |
15.49 ± 1.64 |
4.0 mg kg−1 |
24.80 ± 2.58 |
57.55 ± 5.16 |
5.06 ± 1.25 |
15.39 ± 2.23 |
40.0 mg kg−1 |
27.5 ± 4.09 |
57.17 ± 7.55 |
6.87 ± 2.78* |
14.16 ± 2.77 |
400.0 mg kg−1 |
31.50 ± 6.59* |
59.99 ± 11.68 |
7.27 ± 1.27* |
14.07 ± 2.82 |
Biochemical parameters in serum
The biochemical parameters in serum were presented in Table 2. Generally, the levels of TBIL, ALP, ALT, AST, and AST/ALT were used to evaluating the liver function. The levels of these parameters of the low-dose and middle-dose group were similar to that of the control group (p > 0.05), However, in the high-dose group, the ALT (p < 0.05), AST (p < 0.05), and ALP levels (p < 0.001) increased significantly compared with the control group. The levels of UA, Cr, and BUN are utilized to evaluate the renal functions. No significant difference was observed for UA, Cr, and BUN between the experimental groups and the control group. CK, LDH, and HBDH were generally used for the evaluation of cardiovascular function, and the results showed that there was no significant change of CK, LDH, and HBDH between the experimental groups and the control group.
Table 2 Biochemical parameters in the serum of mice after intraperitoneal injection with Gd2O3:Eu3+ nanotubes (mean ± SD, n = 5)a
Groups |
TBIL (μmol L−1) |
ALT (U L−1) |
AST (U L−1) |
AST/ALT |
ALP (U L−1) |
UA (μmol L−1) |
Cr (μmol L−1) |
BUN (mmol L−1) |
CK (U L−1) |
LDH (U L−1) |
HBDH (U L−1) |
*p < 0.05 versus the control. ***p < 0.001 versus the control. |
Control |
0.72 ± 0.83 |
72.34 ± 21.90 |
166.44 ± 69.37 |
2.26 ± 0.32 |
77.44 ± 18.81 |
177.59 ± 58.46 |
14.51 ± 7.33 |
7.73 ± 1.51 |
882.14 ± 247.23 |
1107.52 ± 477.19 |
426.46 ± 215.27 |
4.0 mg kg−1 |
0.60 ± 0.34 |
76.94 ± 8.34 |
181.86 ± 20.95 |
2.37 ± 0.26 |
60.24 ± 20.62 |
214.27 ± 123.33 |
24.08 ± 11.17 |
8.98 ± 1.96 |
970.10 ± 362.75 |
940.40 ± 380.24 |
362.40 ± 149.91 |
40.0 mg kg−1 |
0.77 ± 0.19 |
93.80 ± 28.86 |
255.04 ± 123.89 |
2.64 ± 0.43 |
82.26 ± 23.35 |
284.05 ± 83.09 |
20.59 ± 8.12 |
8.67 ± 4.06 |
763.96 ± 227.34 |
1123.80 ± 141.91 |
430.46 ± 56.64 |
400.0 mg kg−1 |
0.93 ± 0.0.95 |
135.10 ± 52.55* |
349.14 ± 83.15* |
2.69 ± 0.42 |
143.54 ± 27.15*** |
306.11 ± 54.29 |
20.04 ± 7.03 |
12.54 ± 3.03 |
727.10 ± 208.68 |
1510.18 ± 373.03 |
617.44 ± 231.34 |
In summary, the results indicate that the high-dose Gd2O3:Eu3+ nanotubes could lead to liver injury, but renal toxicity and cardiovascular damage were not obvious. The low-dose and middle-dose Gd2O3:Eu3+ nanotubes hardly exhibited detrimental effects on liver, kidney, and cardiovascular system.
The blood-elements
The changes of blood-elements were presented in Table 3, it can be found that there was no significant difference in the blood-elements between the experimental groups and the control group. In particular, Gd2O3:Eu3+ nanotubes did not cause hematological toxicity such as anemia, lymphopenia, neutropenia or thrombocytopenia even at the highest dose group.
