DOI:
10.1039/C5RA13503F
(Paper)
RSC Adv., 2015,
5, 73892-73900
Improving nutraceutical bioavailability using mixed colloidal delivery systems: lipid nanoparticles increase tangeretin bioaccessibility and absorption from tangeretin-loaded zein nanoparticles
Received
10th July 2015
, Accepted 26th August 2015
First published on 26th August 2015
Abstract
The objective of this study was to evaluate the influence of dietary lipids on the gastrointestinal fate of tangeretin-loaded zein nanoparticles. The zein delivery systems were mixed with different amounts of oil-in-water emulsions to represent varying levels of digestible fat in the diet, and then passed through a simulated gastrointestinal tract model. Tangeretin bioaccessibility increased with increasing fat content due to enhanced solubilization within the mixed micelles formed by lipid digestion products (fatty acids and monoacylglycerols) in the intestinal fluids. Indeed, the tangeretin concentration in the micelle phase was about twelve times higher in the presence of fat droplets than in their absence. The intestinal epithelium absorption study indicated that tangeretin permeability across the model epithelium cells also increased with increasing fat content. This study suggests that utilizing mixed colloidal systems consisting of both lipid nanoparticles and protein nanoparticles may promote the bioavailability of hydrophobic bioactive agents.
1. Introduction
Tangeretin represents a class of polymethoxy flavonoids (PMFs) found almost exclusively in citrus fruits, and particularly the peels of sweet and mandarin oranges.1 PMFs have been shown to exhibit a variety of potential benefits for human health, such as enhancing biochemical events in mammalian cells,2 reducing serum triacylglycerol, very low-density lipoprotein (VLDL), and low-density lipoprotein (LDL) levels.3 PMFs can also inhibit the proliferation of certain types of cancer cells, such as lung, colon and breast cancer cells.4 PMFs have also been demonstrated to modulate the liver and heart function of hypercholesterolemic rats.5
However the oral bioavailability of tangeretin is currently limited due to its poor water solubility, which is related to the presence of numerous methoxyl groups on the flavone backbone.6 We have previously shown that zein nanoparticles and microparticles offer a suitable means of encapsulating and delivering tangeretin.7 Colloidal particles can be formed from zein using antisolvent precipitation due to its high solubility in alcohol solutions but low solubility in water.8 The zein and active component are dissolved in an alcohol solution, which is then injected into water, resulting in the spontaneous formation of zein particles containing the active component. This method has been successfully used to encapsulate lipids, such as fish oil, flax oil, and essential oils.9
The purpose of the current study was to examine the impact of lipid nanoparticles on the potential gastrointestinal fate of tangeretin-loaded zein nanoparticles. It is well known that co-ingestion of digestible lipids can increase the oral bioavailability of lipophilic nutraceuticals and pharmaceuticals by altering their bioaccessibility, absorption, or transformation within the gastrointestinal tract.10 Lipids may enhance the bioavailability of lipophilic molecules through a variety of mechanisms, including stimulating the secretion of digestive juices, increasing gastrointestinal transit times, enhancing their solubility within intestinal fluids through mixed micelle formation, increasing the permeability of the epithelium monolayer, controlling chemical or biochemical transformation, or altering the absorption route (portal vein versus lymphatic system). Recent study showed that the bioefficacy of tangeretin against cancer cells were significantly improved when they were administered in emulsion.11 Both in vitro and in vivo studies indicated the bioavailability of tangeretin was increased when incorporated in to lipid nanoemulsion.12 We therefore hypothesized that mixing tangeretin-loaded zein nanoparticles with digestible lipid nanoparticles would enhance the bioavailability of the lipophilic tangeretin molecules. The influence of lipids on the potential biological fate of the tangeretin was studied using a simulated gastrointestinal model that included mouth, stomach, and small intestine phases, combined with a Caco-2 cell model to study permeability.6
An important aim of this study was to highlight the potential advantages of using combination delivery systems containing a mixture of different types of colloidal particles (in this case zein and lipid nanoparticles) rather than using single types of colloidal particle. This information may be useful in the rational design of oral delivery systems for food and pharmaceutical applications.
