DOI:
10.1039/C5RA13556G
(Paper)
RSC Adv., 2015,
5, 77996-78005
Gellan gum-coated gold nanorods: an intracellular nanosystem for bone tissue engineering†
Received
10th July 2015
, Accepted 8th September 2015
First published on 8th September 2015
Abstract
Gold nanorods (AuNRs) have emerged as an exceptional nanotool for a myriad of applications ranging from cancer therapy to tissue engineering. However, their surface modification with biocompatible and stabilizing biomaterials is crucial to allow their use in a biological environment. Herein, low-acyl gellan gum (GG) was used to coat AuNRs surface, taking advantage of its stabilizing, biocompatible and gelling features. The layer-by-layer based strategy implied the successive deposition of poly(acrylic acid), poly(allylamine hydrochloride) and GG, which allowed the formation of a GG hydrogel-like shell with 7 nm thickness around individual AuNRs. Stability studies in a wide range of pH and salt concentrations showed that the polysaccharide coating can prevent AuNRs aggregation. Moreover, a reversible pH-responsive feature of the nanoparticles was observed. Cytocompatibility and osteogenic ability of GG-coated AuNRs were also addressed. After 14 days of culturing within SaOS-2, an osteoblast-like cell line, in vitro studies revealed that AuNRs-GG exhibit no cytotoxicity, were internalized by the cells and localized inside lysosomes. AuNRs-GG combined with osteogenic media enhanced by two fold the mineralization capacity, as compared to cells exposed to osteogenic media alone. The proposed system has shown interesting features for osteogenesis, and further insights might be relevant for drug delivery, tissue engineering and regenerative medicine.
1. Introduction
Gold nanoparticles (AuNPs) have attracted a great deal of interest in the clinical realms of drug delivery, diagnosis and therapy.1,2 This is mainly due to their straightforward synthesis and easy functionalization processes, their high surface area and their non-toxic nature. In addition, their unique physicochemical properties render them an outstanding nanomaterial for a wide range of applications in regenerative medicine.3,4 However, their use in bone tissue engineering (BTE) is still in its infancy. Recently, spherical AuNPs have shown impressive features for BTE by stimulating the osteogenic differentiation of mesenchymal5,6 and adipose-derived stem cells,7 as well as primary osteoblasts.8 Moreover, their size and surface chemistry may play a role on cell behavior.7,9 Nevertheless, to date, the effect of other kinds of shapes, such as anisotropic gold nanorods, has not been investigated.
As alternative to spherical AuNPs, AuNRs exhibit two plasmon bands: transverse and longitudinal. The longitudinal plasmon band is located in the near-infrared region and is highly sensitive to the local environment, making AuNRs very promising as multimodal nanotool for diagnosis, hyperthermia and imaging.10,11 The multimodal nature of AuNRs is a cutting-edge concept in which BTE may benefit.
The direct use of as-prepared AuNRs with biological materials is highly limited. The cytotoxicity of the cetyltrimethylammonium bromide (CTAB), a surfactant used during their synthesis, and their tendency to aggregate under physiological media can both lead to cell death and limited internalization ability.12 Thus, their surface modification with biocompatible stabilizing agents is primordial for the preparation of a successful candidate. Several methods, including thiol chemistry,13,14 shell coating15,16 and layer-by-layer (LbL) coating17 have been proposed using numerous cell-friendly organic or inorganic compounds. Among them polysaccharides, natural polymers, allowed to produce biocompatible and highly stable AuNPs. Dhar et al.18 have reported the synthesis of AuNPs using gellan gum as reducing and capping agent, improving their biocompatibility and stability at varying pH and ionic strength conditions. These particles were further successfully conjugated with anticancer drugs, showing their potential to be used as drug delivery agent.19,20
Gellan gum (GG) is a non-toxic, biocompatible and biodegradable anionic heteropolysaccharide, secreted by the bacteria Sphingomonas elodea. Its molecular structure is based in one repeating unit consisting of 1,4-α-L-rahmnose, 1,3-β-D-glucose, 1,4-β-D-glucuronic acid and 1,4-bβ-D-glucose.21 In the native form, or high acyl form, two types of acyl substituents are present: acetyl and L-glyceryl.22 Low acyl GG is obtained through alkaline hydrolysis of native GG, which removes both of the acyl residues.23 Due to its gelling properties, GG is used as a food additive and has been approved by the American Food and Drug Administration (FDA) and the European Community.24 In addition, due to their mechanical properties and ability to support cell encapsulation, GG hydrogels have a great potential for TE, namely for the development of scaffolds for cartilage25–28 and intervertebral disc TE applications.29
Our work mainly addresses the surface modification of AuNRs using GG. The proposed strategy is based on the LbL approaches and involved two major steps. Firstly, a pre-coating via adsorption of two oppositely charged polyelectrolytes, poly(acrylic acid) (PAA) and poly(allylamine hydrochloride) (PAH), to allow particle stability. And afterwards, the formation of a GG shell on the gold surface. The developed nanoparticles were characterized using different techniques such as UV-Vis spectroscopy, transmission electron microscopy (TEM) and zeta potential determination. To validate our particles for further bone TE applications, in vitro studies were performed with an osteoblast-like cell line, SaOS-2. These well-characterized cells possess many osteoblastic features, such as the capability to fully differentiate similarly to osteoblastic cells, making them a widely used model.30,31 Nanoparticles cytotoxicity was evaluated by the MTS assay and cellular uptake has been confirmed by transmission electron microscopy. The effect of GG-modified AuNRs (AuNRs-GG) on the osteogenic capacity of SaOS-2 was also assessed, by evaluating both alkaline phosphatase (ALP) activity and calcium deposition, two typical markers of osteogenic differentiation.
2. Results and discussion
It is well known that as-prepared gold nanorods (AuNRs) and their propensity to aggregate under physiological conditions induces cell death and hinder their direct use for biomedical applications. Therefore, surface modification to remove the excess of toxic CTAB and to enhance their stability in solution is needed. In the present work, we report the development of biocompatible AuNRs for bone tissue engineering, taking advantage of the stabilizing, biocompatible and gelling features of gellan gum (GG).