Table 3 Blood-element test and blood coagulation examination of mice after intraperitoneal injection with Gd2O3:Eu3+ nanotubes (mean ± SD, n = 5)
Groups |
WBC (109 L−1) |
RBC (109 L−1) |
HGB (g L−1) |
HCT (L L−1) |
MCV (10−15 L−1) |
MCHC (g L−1) |
RDW (%) |
PLT (109 L−1) |
Control |
6.29 ± 3.35 |
9.58 ± 0.92 |
155.20 ± 16.72 |
51.03 ± 3.72 |
52.70 ± 1.65 |
307.2 ± 11.61 |
21.3 ± 1.63 |
1265.4 ± 158.77 |
4.0 mg kg−1 |
7.90 ± 1.79 |
9.95 ± 0.53 |
161.2 ± 8.67 |
51.85 ± 2.21 |
52.0 ± 0.77 |
311.6 ± 3.78 |
20.7 ± 0.74 |
1212.2 ± 480.51 |
40.0 mg kg−1 |
8.19 ± 2.16 |
10.60 ± 0.51 |
163.6 ± 10.01 |
52.28 ± 2.67 |
51.0 ± 1.44 |
314.8 ± 4.09 |
21.5 ± 1.02 |
976.0 ± 221.16 |
400.0 mg kg−1 |
8.26 ± 2.77 |
9.55 ± 1.02 |
148.6 ± 17.05 |
50.16 ± 5.25 |
51.6 ± 1.34 |
301.8 ± 3.83 |
21.4 ± 1.63 |
1374.6 ± 463.11 |
Histopathological examination
Histopathological studies were carried out to observe the effect of Gd2O3:Eu3+ nanotubes on the vital organs (Fig. 4). Mild congestion and haemorrhage in myocardium were found in all experimental groups. No other significant clinical histopathological alteration in heart tissue can be observed (Fig. 4a–d). In liver tissue, congestion of central veins and hepatic sinusoid, hydropic degeneration and slight necrosis of hepatocytes around the central vein were observed in high-dose group, and mild congestion and edema appeared in middle-dose group. However, these changes were not found in low-dose group (Fig. 4e–h.). The normal red and white pulp in spleen tissue was not affected by Gd2O3:Eu3+ nanotubes (Fig. 4i–l). The mild haemorrhage in lung tissue was observed in the control group and the experimental groups (Fig. 4m–p). The mild degeneration in epithelial cells of renal tubular was only found in high-dose group (Fig. 4q–t).
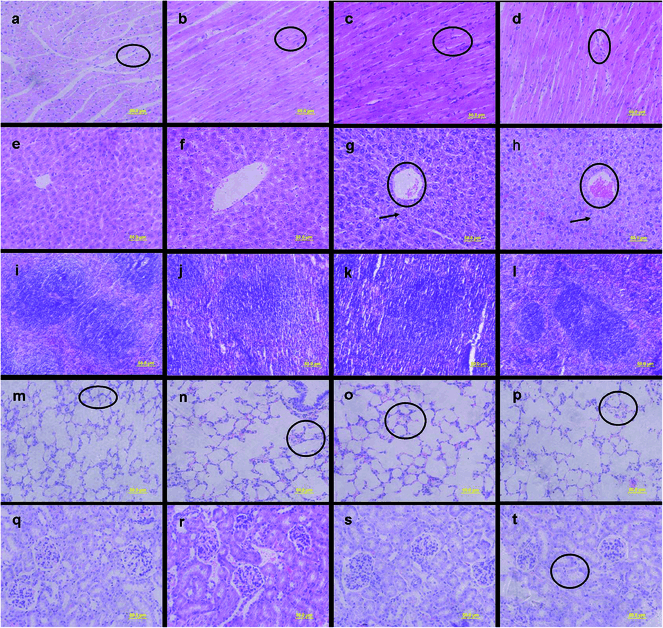 |
| Fig. 4 Histological images of mice tissues (heart, lung, liver, kidney, and spleen) stained with hematoxylin and eosin (HE). Histopathology of the heart tissue (400×) (a–d). (a) Control group; (b) low-dose group; (c) middle-dose group; (d) high-dose group. Circle areas indicated mild congestion and haemorrhage of myocardium. Histopathology of the liver tissue (400×) (e–h). (e) Control group; (f) low-dose group; (g) middle-dose group; (h) high-dose group. Circle areas indicated congestion of central veins. Arrows showed hepatic sinusoid, hydropic degeneration and slight necrosis of hepatocytes around the central vein. Histopathology of the spleen tissue (200×) (i–l). (i) Control group; (j) low-dose group; (k) middle-dose group; (l) high-dose group. No haemorrhage of spleen tissue was observed in both control group and the experimental groups. Histopathology of the lung tissue (400×) (m–p). (m) Control group; (n) low-dose group; (o) middle-dose group; (p) high-dose group. Circle areas indicated mild haemorrhage of lung tissue. Histopathology of the kidney tissue (400×) (q–t). (q) Control group; (r) low-dose group; (s) middle-dose group; (t) high-dose group. Circle areas indicated mild degeneration of epithelial cells of renal tubular. | |
Biodistribution of gadolinium