2. Materials and methods
2.1. Materials
Tangeretin powder with a purity of 98.4% was obtained from Bepharm Ltd. (Shanghai, China). β-Lactoglobulin (with a purity of 92.5%) was obtained from Davisco Foods International (lot JE 002-8-415, Le Sueur, MN). Corn oil was obtained from a local supermarket. Zein (purity 92%, w/w), bile extract (porcine, B8613), porcine pancreas (type II, triacylglycerol hydrolase E.C. 3.1.1.1, PPL), Hank's balance salts (cat. no. H1387) and uranyl acetate were purchased from Sigma-Aldrich (St Louis, MO). Pepsin (CAS: 9001-75-6), sodium chloride (NaCl), sodium hydroxide (NaOH), calcium chloride (CaCl2), hydrochloric acid (HCl), HPLC grade methanol, tetrahydrofuran (THF), trifluoroacetic acid (TFA), acetonitrile (ACN) were obtained from Fisher Scientific (Fairlawn, NJ, USA). Ammonium acetate was obtained from EMD Chemicals Inc. (Gibbstown, NJ, USA). Double distilled water was made from a water purification system (Model D14031, Barnstead Nanopure water system, Dubuque, Iowa, USA). DMEM (Dulbecco's Modification of Eagle's Medium) and non-essential amino acid was purchased from Mediatech Inc., (Manassas, VA). HEPES was purchased from Acros Organics (Geel, Belgium).
2.2. Preparation of tangeretin-loaded zein nanoparticles
The tangeretin-loaded zein nanoparticles were made using an antisolvent precipitation method described previously,13 with some slight modifications. The particles were prepared by injecting an organic phase into an aqueous phase. The organic phase consisted of zein and tangeretin (25
:
1, w/w) dissolved in 90% ethanol solution, while the aqueous phase consisted of β-lactoglobulin (3%, w/v) dissolved in PBS (10 mM, pH 7). The zein nanoparticles were formed spontaneously when the organic phase was injected into the aqueous phase (1
:
3, v/v) dropwise under constant stirring at 1000 rpm (Corning Stirrer PC-420, Corning Inc., USA). The ethanol in the mixture was then evaporated with using a vacuum rotary evaporator (Rotavapor R110, Buchi Crop., Switzerland). Then the sample was freeze dried (VirTis Genesis Lyophilizer, Virtis genesis company inc., USA) and kept in a refrigerator prior to further use.
2.3. Preparation of lipid nanoparticles
β-Lactoglobulin was dissolved in 10 mM phosphate buffer (3%, w/w) and stirred for at least 2 hours to ensure full hydration. A coarse emulsion was prepared by mixing 20% corn oil with aqueous solution using a hand blender (M133/1280, Biospec Products, Inc., ESGC, Switzerland) for 2 min. This coarse emulsion was then passed through a high pressure homogenizer (Microfluidics M-110Y, Newton, MA) at 12
000 psi for 3 times.
2.4. Particle size and ζ-potential measurement
The particle size and ζ-potential of the nanoparticles were determined using a commercial dynamic light scattering and micro-electrophoresis device (Nano-ZS, Malvern Instruments, Worcestershire, UK). The samples were diluted 10 times in PBS buffer solutions (pH 7) at the same pH as the samples being analyzed at room temperature before measurement. The particle size is reported as the intensity-weighted ('Z-average') mean particle diameter, while the particle charge is reported as the ζ-potential.
2.5. Microstructure & visual observations
The microstructure of the colloidal delivery systems was observed using an optical microscope (Nikon Eclipse E400, Nikon Corp., Japan), and the resulting images were acquired using digital image processing software (Micro Video Instruments Inc., Avon, MA). Selected samples were also analyzed using transmission electron microscopy (JEOL JEM-2000FX, JEOL USA, Inc., MA, USA). The general appearance of the colloidal systems and digesta after different gastrointestinal stages were recorded by taking images using a digital camera (Powershot SD1300IS, Canon).
2.6. Potential gastrointestinal fate of tangeretin-loaded zein nanoparticles
An in vitro digestion model was used to study the potential behavior of the delivery systems under simulated gastrointestinal tract14 conditions. Experiments were carried out at different lipid contents by mixing the tangeretin-loaded zein nanoparticles with lipid nanoparticles to simulate different diet compositions (i.e. high versus low fat diets).