2.1 Surface modification of gold nanorods with gellan gum
Fig. 1 schematically represents the functionalization procedure, from CTAB-coated AuNRs to final GG-coated particles based on the layer-by-layer (LbL) method. Firstly, well-dispersed AuNRs were successfully synthesized by a seed-mediated growth method,32 using CTAB as surface passivant as shown in Fig. S1A.† TEM images revealed nanorods with an average length of 47 ± 10 nm and an aspect ratio of approximately 4.7 (Fig. S1B in the ESI†).
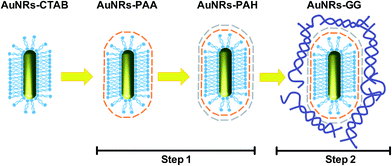 |
| Fig. 1 Schematic representation of gellan gum-based surface modification of gold nanorods. Step 1 – deposition of PAA/PAH bilayer; Step 2 – formation of gellan gum shell. | |
Before GG coating, AuNRs were covered by a thin bilayer of oppositely charged polyelectrolytes in order to improve their stability, achieved by sequential adsorption of the anionic PAA, followed by the adsorption of the cationic PAH. Finally, poly(allylamine hydrochloride)-modified gold nanorods (AuNRs-PAH) were added to a low-acyl GG solution at high temperature. The anionic nature of GG enables the adsorption of the polysaccharide to the positively charged AuNRs-PAH surface through electrostatic interactions.
Because of the immiscibility of dry GG in aqueous solution at room temperature, a 0.5% w/v GG solution was first heated up to 90 °C allowing GG dissolution. Then the suspension of AuNRs-PAH was added to the polysaccharide solution, with a final concentration of 0.25% w/v GG. The mixture was cooled down at room temperature, allowing GG configuration to change from sol into hydrogel. At high temperatures, GG has a disordered coiled conformation, and upon temperature decrease, it undergoes a thermally-reversible coil-double helix transition. Subsequently, the helices self-assemble forming junction zones, resulting in the formation of a three dimensional network.33 At this configuration, GG can exhibit a thermally reversible “weak gel” characteristic. The addition of mono- or divalent cations allows a crosslink between the helices. The interaction between the carboxylic groups of GG and the cations leads to tighter aggregation, which produces stronger gels.21 Based on this principle, we assumed that close to AuNRs surface, gelling properties of GG should be stronger than the fluid gel forming in the rest of the solution due to the interactions between the carboxylic group of GG and the amine group of the positively charged PAH. Therefore, after removing the excess of the polysaccharide, a layer of GG adsorbed onto AuNRs surfaces should be achieved.
2.1.1 Particles characterization. In order to characterize the AuNRs-GG, each step of surface modification was followed by UV-Vis spectrometry, zeta potential and TEM analyses (Fig. 2). Fig. 2A depicts the UV-Vis spectra of the freshly prepared gold nanorods (AuNRs-CTAB), AuNRs-PAH (before GG coating) and AuNRs-GG (after GG coating). The successive deposition of PAA/PAH and then GG bring the longitudinal plasmon band (LPB) maximum to be red-shifted to 6 nm and 17 nm, respectively, due to a change in the surrounding environment of particles. Moreover, after GG coating we observed the presence of a new band located at 260 nm, attributed to GG.
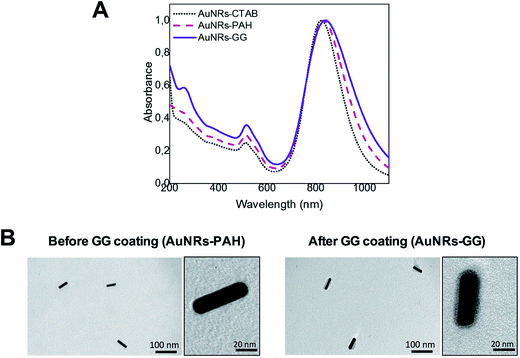 |
| Fig. 2 (A) UV-Vis spectra of AuNRs-CTAB, AuNRs-PAH and AuNRs-GG, and (B) TEM images of gold nanorods before (AuNRs-PAH) and after GG coating (AuNRs-GG). | |
The successful multilayer coating and nanoparticle stability were confirmed by means of measuring the zeta potential of AuNRs at each step of the process. The relative charge of the particles was in agreement with the net charge of each compound. The as-prepared AuNRs are positively charged with ζ = +35.9 ± 1.3 mV, due to the presence of cationic CTAB bilayer on the surface. The addition of PAA changes the surface charge from positive to negative values (ζ = −52.4 ± 3.9 mV), and it switched again to a positive value after PAH coating (ζ = +43.5 ± 2.8 mV). Finally, the nanorods acquired a negative charge of −32.8 ± 4.9 mV after coating, confirming the presence of the heteropolysaccharide on particles surface. The morphology of the AuNRs before and after GG coating was investigated under a TEM, as shown in Fig. 2B. Although the presence of the polyelectrolyte bilayer around the nanoparticles is not obvious, we clearly identified the presence of a shell for the AuNRs-GG samples, with a thickness of 6.8 ± 1.5 nm surrounding individual AuNRs (Fig. 2B). Thence, our surface modification process has shown the successful preparation of AuNRs-GG core–shell structure, where individual AuNRs were coated with GG.
2.1.2. Particles stability. When designing nanoparticles for regenerative medicine applications, their stability against ionic strength and pH needs to be considered. To assess this question, we have tackled the stability of AuNRs before and after GG capping in a range of salt concentrations up to 0.5 M and by varying the pH between 2 and 10. To evaluate and compare the stability of AuNRs-GG over polyelectrolyte-AuNRs, we followed the behavior of the LPB because of its high sensitivity to particle aggregation, resulting from a decrease of intensity magnitude, red-shift and broadness of the band (Fig. 3 and 2S†).