The biodistribution of gadolinium element in different organs of mice was shown in Fig. 5. It can be seen that gadolinium element mainly accumulated in the spleen, liver, lung, kidney, and bone, but rarely distributed in the heart. This is to say, gadolinium could be entrapped in the reticular-endothelial system and transported to all tissues by blood transportation in vivo.25
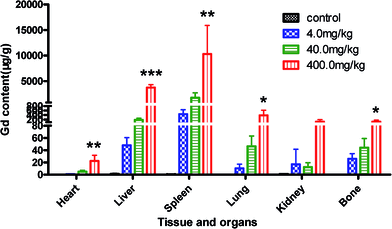 |
| Fig. 5 The biodistribution of gadolinium element (per gram of tissue) in different organs. *p < 0.05, **p < 0.01, ***p < 0.001 versus the control. | |
Activities of hepatic antioxidant enzymes
Oxidative stress is the result of a redox imbalance between the generation of ROS and the compensatory response from the endogenous antioxidant net work.26 To investigate the oxidative damage mechanism of hepatic injury, the activities of antioxidant enzymes SOD, GSH-Px, CAT, and GST were measured (Table 4). The results showed that SOD, GSH-Px, and CAT in high-dose group exhibited lower activities than those in the control group, and the activity of GST in middle-dose group and high-dose group was higher than that in the control group (p < 0.05).
Table 4 Antioxidant enzymes of mice after intraperitoneal injection with Gd2O3:Eu3+ nanotubes (mean ± SD, n = 5)a
Groups |
SOD (unit per mg protein) |
CAT (unit per g protein) |
GST (unit per mg protein) |
GSH-Px (unit per min per mg protein) |
*p < 0.05 versus the control. **p < 0.01 versus the control. ***p < 0.001 versus the control. |
Control |
324.35 ± 43.04 |
460.42 ± 83.78 |
10.99 ± 3.50 |
2385.44 ± 162.05 |
4.0 mg kg−1 |
259.27 ± 57.73 |
474.42 ± 146.34 |
12.92 ± 2.17 |
2063.59 ± 266.18 |
40.0 mg kg−1 |
257.96 ± 26.65 |
307.33 ± 95.89 |
15.93 ± 1.57* |
2257.95 ± 1442.99 |
400.0 mg kg−1 |
177.83 ± 53.71*** |
225.81 ± 77.73** |
18.72 ± 2.67** |
897.88 ± 337.94* |
Effects of Gd2O3:Eu3+ nanotubes on ROS, MDA, and protein carbonyl content
ROS, one of the physiological products of the cells' aerobic metabolism, played an important physiological role in the immune process.27 ROS involves in resisting bacteria, viruses, and other exogenous microbes. When oxidative stress occurs, excess production of ROS can induce cell apoptosis or necrosis. As shown in Table 5, high-dose Gd2O3:Eu3+ nanotubes could increase ROS level (p < 0.05), but no significant differences were observed in the low-dose and middle-dose group.
Table 5 Oxidative stress related biomarker of mice after intraperitoneal injection with Gd2O3:Eu3+ nanotubes (mean ± SD, n = 5)a
Groups |
MDA (nmol mL−1) |
Protein carbonyl (nmol mg−1 protein) |
ROS (% over control) |
*p < 0.05 versus the control. |
Control |
1.60 ± 0.24 |
4.29 ± 1.10 |
1.00 ± 0.01 |
4.0 mg kg−1 |
2.98 ± 2.60 |
4.47 ± 0.62 |
1.20 ± 0.06 |
40.0 mg kg−1 |
2.10 ± 0.56 |
6.28 ± 2.21 |
1.24 ± 0.21 |
400.0 mg kg−1 |
4.85 ± 1.97* |
7.49 ± 1.67* |
1.39 ± 0.04* |
Lipid peroxidation and protein carbonyl content were widely used as the markers of cell membrane damage and oxidative modification of proteins. It can be seen that high-dose Gd2O3:Eu3+ nanotubes increased the levels of MDA and protein carbonylation in the liver tissue (p < 0.05), but there were no differences among low-dose group, middle-dose group, and the control group (Table 5).