Different delivery systems were prepared by mixing the freshly prepared tangeretin-loaded zein nanoparticles and different amounts of stock nanoemulsion and phosphate buffer solution so that they differed only in oil content (0%, 2%, and 4%). The samples were then passed through a simulated GIT similar to that described earlier,15 but with some slight modifications.
Mouth phase. Oral conditions were mimicked by mixing the delivery systems with a simulated saliva fluid (SSF), which was prepared from various salts and mucin as described previously.16 Delivery systems were mixed with SSF at a 1
:
1 volume ratio, and then the resulting mixture was adjusted to pH 6.8 and shaken continuously at 100 rpm in an incubator at 37 °C for 10 min (Innova Incubator Shaker, Model 4080, New Brunswick Scientific, New Jersey, USA).
Stomach phase. Simulated gastric fluid was prepared by mixing 2 g of NaCl and 7 ml of concentrated HCl and then making the volume up to 1 l using distilled water.16 Pepsin was then dissolved in this mixture (0.32%, w/v), the pH was adjusted to 1.2, and the sample from the mouth phase was mixed with it at a 1
:
1 volume ration. The resulting mixture was then adjusted to pH 2.5 and incubated at 100 rpm and 37 °C for 2 h.
Small intestine. Small intestine conditions were simulated using a pH-stat automatic titration unit (Metrohm, USA Inc.). The sample collected from the stomach phase was placed in a container placed in a 37 °C water bath. The pH was adjusted to pH 7.0. Bile salt (187.5 mg in 4 ml pH 7 PBS buffer solution) was then added, and the pH was adjusted back to 7.0. Calcium chloride solution (110 mg dissolved in 1 ml double distilled water) was then added and again the pH was adjusted to 7.0. Finally, freshly prepared pancreatin lipase (60 mg lipase in 2.5 ml pH 7.0 PBS) was added to the solution. At the same time, the automatic titration of pH-stat was started. The free fatty acid released during digestion was calculated using the following equation:17 |
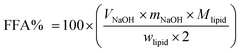 | (1) |
here, VNaOH is the volume of sodium hydroxide solution required to neutralize the free fatty acids (and other sources of H+) released during digestion; mNaOH is the molar concentration of sodium hydroxide solution, which is 0.25 M in this study; Mlipid is the molecular weight of corn oil, which is 800 g mol−1; wlipid is the amount of oil in the reaction system.
2.7. Bioaccessibility determination
The samples obtained after in vitro digestion were collected and centrifuged (Centrifuge 5417R, Eppendorf co., Hamburg, Germany) (20
200 g, 14
000 rpm) at 4 °C for 40 min. The clear micelle phase on the top layer of the samples was filtered through a 220 nm syringe filter (EMD Millipore, Billerica, MA) and analyzed for the tangeretin content using the HPLC method described before.18 The bioaccessibility was then calculated using the following equation: |
 | (2) |
here, Cmicelle is the concentration of tangeretin in the filtered micelle phase, and Craw digesta is the concentration of tangeretin in the raw digesta.
2.8. Cytotoxicity measurement of micelle phase
Caco-2 cells were seeded in 96-well plates at a density of 20
000 cells per well in 200 μl complete DMEM media (10% FBS, 1% antibiotic, 1% non-essential amino acid). After 24 h, cells were treated with different concentrations of micelle phase diluted with serum complete media. Tangeretin dissolved in DMSO was used as a control group and the final DMSO concentration in the medium was less than 1%. After incubation for 24 h, cells were analyzed using the 3-(4,5-dimethylthiazol-2-yl)-2,5-diphenyltetrazolium bromide (MTT) assay. Media in each well was replaced by 100 μl freshly prepared MTT solution (0.5 mg ml−1 dissolved in DMEM media). After 2 h incubation at 37 °C, MTT solution was dumped and the reduced formazan dye was solubilized by adding 100 μl of DMSO to each well. After gentle mixing, the absorbance was monitored at 570 nm using a plate reader (TECAN, Phenix Research Products, Candler, NC, USA).19
2.9. Tangeretin permeability determination using Caco-2 monolayers
Caco-2 cell monolayer maintenance and permeability determination was conducted as described before.20 Caco-2 cells were seeded on transwell permeable supports containing 0.4 μm polycarbonate membranes (Corning Incorporated, Corning, NY) at a seeding density of 2.6 × 105 cells per cm2. The medium was changed 16 h after seeding. The media in the apical and basolateral compartments were changed every other day. This process was maintained for about 21 days until the transendothelial electrical resistance of the filter was about 260 Ω cm2.