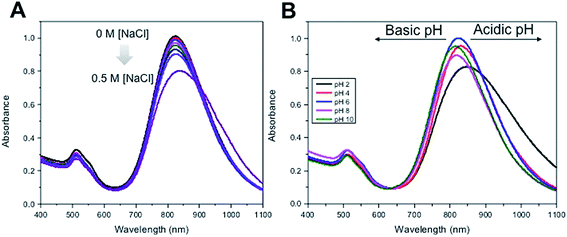 |
| Fig. 3 UV-Vis spectrometry analysis of AuNRs stability against ionic strength and pH. (A) UV-Vis spectra of AuNRs-GG solutions at 0, 0.001, 0.005, 0.01, 0.02, 0.05, 0.1 and 0.5 M of NaCl; (B) UV-Vis spectra of AuNRs-GG solutions at pH varying from 2 to 10. | |
Stability against ionic strength. The effect of the ionic strength was evaluated 24 hours after the addition of a solution of NaCl with final concentration ranging from 0 to 0.5 M. The Fig. S2A† and 3A represent the UV-Vis spectra of both AuNRs-PAH and AuNRs-GG, respectively. AuNRs-PAH remained stable up to 0.02 M. At 0.05 M, the LBP underwent a significant change (e.g. drop of intensity and broadening), arising from a large aggregation of the particles because of charge screening (Fig. S2A†). Increasing salt concentrations induced a complete precipitation of the nanorods. However, when particles were functionalized with GG, the LPB remained similar between 0 and 0.1 M, with a slight change at 0.5 M, suggesting that AuNRs were stable at higher salt concentration (0.1 M) and then at 0.5 M an aggregation starts to occur. The high molecular weight (1000 kg mol−1) of GG provides steric stability and therefore the AuNRs-GG exhibited a greater colloidal stability against salt addition as compared to nanorods functionalized with lower molecular weight polyelectrolytes (Mw = 15
000 and 5000 g mol−1 for PAH and PAA, respectively).
Stability against pH. The effect of the pH was evaluated 24 hours after the addition of a solution of HCl or NaOH to adjust the pH value to 2, 4, 6, 8 and 10. The Fig. S2B† and 3B represent the UV-Vis spectra of both AuNRs-PAH and AuNRs-GG, respectively. The aggregation and precipitation of AuNRs-PAH is evident at basic pH (8 and 10), whereas in acidic conditions (2, 4 or 6), the nanoparticles are more stable, with a higher stability at pH 6 (Fig. S2B†). These observations are related to the protonation state of PAH. As PAH exhibits a pKa around 8.6, the polyelectrolyte is positively charged due to the protonation of the amino group in acidic conditions, which maintains a colloidal stability. However, under basic pH, the neutral charge of PAH leads to a decrease of repulsion between nanoparticles and aggregation occurs.On the other hand, UV-Vis spectra measured at different pH values for AuNRs-GG, shown in Fig. 3B, revealed that no obvious aggregation occurred for a pH range from 4 to 10 since narrow LPB were observed. The LPB underwent a shift from red to blue as the pH was increased, suggesting an AuNRs-GG pH-responsiveness behavior. At pH 2, we noticed a decrease of intensity and widening of the LPB and by eye we could clearly observe particles flocculation (Fig. S3A†). Typically, when particles aggregate, the color of the suspension usually turns from red to blue. However, in our case, the color precipitates were red, suggesting that the interactions between rods were low. To verify the reversibility or irreversibility of AuNRs-GG aggregation, we increased again the pH of the solution from 2 to 6. Upon increase of the pH, LPB underwent a blue-shift and its sharpness started decreasing (Fig. S3B†). The AuNRs-GG seemed to disassemble and reversed to solution (data not shown). It is worth mention that GG is pH-sensitive34 and stable from pH values comprised between 3 and 10. As the pH is increased, the number of junction zones tends to decrease since the electrostatic repulsion between the carboxylic groups of GG is higher. On the contrary, as the pH decreases, the interactions between the GG helices enhance junction zone formation. As a consequence, basic pH leads to the formation of weaker gel, whereas acid pH results in strengthened gels compared to the one forming at neutral pH. Therefore, it can be assumed that the shift of the LPB and the reversibility of aggregation are correlated to the pH-responsiveness of GG around the AuNRs. Herein, GG not only embeds the AuNRs-PAH, but as well protects them against aggregation. In addition, AuNRs-GG has shown to be pH-sensitive, which is an interesting feature for sensing purposes.
Biological environment requires working at pH around 7–8 and high ionic strength. Therefore, the synthesized hybrid materials have shown more promising features as compared to polyelectrolytes-modified nanoparticles. These results are in agreement with Dhar and co-workers,18 where gold nanoparticles, synthesized in presence of GG as reducing and capping agent, showed good stability under pH and ionic strength conditions.
2.2. In vitro studies of AuNRs-GG for bone tissue engineering strategies
2.2.1. Cell viability and internalization of AuNRs-GG within SaOS-2 osteoblast-like cells. The cell viability and the internalization ability of the developed AuNRs-GG has been assessed in vitro, by means of using SaOS-2, a human osteoblast-like cell line commonly used as osteoblastic model.35SaOS-2 cells were maintained in the presence of the nanorods for 1, 3, 7 and 14 days of culturing. Basal media without particles (control) and basal media containing 50 pM of AuNRs-GG were changed and added every 3 days to the cells culture. As shown in Fig. 4, for all time-points, the AuNRs-GG did not affect cell viability, with values always superior to 98% in relation to the viability observed in control samples. To ascertain that AuNRs-GG were internalized by the cells, we verified their localization under TEM after 14 days of culturing. The images, as depicted in Fig. 5, revealed that AuNRs-GG were found inside cells, aggregated within multilamellar vesicles, identified as lysosomes. Previous studies suggested receptor-mediated endocytosis as the mechanism of cellular uptake for biomolecules within the size range of AuNRs.12 The internalization mechanism is based on the recognition of proteins present on the serum-containing media by receptors of cellular membrane. When included in serum-containing media, media proteins, mostly bone serum albumin,12 absorb on particles surface, changing their surface charge to values similar to the media alone. Thus, particles can be recognized by the cellular receptors and consequently be internalized.