Effects of Gd2O3:Eu3+ nanotubes on inflammatory cytokines
IL-1β, IL-8, and TNF-α in the liver tissues were chosen as the indicators of inflammatory response. The results showed that the levels of IL-1β (p < 0.05), IL-8 (p < 0.05), and TNF-α (p < 0.01) increased after treatment with high-dose Gd2O3:Eu3+ nanotubes. However, there was no difference among low-dose group, middle-dose group, and the control group (Fig. 6).
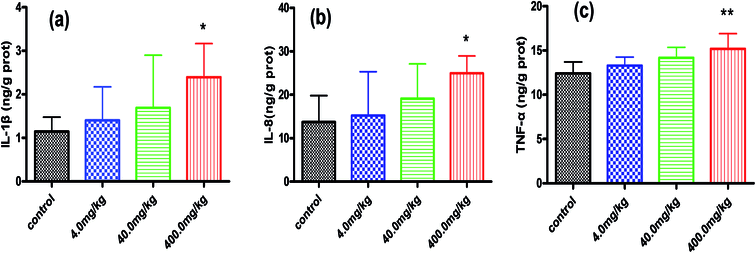 |
| Fig. 6 Levels of IL-1β, IL-8, and TNF-α of mice after intraperitoneal injection with Gd2O3:Eu3+ nanotubes. (a) IL-1β; (b) IL-8; (c) TNF-α. | |
Measurement of MMP
Mitochondrion is one of the most important organelles for ROS generation in cells, and the decrease of MMP is a key step during apoptosis.28 As shown in Fig. 7, high-dose Gd2O3:Eu3+ nanotubes could induce the decrease of MMP (p < 0.05), but there are no significant changes in MMP of low-dose and middle-dose group.
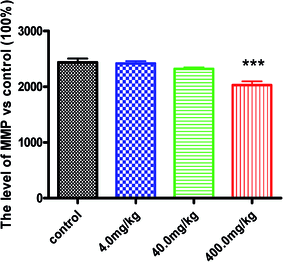 |
| Fig. 7 Mitochondrial membrane potential (MMP) of mice after intraperitoneal injection with Gd2O3:Eu3+ nanotubes. ***p < 0.001 versus the control. | |
Discussion
Toxicity assessments in vivo include acute, subacute, sub-chronic, and chronic toxicity studies. The objective of acute toxicity assessments is usually to identify the maximum tolerated dose (MTD). Subacute toxicity studies are usually the extension of acute toxicity evaluation. Generally, it would take 4–5 weeks to detect adverse effects and toxicity symptoms, and the observation periods for sub-chronic and chronic toxicity studies are 13 weeks and 18–30 months, respectively. Sub-chronic and chronic toxicity studies are performed to satisfy requirements of approval for human use.29 In our study, we performed acute toxicity study firstly, and drew a conclusion that no death of mice was provoked by Gd2O3:Eu3+ nanotubes at a dose of 2000 mg kg−1 within 14 days. On this basis, the subacute toxicity and hepatic injury mechanism of Gd2O3:Eu3+ nanotubes in mice were investigated in detail. It can be found that the hepatic injury was induced by Gd2O3:Eu3+ nanotubes, which were consistent with our previous research results.18 Furthermore, the relative organ weight of spleen in middle-dose and high-dose group was much higher than that of the control group. When the mice were sacrificed, splenomegaly was observed in high-dose group. The reason was that the spleen might be in the stress state after intraperitoneal injection. Gd2O3:Eu3+ nanotubes, as xenobiotics, may cause the hypersplenism, splenomegaly, and medulla cell hyperplasia. Severe hypersplenism could lead to anemia, leukopenia and/or thrombocytopenia.30 Some splenomegaly and hypersplenism had no symptom.31 In our study, the splenomegaly mice did not exhibit obvious symptom and any changes of hematological system.