The micelle phases were collected after in vitro digestion and filtered through a 220 nm membrane. They were then diluted with Hank's Balanced Salt (HBSS, pH 7.4) to obtain tangeretin concentrations suitable for permeability determinations. Tangeretin dissolved in DMSO was used as a control group. All the solutions used in this experiment were pre-warmed in a 37 °C water bath. The Caco-2 monolayer transwell was incubated with HBSS for 30 min before the experiment. Then both the apical and basolateral compartments were rinsed twice with HBSS. An aliquot (1.5 ml) of each sample was added to the apical compartment and 2.5 ml pre-warmed HBSS was added to the basolateral compartment. Then, this plate was placed in an incubator. Every 30 min, 200 μl of solution was withdrawn from the apical compartment without adding new sample and 100 μl of sample was taken from the basolateral compartment and replaced by same amount of pre-warmed HBSS. The whole experiment duration was 2 hours. The transendothelial electrical resistance were monitored every time before and after the experiment and before each sampling time. The concentration of tangeretin in each sample were analyzed using HPLC method. The apparent permeability coefficient (cm s−1) was calculated with the following equation:
|
Papp = (dQ/dt)(1/(AC0))
| (3) |
d
Q/d
t is the steady-state flux (μmol s
−1),
A is the surface of the filter (4.67 cm
2),
C0 is the concentration of tangeretin added to apical compartment of each well (μM).
2.10. Data analysis
All data in this study are expressed as mean ± SD. Student's t-test was used to determine the significance of difference between two groups. One way ANOVA was used to analyze the significance of difference for more than three groups. 5% significance level was used for all tests.
3. Results and discussion
3.1. Characterization of delivery systems
In this study, mixed delivery systems were prepared that contained a combination of tangeretin-loaded zein nanoparticles and digestible lipid nanoparticles. Knowledge of the initial characteristics of these nanoparticles is important to understand their subsequent behavior in the simulated GIT. We therefore measured the size, charge, and morphology of the particles using light scattering, electrophoresis, and microscopy. The mean particle diameters of the freshly prepared nanoemulsions, tangeretin-loaded zein nanoparticles, and their mixture were 197, 248, and 215 nm respectively (Fig. 1). The ζ-potentials of the initial lipid nanoparticles (−29 mV), tangeretin-loaded zein nanoparticles (−25 mV) and mixed system (−52 mV) were all highly negative. The transmission electron microscopy (TEM) measurements suggested that the zein nanoparticles (smooth surfaces) and lipid nanoparticles (crinkly surfaces) existed separately in the mixture (Fig. 1), which can be attributed to the strong electrostatic repulsion between them. We confirmed the nature of the different kinds of nanoparticles in the mixed systems by taking TEM images of samples containing only lipid nanoparticles which had crinkly surfaces and only zein nanoparticles which had smooth surfaces (data not shown). The crinkly appearance of the lipid nanoparticles is probably due to crystallization of the uranyl acetate dye on their surfaces during sample preparation for electron microscopy. Optical microscopy images of the mixed delivery systems confirmed that they had good stability to aggregation, with no evidence of large particles in the system (Fig. 2a). The colloidal delivery systems all had good stability to gravitational separation (creaming or sedimentation), as demonstrated by the fact that no phase separation was observed after 24 hours incubation, which can be attributed to their small initial particle size and stability to aggregation.