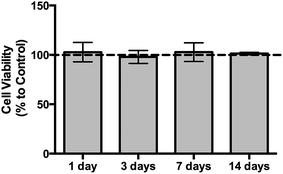 |
| Fig. 4 Cell viability of SaOS-2 after incubation with AuNRs-GG for 1, 3, 7 and 14 days. Results are expressed as average ± standard deviation (n = 9). | |
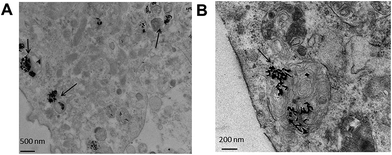 |
| Fig. 5 TEM images of SaOS-2 cytoplasm, after 14 days of culture with AuNRs-GG. (A) 25 000× magnification and (B) 60 000× magnification. Black arrows point AuNR-GG clusters. | |
2.2.2. Effect of AuNRs-GG on osteogenic differentiation of SaOS-2. To evaluate the effect of AuNRs-GG for bone tissue engineering approaches, SaOS-2 were cultured up to 21 days in basal media (BM) and osteogenic media (OM) as controls and supplemented with AuNRs-GG (50 pM) using both media (NRBM, NROM, respectively). Alkaline phosphatase (ALP) activity, and mineralization capacity, two phenotypic markers of osteogenesis, known to be enhanced within OM,8 were analyzed for each condition at days 2, 7, 14 and 21.ALP activity, an early marker for osteoblast differentiation, was determined by staining and quantification assays, as shown in Fig. 6. The staining (Fig. 6A) revealed a time-dependent increase of ALP activity for all media with higher level of expression under OM. In order to gain a deeper understanding regarding this marker, ALP activity was quantified and normalized per DNA content (Fig. 6B). A high level of activity for both basal media and osteogenic media at day 2 was observed, which is in agreement with the literature.36,37 SaOS-2 are characterized by a high ALP activity when cultured in vitro,36 with or even without β-glycerophosphate in confluent cultures.37 Moreover, ALP activity was higher when cells were cultured in the presence of osteogenic inductors, as noticed by an increase of 30% between OM and BM. However, at day 2, the ALP activity of SaOS-2 cultured in presence of AuNRs-GG (NRBM or NROM) was lower compared to their respective control media (BM or OM), as shown in Fig. 6B. Typically, during the osteogenic differentiation process, ALP activity reaches a peak and then starts to decrease due the production of calcium nodules.38,39 In our experiment, the ALP activity reached its maximum at day 7 and then dropped for all conditions, except for NRBM in which case the ALP continued its increase up to 21 days and finally reached an ALP activity closer to the one detected for BM. We can assume that in absence of osteogenic inductors (i.e. preventing any external effect in the ALP activity), the addition of AuNRs at the beginning of the cell proliferation may play a role on the ALP production/activity of SaOS-2 that, over the time, fades away. This is as well in agreement with the results obtained for NROM showing that even with lower ALP activity at day 2, the increase of ALP at day 7 is more significant as compared to the increase observed for OM, suggesting that SaOS-2 recovered and maintained their features.
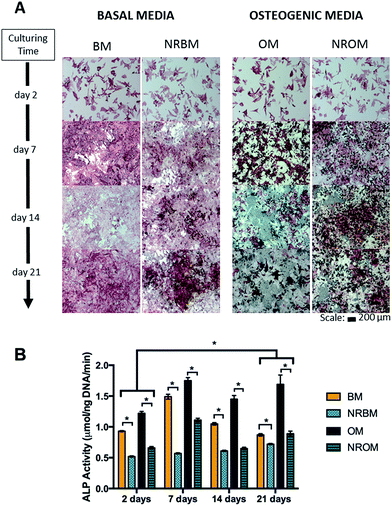 |
| Fig. 6 (A) ALP staining and (B) normalized ALP activity of SaOS-2 cultured through 2, 7, 14 and 21 days with BM, NRBM, OM and NROM. Results are expressed as average ± standard deviation (n = 9, *p < 0.05). | |
Production of mineral deposit is a key phenotypic feature of osteogenic differentiation. Herein, the mineralization was qualitatively assessed by alizarin red staining and SEM combined with EDS analysis. Calcium deposition was also quantitatively evaluated. When cultured with osteogenic inductors, SaOS-2 cells were able to produce mineralized nodules in absence or presence of nanoparticles in a time-dependent manner, as noticed by an increase and saturation of red staining (Fig. 7A). After 7 days of culture (Fig. 7A) a higher content of calcium were visualized in the presence of AuNRs-GG, suggesting that nanoparticles may promote mineralization. At days 14 and 21, the presence of black spots in higher number for NROM was observed, which were identified as mineralization nodules as depicted in Fig. 7B. These results were supported by calcium quantification for days 14 and 21. For both time-points, the calcium content was significantly higher when SaOS-2 were cultured with AuNRs-GG, as compared to the control (Fig. 7C). Concerning day 21, the calcium content was 50% higher than control samples.