Liver as an antidotal organ plays an important role for the metabolism of harmful xenobiotics such as drugs and toxic chemicals. These excessive chemicals may mainly cause liver injury characterized by abnormality of hepatic function, and hydropic degeneration, apoptosis or necrosis of hepatocytes, etc.26 Extensive research works have been carried out to investigate liver injury and the mechanism induced by nanoparticles.32 Guo et al. explored the synergistic effects of SiNPs (silica nanoparticles) and CdCl2 on their biodistribution and subacute toxicity in mice.33 In the SiNPs + CdCl2 group, the cadmium accumulation in the liver was markedly increased, and the significant hepatic oxidative stress was induced by co-exposure to SiNPs and CdCl2. The severe infiltration of inflammatory cells, hyperplasia of mesothelial cells, hydropic degeneration, and spotty necrosis in multiple areas were found. Moreover, liver dysfunction induced by nano-copper has been investigated in recent years.34 The results indicated that nano-copper particles could induce hepatotoxicity and hepatocyte cell death via the activation of oxidative stress-responsive cell signaling pathway. In addition, it was reported that gadolinium chloride could promote the liver cell proliferation, DNA replication, and inhibit apoptosis caused by carbon tetrachloride.35,36 Gadolinium chloride pretreatment could also prevent cadmium chloride-induced liver damage in both wild-type and metallothioneins (MT)-null mice.37 However, Greisberg et al. reported that Gd could inhibit the proliferation and induce the apoptosis of macrophage.29 In our study, the hepatotoxicity mechanism of Gd2O3:Eu3+ nanotubes in mice was studied in detail. The results indicated that the ALT, AST, and ALP levels in high-dose group increased significantly compared with that of the control group, so the hepatic dysfunction was induced by high-dose Gd2O3:Eu3+ nanotubes. Moreover, the pathology results also further proved the hepatic injury such as congestion, hydropic degeneration, and slight necrosis of hepatocytes in high-dose group.
The antioxidative system can protect organisms from the attack of free radicals. Aerobic organisms possess antioxidative enzymes such as SOD, GSH-Px, and CAT, and superoxide radical. The antioxidative enzymes (SOD, GSH-Px, and CAT) can reduce superoxide radical, protect polyunsaturated fatty acid from lipid peroxidation, and further preserve the intact structure of the cell membrane. GSH-Px plays a vital role in the protection of cells against oxidative stress, which acts as an important water-phase non-enzymatic antioxidant and an essential cofactor for antioxidant enzymes taking part in cellular redox reactions.38 When hepatocytes are damaged, GST that mainly exists in the liver would be quickly released into the blood and lead to elevated GST activity. MDA as a biomarker of lipid peroxidation reflects the degree of cell damage.39 In general, the antioxidant enzymes would significantly decrease when the lipid peroxidation is elevated in some disease. The reason is that the increased lipid peroxidation would consume more enzymes.38 According to the hierarchical oxidative stress hypothesis, it is the tier-1 stage for oxidative stress response, and the tier-2 stage for oxidative stress response is the inflammatory response.40
Oxidative stress is the collective pathophysiological mechanism of many hepatopathies. Oxidative stress caused hepatic injury mainly by priming lipid peroxidation and destroying the enzyme activity. In addition, ROS can launch a variety of inflammatory cytokines, such as TGF-β, IL-1β, IL-6, IL-8, and TNF-α, etc., which can lead to neutrophils infiltration, reinforce and promote inflammation, eventually result in liver cell apoptosis.41 In this study, the high-dose Gd2O3:Eu3+ nanotubes induced hepatic dysfunction via oxidative stress, and the antioxidative enzymes (SOD, GSH-Px, and CAT) of high-dose group exhibited lower activities than that of the control group. The levels of ROS, MDA, and protein carbonylation also increased by high-dose Gd2O3:Eu3+ nanotubes treatment. In the meantime, the inflammatory cytokines such as IL-1β, IL-8, and TNF-α increased after treatment by high-dose Gd2O3:Eu3+ nanotubes. It was reported that the underlying mechanism of oxidative stress for hepatic injury exposure to the xenobiotics was via mitogen-activated protein kinase (MAPK) pathway. The xenobiotics could trigger oxidative stress, and the transcription of detoxification genes was preferentially activated, which include extracellular signal-regulated kinase (ERK), c-Jun NH2-terminal kinase/stress-activated protein kinase (JNK/SAPK), and p38.42,43 Once activated, ERK, JNK, and p38 phosphorylated proteins and transcription factors could result in the enhancement of the transcriptional activity of a multitude of genes.44,45