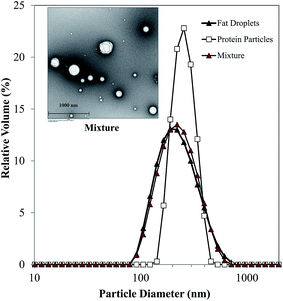 |
| Fig. 1 Particle size distribution of fat droplets, protein nanoparticles, and the mixed system. The inset shows a transmission electron microscopy image of the mixed system. | |
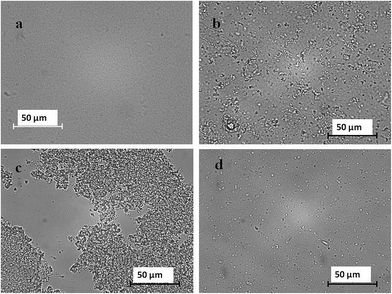 |
| Fig. 2 Microscopic image of initial delivery system (a) and delivery system after digestion in (b) mouth, (c) stomach, (d) small intestine. | |
3.2. Gastrointestinal fate of colloidal delivery systems
Colloidal delivery systems encounter a series of physicochemical and physiological environments as they pass through the various stages of the human GIT, such as changes in pH, ionic strength, agitation, enzyme activities, and surface active agents.21 We therefore measured changes in the properties of mixed colloidal delivery systems (containing 2% fat) as they passed through the simulated mouth, stomach, and small intestine phases of the GIT model.
Mouth. The mean diameter of the particles in the system increased from around 215 to 424 nm after incubation in the simulated saliva fluids (Fig. 3a), which suggests that some particle aggregation occurred. This observation was supported by the optical microscopy images, which clearly showed evidence of extensive particle aggregation (Fig. 2b). The origin of this effect can be attributed to particle flocculation induced by the presence of mucin in the artificial saliva. Mucin is a large glycoprotein that can promote particle aggregation under oral conditions through both bridging and depletion mechanisms.22 The magnitude of the negative charge on the particles decreased when they moved from the initial to the mouth phases (Fig. 3b), which can be attributed to electrostatic screening effects by salts in the simulated saliva, as well as to possible adsorption of mucin molecules to the lipid droplet surfaces.
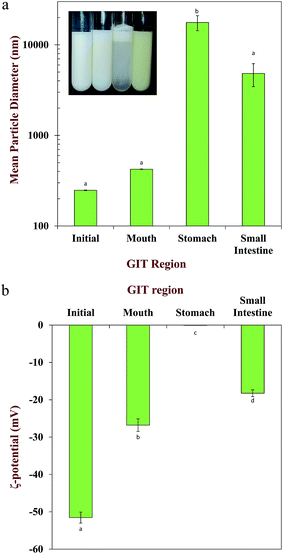 |
| Fig. 3 a) Mean particle diameters and appearances of mixed systems initially containing protein nanoparticles and lipid nanoparticles (2% oil) after passage through various stages of a simulated gastrointestinal tract (GIT). The inset shows the appearance of the system at different digestion stages (means with different letters are significantly different, p < 0.05). (b) Mean particle charges of mixed systems initially containing protein nanoparticles and lipid nanoparticles (2% oil) after passage through various stages of a simulated gastrointestinal tract (GIT). The inset shows the appearance of the system at different digestion stages (means with different letters are significantly different, p < 0.05). | |
Stomach. There was a large increase in the mean particle diameter measured by light scattering (Fig. 3a) and evidence of extensive particle aggregation in the optical microscopy images (Fig. 2c) when the samples moved from the mouth to the stomach phases. A number of different physicochemical phenomena may contribute to the instability of the nanoparticles within the gastric environment. First, both the zein and lipid nanoparticles used in this study were coated with a globular protein (β-lactoglobulin) that has an isoelectric point around pH 5. Consequently, the nanoparticles will have passed through a point of zero charge when they moved from the simulated mouth (pH 7) to the simulated gastric (pH 2) fluids, which may have promoted irreversible aggregation due to a reduction in electrostatic repulsion. Second, anionic mucin molecules from the saliva may have caused bridging flocculation of the cationic protein-coated nanoparticles in the stomach. Third, the relatively high ionic strength of the gastric fluids may have reduced any electrostatic repulsion between the nanoparticles leading to aggregation. Fourth, hydrolysis of the β-lactoglobulin coating around the nanoparticles by digestive enzymes (pepsin) may have reduced their aggregation stability. The electrical charge on the nanoparticles in the stomach was close to zero (Fig. 3b). One might expect nanoparticles coated by β-lactoglobulin to be strongly positively charged at pH 2 because this is well below their isoelectric point. The fact that the charge was near zero may have been because anionic mucin adsorbed to the droplet surfaces, thereby neutralizing some of the positive charge from the proteins. In addition, some of the surface proteins may have been digested by the pepsin, which would have altered the surface charge. The droplets in the emulsion were highly unstable to gravitational separation (Fig. 3a) under gastric conditions, which can be attributed to the relatively large particle size caused by droplet aggregation. Interestingly, we did not observe a sediment layer in these systems suggesting that the zein nanoparticles associated with the lipid nanoparticles and the overall density of the flocs formed was less than that of water.