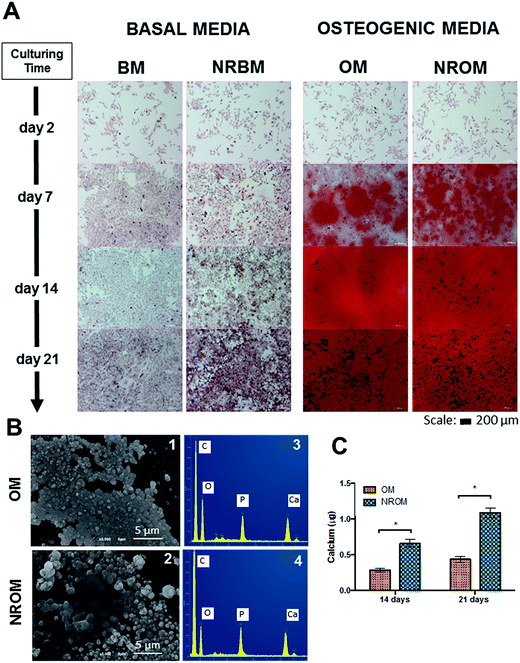 |
| Fig. 7 (A) Alizarin red staining of SaOS-2 after 2, 7, 14 and 21 days of culture with BM, NRBM, OM and NROM. (B) SEM images (1 and 2) and EDS analysis (3 and 4) of SaOS-2 cultured with OM and NROM, respectively. (C) Calcium quantification after 14 and 21 days in OM or NROM. Results are expressed as average ± standard deviation (n = 9, *p < 0.05). | |
Therefore, the results showed that AuNRs-GG supplemented in OM can enhance osteogenesis of SaOS-2 by increasing mineralization of the extracellular matrix, despite a lower ALP activity. We hypothesized that the typical high level of ALP activity found in SaOS-2 was sufficient to allow mineralization in osteogenic media conditions and that the decrease of ALP observed in NROM is not significant for inhibiting the mineralization process.
In brief, AuNRs-GG may potentiate the effect of the osteogenic media towards osteocyte-like phenotype, with concomitant reduction of ALP activity and increased mineralization capacity.38,39 Yi et al. reported that AuNPs may stimulate osteogenic differentiation of mesenchymal stem cells through a mechanical stimulus which activates p38 mitogen-activated protein kinase (MAPK) pathway.6 However, the cell proliferation, differentiation and formation of mineralized nodules in presence of spherical AuNPs have behaved differently depending on the type of cells, size, surface composition and concentrations of AuNPs. For example, human adipose-derived stem cells can differentiate faster when cultured with 30 nm and 50 nm AuNPs than the one treated with smaller (15 nm) or bigger (75 and 100 nm) AuNPs.7 In addition, Li et al. have reported that the surface chemistry on AuNPs control the human bone marrow-derived mesenchymal stem cells differentiation.40 Based of those findings, we can hypothesize that both AuNRs (47 × 10 nm) and gellan gum synergistically have a positive influence on osteogenesis. However, future studies should be performed to fully understand how AuNRs-GG interact with the osteogenic differentiation dynamics, namely the mechanisms that led to a reduction of ALP activity but to an improvement of the osteogenic media effect on mineralization.
3. Experimental
Materials
Chloroauric acid (HAuCl4), cetyltrimethylammonium bromide (CTAB), ascorbic acid, silver nitrate, poly(acrylic acid), PAA, sodium salt (Mw = 5000), poly(allylamine hydrochloride), PAH (Mw = 15
000), Gelzan™ CM Gelrite® (Mw = 1000 kg mol−1), Dulbecco's modified Eagle's medium low glucose (DMEM), phosphate buffered saline (PBS) solution, dexamethasone, β-glycerophosphate, naphthol ASMX phosphate/fast violet B salt, alizarin red S, ethylenediamine tetraacetic acid (EDTA) and diethanolamine were purchased from Sigma-Aldrich. Hydrochloric acid and sodium hydroxide were purchased from VWR. Glutaraldehyde was acquired from Electron Microscopy sciences and epon resin from TAAB. TrypLE™ express enzyme was purchased from Life Technologies. Fetal bovine serum (FBS) and antibiotic–antimycotic solution were purchased from Alfagene. Formalin was purchased from Enzifarma and ascorbic acid 2-phosphate sesquimagnesium salt hydrate from Comercial Rafer, CellTiter W 96 AQueous one solution cell proliferation assay kit was acquired from Promega, Quant-iT PicoGreen dsDNA kit from Molecular Probes Invitrogen and CA CoMe Sys1 kit from Roche. In-house distilled water was purified using a Milli-Q® direct water purification system from Millipore.
Synthesis of gold nanorods
The gold nanorods (AuNRs) were synthesized by the seed-mediated growth method.32 First, a gold seed solution was prepared by the borohydride reduction of 0.25 mM HAuCl4 in an aqueous 0.2 M CTAB solution. Subsequently, 100 μL seed solution was added to a 21.6 mL growth solution containing 0.2 M CTAB, 1.15 mM HAuCl4, 56 mM of HCl, 2.3 mM ascorbic acid and 0.23 mM silver nitrate. The solution was aged for 24 hours at 30 °C to ensure the complete formation of AuNR. CTAB excess was removed by centrifugation and particles were dispersed in Milli-Q® water. The concentration of AuNRs was determined at the longitudinal plasmon band (LPB) peak maximum using molar extinction coefficient ε = 5.109 M−1 cm−1.41
Pre-coating of polyelectrolytes through layer-by-layer
Purified particles were modified using a layer-by-layer approach.42 Briefly, a solution of 1.60 nM of particles was added, drop-wise and under vigorous stirring, to an aqueous solution of 4 g L−1 of anionic PAA with 6 mM NaCl. PAA adsorption was allowed to proceed for 2 hours, at room temperature (RT) and under stirring. PAA excess was removed by centrifugation and particles were re-dispersed in Milli-Q® water. The process was repeated with a solution of 4 g L−1 of cationic PAH with 6 mM NaCl. After, PAA/PAH-coated gold nanorods were twice washed by centrifugation.
Gellan gum coating
Low acyl gellan gum (Gelzan™ CM Gelrite®, GG) solution was prepared with a concentration of 0.5% (w/v), in ultra-pure water. To allow its dissolution, GG solution was heated up to 90 °C, under vigorous stirring. Then, PAA/PAH-modified AuNRs were added drop-wise, and the solution was continuously heated and stirred for 5 minutes. The mixture was cooled down at room temperature and gently stirred overnight. The solution was freed of excess of gellan gum by two consecutive centrifugations, and re-dispersed in purified water. The final concentration of GG-coated gold nanorods was adjusted to 0.3 nM for the in vitro assays and the particles were stable at least for 6 month in the aqueous solution.