Setyawati et al. reported that Gd2O3:Tb3+ nanoparticles could induce cytotoxicity and DNA damage on human neonatal foreskin fibroblast cells in a dose-dependent way.1 Jin et al. also reported that Gd2O3:Eu3+ nanotubes inhibited the viability of bone marrow stromal cells (BMSCs) and induced cell necrosis in concentration and time-dependent manners.46 However, Gd2O3:Eu3+ nanoparticles were relatively nontoxic on rat lung epithelial cell line.13 The cytotoxic effects of Gd2O3:Eu3+ nanorods on Chang liver cells and BXPC cells were examined by using MTT assay, and the results exhibited no obvious cytotoxic effect.11 So it can be deduced that the possible reasons of toxicity differences might be involved in as follows: their size and morphologies of nanomaterials, and the cell models etc.47–49
In summary, Gd2O3:Eu3+ nanotubes have found potential applications in field of nanomedicine, which may be used as contrast agents in MRI, site-specific drug carriers for cancer therapy, X-ray tomography, and ultrasound scanner etc. At present, the experimental results indicated that they have showed some adverse bioeffects. Therefore, a thorough evaluation on their toxicity must be taken into consideration in the future. Moreover, controllable nanosurface chemistry may be used to solve adverse effects of Gd2O3:Eu3+ nanotubes before the use in biomedical field.
Conclusions
In this study, the biodistribution, subacute toxicity, and the oxidative damage mechanism of hepatic injury were studied after intraperitoneal injection with Gd2O3:Eu3+ nanotubes in mice. The results indicated that the relative organ weight of spleen in the high-dose group increased significantly. However, there was no obvious toxicity for hematological system. The ALT, AST, and ALP levels in the high-dose group increased compared with that of the control group. These changes demonstrated that the significant lesion of liver was induced by high-dose Gd2O3:Eu3+ nanotubes. The histopathological and the biodistribution results were in consistent with biochemical results. Moreover, from the changes of SOD, GSH-Px, CAT, GST, ROS, MDA, protein carbonylation, and the inflammatory cytokines, it can be deduced that the oxidative stress and inflammatory mediators may be the mechanism of liver lesions of induced by high-dose Gd2O3:Eu3+ nanotubes.
Conflict of interest
The authors declare that no competing interests exist.
Acknowledgements
This research was supported by the National Natural Science Foundations of China (20971034, 21271059, 31470961, 21301046, 51302062), the Research Fund for the Doctoral Program of Higher Education of China (20111301110004, 20131301120004), Hebei Province “Hundred Talents Program” (BR2-202), “Three Three Three” Talents Project of Hebei Province (A201401002), Training Program for Innovative Research Team and Leading Talent in Hebei Province University (LJRC024), China Postdoctoral Science Foundation Funded Project (2013M530119, 2014T70226), Key Program of Hebei University Scientific Research Project (ZD2015031), and SRF for ROCS (SEM).
References
- M. I. Setyawati, P. K. Khoo, B. H. Eng, S. Xiong, X. Zhao, G. K. Das, T. T. Tan, J. S. Loo, D. T. Leong and K. W. Ng, J. Biomed. Mater. Res., Part A, 2013, 101, 633–640 CrossRef PubMed.
- S. Y. Kim, J. K. Park, S. S. Kang, Y. C. Byung, H. C. Sung, W. S. Jung, W. S. Dae and H. N. Sang, Nucl. Instrum. Methods Phys. Res., Sect. A, 2007, 576, 70–74 CrossRef CAS PubMed.
- A. Klasson, M. Ahren, E. Hellqvist, F. Söderlind, A. Rosén, P. O. Käll, K. Uvdal and M. Engström, Contrast Media Mol. Imaging, 2008, 3, 106–111 CrossRef CAS PubMed.
- R. F. Barth and A. H. Soloway, Mol. Chem. Neuropathol., 1994, 21, 139–154 CrossRef CAS PubMed.
- M. O. Oyewumi, R. A. Yokel, M. Jay, T. Coakley and R. J. Mumper, J. Controlled Release, 2004, 95, 613–626 CrossRef CAS PubMed.