Small intestine. The mean particle diameter remained relatively large after incubation in the small intestinal fluids (Fig. 3a), but the large aggregates formed within the gastric fluids appeared to have largely dissociated (Fig. 2d). In the presence of pancreatin, the triacylglycerol molecules in the lipid droplets will be converted to a monoacylglycerol23 and two free fatty acids (FFAs) by pancreatic lipase. These MAG and FFAs will combine with phospholipids and bile salts to form mixed micelles (micelles and vesicles) that can solubilize lipophilic compounds, and then carry them through the mucous layer to the small intestine cell surfaces.10a The pancreatic lipase used in this study was a crude extract that also has protease activity. Consequently, the β-lactoglobulin and zein in the protein nanoparticles would have been fully or partially hydrolyzed, thereby releasing the tangeretin in the intestinal fluids. As a result, the digesta is likely to contain a complex mixture of different types of colloidal particles, including undigested lipid particles, undigested protein particles, micelles, vesicles, and insoluble matter. The high negative charge on the particles in these samples can therefore be attributed to the anionic nature of the free fatty acids, bile salts, phospholipids, and proteins at neutral pH conditions (Fig. 3b).
3.3. Digestibility of colloidal delivery systems
In this section, we used the pH-stat method to measure the digestion of the various colloidal delivery systems. As mentioned earlier, the crude pancreatic lipase extract used in this study has both lipase and protease activity, and therefore we would expect both the proteins and lipids to be digested. The amount of alkaline solution required to maintain the solution at pH 7.0 throughout the digestion period was measured (Fig. 4a), and then this information was used to calculate the percentage of free fatty acids (FFA) released from the samples containing lipid nanoparticles (Fig. 4b). Prior to calculating the FFAs for these samples, the volume of alkaline solution titrated into the lipid-free solutions was subtracted to take into account changes in pH induced by protein digestion.
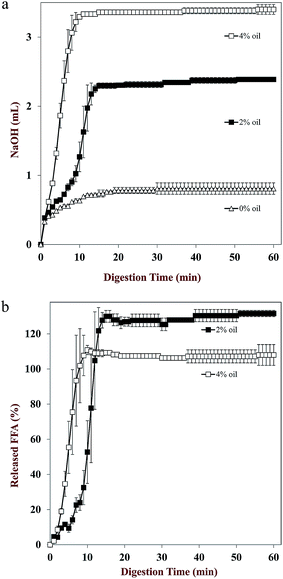 |
| Fig. 4 a) pH-Stat titration curves carried out under simulated small intestinal conditions for mixed colloidal delivery systems containing tangeretin-loaded zein nanoparticles and different concentrations of lipid nanoparticles (0 to 4% oil). (b) Calculated free fatty acids released under simulated small intestinal conditions for mixed colloidal delivery systems containing tangeretin-loaded zein nanoparticles and different initial concentrations of lipid nanoparticles (2 or 4% oil). | |
The volume of alkaline solution titrated into the samples increased rapidly during the first few minutes of digestion, and then increased more gradually at longer digestion times (Fig. 4a). The fact that an appreciable increase in volume was observed for the system containing no lipids can be attributed to the hydrolysis of proteins (β-lactoglobulin and/or zein). For both systems containing lipid nanoparticles, there was a rapid initial increase in FFAs released during the first 10 minutes, and then a relatively constant value was reached at longer times (Fig. 4b). These results suggest that the lipid phases were fully digested within the small intestine stage, thereby leading to the formation of free fatty acids and monoacylglycerols that could form mixed micelles to solubilize the tangeretin. The fact that the delivery system initially containing 4% oil had twice as much digestible lipid as the one containing 2% oil would be expected to lead to more mixed micelles.