Particles stability
Stability under different electrolytic concentrations was evaluated by addition of a NaCl solution to AuNRs-PAH and AuNRs-GG. Fixed quantities of 2 M NaCl were added to 2 mL solutions of each particles type, in order to have the desired final salt concentrations of 0.001, 0.005, 0.01, 0.02, 0.05, 0.1 and 0.5 M. Solutions were properly mixed and left overnight at room temperature. UV-Vis spectra were acquired 24 hours after salt addition and normalized for further comparison.
In order to evaluate particles stability at different pH values comprised between 2 and 10, fixed amounts of HCl, for pH below 6, or NaOH, for pH above 6, were added to 2 mL of particles solution. Solutions were properly mixed and left overnight at room temperature. UV-Vis spectra were acquired 24 hours after pH modifications.
Characterizations
Ultraviolet-visible spectra (UV-Vis) were collected from 1100 to 200 nm wavelength and absorbance was determined using a UV-Vis spectrophotometer (Shimadzu, UV-1601). Surface potential was measured with a Zetasizer (Malvern, NanoZS). All the samples were read three times and surface charge was calculated using the Smoluchowski equation. Transmission electron microscopy (TEM) was used to confirm particles rod-shape and the GG coating. Samples were prepared by placing a drop of each type of particles solution above a copper grid and letting it dry completely, under a light source. TEM images were acquired using JEOL JEM 1400 TEM (Tokyo, Japan). Images were digitally recorded using a CCD digital camera Orious 1100W Tokyo, Japan. Scanning electron microscopy (SEM), combined with energy dispersive spectrometry (EDS) analyses were performed using a scanning electron microscope (JSM-6010LV, JEOL, Japan) equipped with an energy dispersive spectroscope (INCAx-Act, PentaFET Precision, Oxford Instruments, UK), at low vacuum (70 Pa) and 15 kV.
Cell culture
SaOS-2 cells (sarcoma osteogenic) were cultured in basal medium (BM) composed of low-glucose Dulbecco's modified eagle medium (DMEM) supplemented with 10% v/v fetal bovine serum (FBS) and 1% v/v antibiotic/antimycotic. Cultures were maintained until confluence at 37 °C under humidified atmosphere of 5% CO2 in air, with culture medium being changed twice a week.
Cell viability
Assessment of the potential effect of AuNRs on cell viability was performed using a standard 3-(4,5-dimethylthiazol-2-yl)-5(3-carboxymethoxyphenyl)-2(4-sulfofenyl)-2H-tetrazolium (MTS) viability test. All materials were processed under sterile conditions. Particles sterilization was achieved by passing the different solutions through a 0.22 μm filter. The MTS viability test on SaOS-2
43 was performed after contact of cells with AuNRs-GG.
Cells were grown as monolayers as described above. After several passages, confluent cells were detached from the flasks using TrypLE™ express enzyme and a diluted suspension was centrifuged at 1200 rpm for 5 minutes. SaOS-2 cells were seeded in each well of a 24-well tissue culture polystyrene plate (three replicates per sample) at a density of 25 × 103 cells per well and cultured for 24 hours at 37 °C in a 5% CO2 atmosphere. Then, AuNRs-GG were added to each well, making up a final concentration of 50 pM. The medium was changed every three days, followed by an addition of new particles. Cells cultured without particles supplementation were used as a control. The MTS test was performed after 1, 3, 7 and 14 days of culture at 37 °C and 5% CO2 atmosphere. Culture medium was removed and cells were washed twice with PBS at pH 7.4. Subsequently, 350 mL of a mixture containing serum-free culture medium without phenol red and MTS solution from CellTiter W 96 AQueous one solution cell proliferation assay kit, at a ratio of 5
:
1 were added to each well. Optical density (OD) was measured at 490 nm using a microplate reader (Synergy HT, Biotek) after 3 hours incubation at 37 °C and 5% CO2 atmosphere. The percentage of cell viability was calculated by normalization with the mean OD value obtained for the control. All tests were performed in triplicate (n = 9).
Cell internalization of AuNRs-GG
SaOS-2 cells were cultured in the same conditions used for MTS assay, but on the top of TCPS cover slips. After each time point, cell culture medium was removed and cells were washed twice with PBS buffer. Then, cells were fixed with 10% formalin for 15 minutes, at room temperature. After, formalin was removed; cells were washed with PBS and stored in PBS, protected from light at 4 °C, until processing. Samples were fixed in 2% glutaraldehyde and 4% paraformaldehyde in cacodylate buffer 0.1 M (pH 7.4), dehydrated and embedded in epon resin. Ultrathin sections (40–60 nm thickness) were prepared on a Leica Reichert SuperNOVA ultramicrotome (Germany) using diamond knives (DDK, Wilmington, DE, USA). The sections were mounted on 200 mesh copper or nickel grids, stained with uranyl acetate and lead citrate for 15 minutes each, and examined under a JEOL JEM 1400 TEM (Tokyo, Japan). Images were digitally recorded using a CCD digital camera (Orious 1100W Tokyo, Japan).
Effect of AuNRs-GG on osteogenic differentiation
To study the effect of AuNRs-GG in osteogenic differentiation, cells were seeded at 20
000 cells per well in 24-well tissue culture plates in BM. After 24 hours of culture, the medium was renewed to BM or osteogenic medium (hereafter designed OM) composed of BM with 100 nM dexamethasone, 10 mM β-glycerophosphate and 0.05 mM ascorbic acid 2-phosphate sesquimagnesium salt hydrate. Finally, AuNR-GG were added at the final concentration of 50 pM to each medium BM, and OM (hereafter designated NPBM and NPOM, respectively), and sterilized by filtration (0.22 μm). BM and OM without AuNRs were used as controls. Culture media were changed twice a week. At days 2, 7, 14 and 21 cells were recovered and the following assays were performed.
Immunocytochemistry
To assess cell differentiation alkaline phosphatase (ALP) and alizarin red S stainings were performed. First, cells were fixed with 10% formalin for 15 minutes at RT. For ALP staining, cells were then incubated in naphthol ASMX phosphate/fast violet B salt for 30 minutes at 37 °C. In the case of alizarin red S staining, cells were incubated for 5 minutes in a solution of 2% (w/v) of alizarin red S in distilled water, pH 4.3. Images were obtained using Stemi 2000-C stereoscope for both stainings.