- B. Chertok, B. A. Moffat, A. E. David, F. Yu, C. Bergemann, B. D. Ross and V. C. Yang, Biomaterials, 2008, 29, 487–496 CrossRef CAS PubMed.
- S. Hu, S. Chen, D. Liu and C. S. Hsiao, Adv. Mater., 2008, 20, 2690–2695 CrossRef CAS PubMed.
- C. Plank, U. Schillinger, F. Scherer, C. Bergemann, J. S. Rémy, F. Krötz, M. Anton, J. Lausier and J. Rosenecker, Biol. Chem., 2003, 384, 737–747 CrossRef CAS PubMed.
- S. J. Son, J. Reichel, B. He, M. Schuchman and S. B. Lee, J. Am. Chem. Soc., 2005, 127, 7316–7317 CrossRef CAS PubMed.
- T. Y. Liu, S. H. Hu, D. M. Liu, S. Y. Chen and I. W. Chen, Nano Today, 2009, 4, 52–65 CrossRef CAS PubMed.
- C. Zhou, H. Wu, C. Huang, M. Wang and N. Jia, J. Controlled Release, 2014, 31, 675–684 CAS.
- N. Luo, X. Tian, C. Yang, J. Xiao, W. Hu, D. Chen and L. Li, Phys. Chem. Chem. Phys., 2013, 15, 12235–12240 RSC.
- K. Zhang, T. Holloway, M. Bahoura, A. K. Pradhan, R. Prabakaran, J. Pradhan, S. Smith, J. C. Hall, G. T. Ramesh, D. R. Sahu and J. L. Huang, Proc. SPIE, 2009, 7291, 729104–729110 CrossRef PubMed.
- S. Dixit, M. Das, S. Alwarappan, N. L. Goicochea, M. Howell, S. Mohapatra and S. Mohapatra, RSC Adv., 2013, 3, 2727–2735 RSC.
- E. Hemmer, H. Takeshita, T. Yamano, T. Fujiki, Y. Kohl, K. Löw, N. Venkatachalam, H. Hyodo, H. Kishimoto and K. Soga, J. Mater. Sci.: Mater. Med., 2012, 23, 2399–2412 CrossRef CAS PubMed.
- G. Jia, K. Liu, Y. Zheng, Y. H. Song, M. Yang and H. P. You, J. Phys. Chem. C, 2009, 113, 6050–6055 CAS.
- C. R. Patra, S. S. Abdel Moneim, E. Wang, T. Coakley and R. J. Mumper, Toxicol. Appl. Pharmacol., 2009, 240, 88–98 CrossRef CAS PubMed.
- H. Liu, C. Zhang, Y. Tan, J. Wang, K. Wang, Y. Zhao, G. Jia, Y. Hou, S. Wang and J. Zhang, J. Nanopart. Res., 2014, 16, 2303–2313 CrossRef.
- A. Ghosh and P. C. Sil, Chem.-Biol. Interact., 2009, 177, 96–106 CrossRef CAS PubMed.
- J. X. Wang, Y. B. Fan, Y. Gao, Q. H. Hu and T. C. Wang, Biomaterials, 2009, 30, 4590–4600 CrossRef CAS PubMed.
- M. M. Bradford, Anal. Biochem., 1976, 72, 248–254 CrossRef CAS.
- C. Dai, G. Cheng, Y. Dong, J. Duan, S. Wang and J. Zhang, Biol. Trace Elem. Res., 2013, 156, 188–195 CrossRef CAS PubMed.
- S. Chen, Y. Hou, G. Cheng, C. Zhang, S. Wang and J. Zhang, Biol. Trace Elem. Res., 2013, 154, 156–166 CrossRef CAS PubMed.
- J. Yang, C. Li, Z. Y. Cheng, X. M. Zhang, Z. W. Quan, C. M. Zhang and J. Lin, J. Phys. Chem. C, 2007, 111, 18148–18154 CAS.
- R. Kumar, I. Roy, T. Y. Ohulchanskky, L. A. Vathy, E. J. Bergey, M. Sajjad and P. N. Prasad, ACS Nano, 2010, 4, 699–708 CrossRef CAS PubMed.
- P. Manna, M. Ghosh, J. Ghosh, J. Das and P. C. Sil, Nanotoxicology., 2012, 6, 1–21 CrossRef CAS PubMed.