3.4. Tangeretin bioaccessibility
The amount of a lipophilic bioactive component solubilized within the mixed micelle phase is normally taken as a measure of its bioaccessibility.24 We therefore analyzed the amount of tangeretin in the mixed micelle phase obtained by centrifuging the digested sample collected at the end of the GIT model. Normally after centrifugation, the digested sample has three layers: a creamy layer at the top containing any undigested lipid; a clear layer at the middle containing the mixed micelles; and a pellet at the bottom containing dense insoluble materials, such as calcium soaps, undigested proteins, and precipitated compounds.10a In our study, all of the fat in the delivery systems was fully digested (Fig. 4b), and so only two layers were observed: the mixed micelle phase and the pellet.
The bioaccessibility of the tangeretin increased as the concentration of co-ingested lipid phase increased (Fig. 5), being around 15, 26, and 37% for delivery systems initially containing 0, 2 and 4% oil. As mentioned earlier, this effect can be attributed to the increased level of mixed micelles available to solubilize any lipophilic tangeretin molecules released from the digested zein nanoparticles.25 At the highest fat content used in this study, the tangeretin concentration in the micelle phase was determined to be 12.4 ± 1.8 μM, while the saturation concentration of tangeretin in pure water is around 0.93 ± 0.02 μM, which clearly shows that the digested lipids were able to greatly increase tangeretin solubilization in the aqueous intestinal fluids.
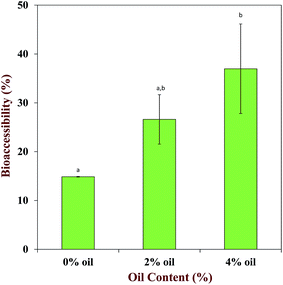 |
| Fig. 5 Influence of initial oil content on tangeretin bioaccessibility in mixed colloidal delivery systems containing tangeretin-loaded zein nanoparticles and lipid nanoparticles (means with different letters are significantly different, p < 0.05). | |
Further information about the properties of the mixed micelle phase was obtained by analyzing the particle size using dynamic light scattering. The mean diameters of the particles in the micelle phase for delivery systems initially containing 0, 2 or 4% oil were 61.5, 69.1 and 112.5 nm, respectively. In the absence of fat, these particles may have been micelles formed by the bile salts and phospholipids in the simulated small intestinal fluids. In addition, there may have been other forms of particles present in this phase, including protein nanoparticles that were not fully digested. In the presence of fat, the increase in particle size may have been due to the presence of mixed micelles consisting of bile salts, phospholipids, free fatty acids, and monoacylglycerols. The mixed micelle phase resulting from lipid digestion typically contains a combination of small micelles and large vesicle structures.26 These mixed micelles are able to transfer the lipophilic bioactive components across the mucous layer so that they can be absorbed by epithelium cells.10a,27
3.5. Cytotoxicity of tangeretin on Caco-2 cells
When performing cell permeability studies it is important to ensure that the material being tested does not appreciably alter cell viability. An MTT assay was therefore carried out to establish the potential toxicity of the mixed micelle phases collected from the simulated GIT on the Caco-2 cell monolayers. Tangeretin dissolved within DMSO did not significantly decrease cell viability over a relatively wide concentration range (Fig. 6), e.g., at 0.1 μM almost all the cells were alive, and even at 20 μM the cell viability was still around 80%. Prior to analysis the mixed micelle phases were diluted with buffer solution to obtain a range of tangeretin concentrations. Consequently, both the tangeretin and mixed micelle concentrations in these samples varied. Our results suggest that the mixed micelles had an appreciable impact on cell viability, since when compared at the same tangeretin concentrations they caused more decrease in cell viability than the control (Fig. 6). For example, cell viability was close to 100% at 0.1 μM tangeretin (0.1% v/v mixed micelle phase), but only 20% at 20 μM tangeretin (20% v/v mixed micelle phase). This effect may be caused by increased levels of bile salts in the system, since it has previously been reported that these surface active lipids promote cell death by binding to the mitochondrial membrane, which causes loss of cytochrome activity and mitochondrial membrane potential.28 For this reason, we used a tangeretin concentration of 5 μM in our permeability studies to ensure that the mixed micelles did not promote a significant decline in Caco-2 cell viability.