ALP activity and calcium quantification
To quantify the effect of AuNRs in osteogenic differentiation, ALP activity and calcium were measured. For that purpose, cells were previously lysed in Milli-Q water for 1 hour at 37 °C followed of 1 h at −80 °C. In the case of ALP quantification, lysed cells were incubated with 0.2% w/v p-nitrophenyl phosphate in 1 M diethanolamine for 1 hour at 37 °C. The reaction was stopped using a solution of 2 M NaOH/0.2 mM EDTA. Absorbance was read at 405 nm in a microplate reader (Synergy HT, Bio-Tek) and converted into product concentrations using a calibration curve built with p-nitrophenol standard. ALP activity was normalized to double-stranded DNA (dsDNA) using the Quant-iT PicoGreen dsDNA kit accordingly with manufacturer's instructions. For calcium quantification, CA CoMe Sys1 kit was used accordingly with manufacturer's instructions. Briefly, HCl 6 M was added to lysed cells, incubated for 5 minutes at RT with R1 component, followed by 2 minutes at 37 °C with R2 component and the absorbance was read at 570 nm in a microplate reader (Synergy HT, Bio-Tek). After, the absorbance was converted in mass of calcium present using a calibration curve of CaCl2.
Statistical analysis and software
Statistical analysis was performed using GraphPad Prism 6 software. Cell viability data, ALP activity and calcium quantity were averaged and mean ± standard deviation was plotted. Statistical significance was determined by a two-tailed t-test. The critical level of statistical significance chosen was p < 0.05.
4. Conclusion
Herein, we have reported the surface modification of gold nanorods using a natural and biocompatible low-acyl gellan gum. Based on a layer-by-layer approach, AuNRs were covered by a gellan gum shell with a thickness around 7 nm. The presence of high molecular weight gellan gum around the gold nanorods significantly enhanced stability in solution at different pH values and ionic strengths as compared to nanorods modified with polyelectrolytes. In vitro studies using a relevant osteoblast-like cell line revealed that AuNRs-GG were not cytotoxic after 14 days of culturing and were localized inside lysosomes. Moreover, AuNRs-GG supplemented in osteogenic media promoted an increase of mineralization of the osteoblast-like cells, despite a decrease of the ALP activity. The proposed system has shown interesting features for osteogenesis, and further insights might be achieved using stem cells, and relevant 3D tissue engineering models. The enhanced stability and biocompatibility of AuNRs-GG paves the way for their future application in the biomedical field, namely for bone tissue engineering purposes.
Acknowledgements
The authors would like to thank QREN (ON.2 – NORTE-01-0124-FEDER-000018) co-financed by North Portugal Regional Operational Program (ON.2 – O Novo Norte), under the National Strategic Reference Framework (NSRF), through the European Regional Development Fund (ERDF) for providing financial support to this project. The Portuguese Foundation for Science and Technology (FCT) distinction attributed to J. M. Oliveira under the FCT Investigator program (IF/00423/2012) is also greatly acknowledged. Sílvia Vieira was awarded a FCT PhD scholarship (SFRH/BD/102710/2014). The authors also thank the financial support provided under the ERC funded project ComplexiTE (grant ERC-2012-ADG_20120216-321266).
References
- Y. S. Zhang, Y. Wang, L. Wang, Y. Wang, X. Cai, C. Zhang and L. V. Wang, Theranostics, 2013, 3, 532–543 CrossRef PubMed.
- Y.-C. Yeh, B. Creran and V. M. Rotello, Nanoscale, 2012, 4, 1871–1880 RSC.
- S. Verma, A. J. Domb and N. Kumar, Nanomedicine, 2011, 6, 157–181 CrossRef CAS PubMed.
- G. G. Walmsley, A. McArdle, R. Tevlin, A. Momeni, D. Atashroo, M. S. Hu, A. H. Feroze, V. W. Wong, P. H. Lorenz, M. T. Longaker and D. C. Wan, Nanomedicine, 2015, 1253–1263 CrossRef CAS PubMed.
- D. N. Heo, W.-K. Ko, M. S. Bae, J. B. Lee, D.-W. Lee, W. Byun, C. H. Lee, E.-C. Kim, B.-Y. Jung and I. K. Kwon, J. Mater. Chem. B, 2014, 2, 1584–1593 RSC.
- C. Yi, D. Liu, C.-C. Fong, J. Zhang and M. Yang, ACS Nano, 2010, 4, 6439–6448 CrossRef CAS PubMed.
- W.-K. Ko, D. N. Heo, H.-J. Moon, S. J. Lee, M. S. Bae, J. B. Lee, I.-C. Sun, H. B. Jeon, H. K. Park and I. K. Kwon, J. Colloid Interface Sci., 2015, 438, 68–76 CrossRef CAS PubMed.
- D. Zhang, D. Liu, J. Zhang, C. Fong and M. Yang, Mater. Sci. Eng., C, 2014, 42, 70–77 CrossRef CAS PubMed.
- J. J. Li, N. Kawazoe and G. Chen, Biomaterials, 2015, 54, 226–236 CrossRef CAS PubMed.
- L. M. Liz-marzán, J. Pérez-juste and I. Pastoriza-santos, Media, 2008, 103–111 Search PubMed.
- Z. Zhang, J. Wang and C. Chen, Theranostics, 2013, 3, 223–238 CrossRef CAS PubMed.
- A. M. Alkilany, P. K. Nagaria, C. R. Hexel, T. J. Shaw, C. J. Murphy and M. D. Wyatt, Small, 2009, 5, 701–708 CrossRef CAS PubMed.
- J. Choi, J. Yang, D. Bang, J. Park, J.-S. Suh, Y.-M. Huh and S. Haam, Small, 2012, 8, 746–753 CrossRef CAS PubMed.