- K. K. Griendling and G. A. FitzGerald, Circulation, 2003, 108, 1912–1916 CrossRef PubMed.
- J. C. Chang, S. J. Kou, W. T. Lin and C. S. Liu, World J. Cardiol., 2010, 2, 150–159 CrossRef PubMed.
- J. K. Greisberg, J. M. Wolf, J. Wyman, L. Zou and R. M. Terek, J. Orthop. Res., 2001, 19, 797–801 CrossRef CAS.
- R. Edward and M. D. Eichner, Am. J. Med., 1979, 66, 311–320 CrossRef.
- O. R. McIntyre and F. G. Ebauch, Ann. Intern. Med., 1967, 66, 301–316 CrossRef CAS.
- V. J. Mohanraj and Y. Chen, Nanoparticles – a review, Trop. J. Pharm. Res., 2007, 5, 561–573 Search PubMed.
- M. Guo, X. Xu, X. Yan, S. Wang, S. Gao and S. Zhu, J. Hazard. Mater., 2013, 260, 780–788 CrossRef CAS PubMed.
- A. D. Abid, D. S. Anderson, G. K. Das, L. S. van Winkle and I. M. Kennedy, Part. Fibre Toxicol., 2013, 10, 1–10 CrossRef CAS PubMed.
- H. Ishiyama, M. Sato, K. Matsumura, M. Sento, K. Ogino and T. Hobara, Pharmacol. Toxicol., 1995, 77, 293–298 CAS.
- D. A. Badger, R. K. Kuester, J. M. Sauer and I. G. Sipes, Toxicology, 1997, 121, 143–153 CrossRef CAS.
- E. B. Harstad and C. D. Klaassen, Toxicol. Appl. Pharmacol., 2002, 180, 178–185 CrossRef CAS PubMed.
- S. Helmut, Free Radical Biol. Med., 1999, 27, 916–921 CrossRef.
- J. Wang, B. Chen, H. Li, H. Yu, Y. Zhao, J. Sun, Y. Li, G. Xing, H. Yuan, J. Tang, Z. Chen, H. Meng, Y. Gao, C. Ye, Z. Chai, C. Zhu, B. Ma, X. Fang and L. Wan, Biochem. Pharmacol., 2006, 71, 872–881 CrossRef CAS PubMed.
- A. Nel, T. Xia, L. Madler and N. Li, Science., 2006, 311, 622–627 CrossRef CAS PubMed.
- J. A. Sanchez-Alcazar, E. Schneider, M. A. Martinez, P. Carmona, I. Hernandez-Munoz, E. Siles, P. de la Torre, J. Ruiz-Cabello, I. Garcia and J. A. Solis-Herruzo, J. Biol. Chem., 2000, 275, 13353–13361 CrossRef CAS PubMed.
- Z. Galcheva-Garzova, B. Derijard, J. H. Wu and R. J. Davis, Science, 1994, 265, 806–808 Search PubMed.
- J. M. Kyriakis and J. Avruch, BioEssays, 1996, 18, 567–577 CrossRef CAS PubMed.
- M. Karin, J. Biol. Chem., 1995, 270, 16483–16486 CrossRef CAS PubMed.
- B. H. Yang, Q. J. Wang, R. H. Lei, C. Q. Wu, C. Shi, Q. X. Wang, Y. Yuan, Y. Wang, Y. W. Luo, Z. H. Hu, H. Z. Ma and M. Y. Liao, J. Nanosci. Nanotechnol., 2010, 10, 8527–8537 CrossRef CAS PubMed.
- Y. Jin, S. Chen, J. Duan, G. Jia and J. Zhang, J. Inorg. Biochem., 2015, 146, 28–36 CrossRef CAS PubMed.
- R. A. Sperling, E. Casals, J. Comenge, N. G. Bastús and V. F. Puntes, Curr. Drug Metab., 2009, 10, 895–904 CrossRef CAS.
- A. Verma and F. Stellacci, Small, 2010, 6, 12–21 CrossRef CAS PubMed.
- L. W. Zhang and N. A. Monteiro-Riviere, Toxicol. Sci., 2009, 110, 138–155 CrossRef CAS PubMed.
Footnote |
† These authors contributed equally to this work. |
|
This journal is © The Royal Society of Chemistry 2015 |
Click here to see how this site uses Cookies. View our privacy policy here.