- X. Qiu, Y. Wang, Y. Cui, Z. Sun, R. Liu, W. Huang, F. Zhao, Y. Zu, H. Yuan, X. Chang and X. Gao, J. Nanosci. Nanotechnol., 2012, 12, 6893–6899 CrossRef CAS PubMed.
- A. S. Monem, N. Elbialy and N. Mohamed, Int. J. Pharm., 2014, 470, 1–7 CrossRef CAS PubMed.
- S. Shen, H. Tang, X. Zhang, J. Ren, Z. Pang, D. Wang, H. Gao, Y. Qian, X. Jiang and W. Yang, Biomaterials, 2013, 34, 3150–3158 CrossRef CAS PubMed.
- L. Wang, X. Jiang, Y. Ji, R. Bai, Y. Zhao, X. Wu and C. Chen, Nanoscale, 2013, 5, 8384–8391 RSC.
- S. Dhar, E. M. Reddy, A. Shiras, V. Pokharkar and B. L. V. Prasad, Chem.–Eur. J., 2008, 14, 10244–10250 CrossRef CAS PubMed.
- S. Dhar, V. Mali, S. Bodhankar, A. Shiras, B. L. V Prasad and V. Pokharkar, J. Appl. Toxicol., 2011, 31, 411–420 CrossRef CAS PubMed.
- S. Dhar, E. M. Reddy, A. Prabhune, V. Pokharkar, A. Shiras and B. L. V Prasad, Nanoscale, 2011, 3, 575–580 RSC.
- L. Gasperini, J. F. Mano and R. L. Reis, J. R. Soc., Interface, 2014, 11, 20140817 CrossRef PubMed.
- R. Chandrasekaran, A. Radha and V. G. Thailambal, Carbohydr. Res., 1992, 224, 1–17 CrossRef CAS.
- K. S. Kang, G. T. Veeder, P. J. Mirrasoul, T. Kaneko and I. W. Cottrell, Appl. Environ. Microbiol., 1982, 43, 1086–1091 CAS.
- T. Osmałek, A. Froelich and S. Tasarek, Int. J. Pharm., 2014, 466, 328–340 CrossRef PubMed.
- J. Silva-Correia, V. Miranda-Gonçalves, A. J. Salgado, N. Sousa, J. M. Oliveira, R. M. Reis and R. L. Reis, Tissue Eng., Part A, 2012, 18, 1203–1212 CrossRef CAS PubMed.
- J. T. Oliveira, T. C. Santos, L. Martins, M. A. Silva, A. P. Marques, A. G. Castro, N. M. Neves and R. L. Reis, J. Tissue Eng. Regener. Med., 2009, 3, 493–500 CrossRef CAS PubMed.
- J. Silva-Correia, J. M. Oliveira, S. G. Caridade, J. T. Oliveira, R. A. Sousa, J. F. Mano and R. L. Reis, J. Tissue Eng. Regener. Med., 2011, 5, e97–107 CrossRef CAS PubMed.
- J. T. Oliveira, L. Martins, R. Picciochi, P. B. Malafaya, R. A. Sousa, N. M. Neves, J. F. Mano and R. L. Reis, J. Biomed. Mater. Res., 2010, 93, 852–863 CAS.
- S. Pina, J. M. Oliveira and R. L. Reis, Adv. Mater., 2015, 27, 1143–1169 CrossRef CAS PubMed.
- S. B. Rodan, Y. Imai, M. A. Thiede, G. Wesolowski, D. Thompson, Z. Bar-Shavit, S. Shull, K. Mann and G. A. Rodan, Cancer Res., 1987, 47, 4961–4966 CAS.
- M. Prideaux, A. R. Wijenayaka, D. D. Kumarasinghe, R. T. Ormsby, A. Evdokiou, D. M. Findlay and G. J. Atkins, Calcif. Tissue Int., 2014, 95, 183–193 CrossRef CAS PubMed.
- B. Nikoobakht and M. A. El-Sayed, Chem. Mater., 2003, 15, 1957–1962 CrossRef CAS.
- R. Chandrasekaran, R. P. Millane, S. Arnott and E. D. T. Atkins, Carbohydr. Res., 1988, 175, 1–15 CrossRef CAS.
- C. S. F. Picone and R. L. Cunha, Carbohydr. Polym., 2011, 84, 662–668 CrossRef CAS PubMed.
- C. Pautke, M. Schieker, T. Tischer, A. Kolk, P. Neth, W. Mutschler and S. Milz, Anticancer Res., 2004, 24, 3743–3748 Search PubMed.
- E. Murray, D. Provvedini, D. Curran, B. Catherwood, H. Sussman and S. Manolagas, J. Bone Miner. Res., 1987, 2, 231–238 CrossRef CAS PubMed.
- D. J. McQuillan, M. D. Richardson and J. F. Bateman, Bone, 1995, 16, 415–426 CAS.
- G. S. Stein, J. B. Lian, A. J. van Wijnen, J. L. Stein, M. Montecino, A. Javed, S. K. Zaidi, D. W. Young, J.-Y. Choi and S. M. Pockwinse, Oncogene, 2004, 23, 4315–4329 CrossRef CAS PubMed.
- A. Neve, A. Corrado and F. P. Cantatore, Cell Tissue Res., 2011, 343, 289–302 CrossRef CAS PubMed.
- J. J. Li, N. Kawazoe and G. Chen, Biomaterials, 2015, 54, 226–236 CrossRef CAS PubMed.
- C. J. Orendorff and C. J. Murphy, J. Phys. Chem. B, 2006, 110, 3990–3994 CrossRef CAS PubMed.
- A. Gole and C. J. Murphy, Chem. Mater., 2005, 17, 1325–1330 CrossRef CAS.
- A. J. Salgado, J. E. Figueiredo, O. P. Coutinho and R. L. Reis, J. Mater. Sci.: Mater. Med., 2005, 16, 267–275 CrossRef CAS PubMed.
Footnote |
† Electronic supplementary information (ESI) available. See DOI: 10.1039/c5ra13556g |
|
This journal is © The Royal Society of Chemistry 2015 |