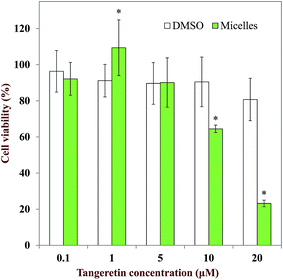 |
| Fig. 6 Influence of tangeretin dissolved in DMSO or mixed micelles on Caco-2 cell viability. Cells were seeded at 20 000 cells per well on a 96 well plate 24 hours before treatment. The samples were diluted using serum complete media to obtain a range of tangeretin concentrations (*p < 0.05). | |
3.6. Permeability of digested tangeretin micelle on Caco-2 cell monolayer
Tangeretin concentration used for this study was 5 μM based on our MTT result. As shown in Fig. 7a, Caco-2 cell monolayer integrity was well maintained during the two hour treatment. During The permeability of the Caco-2 cells increased with increasing fat content in the mixed colloidal dispersions, as demonstrated by the faster rate of tangeretin loss from the apical side of the cells for the samples initially containing 4% oil (Fig. 7b). In addition, the calculated apparent permeability coefficients (Papp) of the tangeretin were (18.1 ± 4.5) × 10−6, (17.1 ± 1.8) × 10−6, (19.3 ± 2.0) × 10−6, and (26.8 ± 1.8) × 10−6 cm s−1 for the control group, micelles from 0% oil, micelles from 2% oil, and micelles from 4% oil, respectively (Table 1). It has been reported that a substance has a high permeability when its Papp is greater than 10 × 10−6 cm s−1,6 and so all the samples analyzed in this study had high permeability. Nevertheless, the permeability of tangeretin did increase with increasing oil content in the initial delivery system, which can be partly attributed to the ability of the mixed micelles formed by lipid digestion products to increase the intestinal solubility of tangeretin.10a,c In addition, free fatty acids and emulsifiers are known to enhance intestinal permeability by increasing the dimensions of the tight junctions between epithelium cells.29
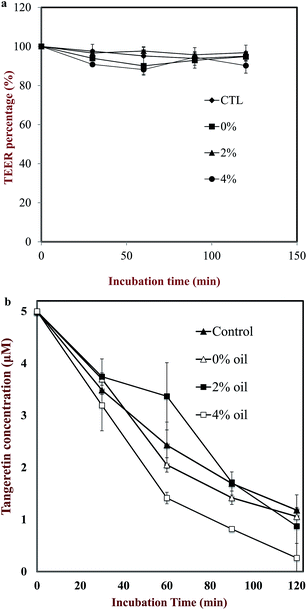 |
| Fig. 7 a) TEER percentage of each sample at different time. (b) Tangeretin concentration in the apical compartment of Caco-2 transwell cells at different times for different delivery systems. Control: tangeretin dissolved in HBSS; 0% oil: digested phase from tangeretin nanoparticles with no oil; 2%: digested phase from tangeretin nanoparticles mixed with 2% oil; 4%: digested phase from tangeretin nanoparticles mixed with 4% oil. The oil was delivered as lipid nanoparticles. | |
Table 1 Caco-2 monolayer permeability of digested phases from delivery systems initially containing tangeretin nanoparticles and different oil contents (delivered in the form of lipid nanoparticles). The control consisted of tangeretin dissolved in DMSOa
Delivery system |
Papp (×10−6 cm s−1) |
Means with different letters are significantly different, p < 0.05. |
Control |
18.1 ± 4.5a |
0% oil |
17.1 ± 1.8a |
2% oil |
19.3 ± 2.0a |
4% oil |
26.8 ± 1.8b |
4. Conclusions
This study shows that the bioavailability of tangeretin encapsulated within zein nanoparticles can be increased by mixing them with lipid nanoparticles. This result supports the notion that mixed delivery systems containing combinations of different kinds of nanoparticles may have advantages over single-nanoparticle systems. One kind of nanoparticle may be designed to encapsulate and protect the bioactive agent in a product during storage, whereas the other kind of nanoparticle is designed to increase its bioavailability within the gastrointestinal tract.
Light scattering and microscopy measurements showed that mixed colloidal delivery systems could be successfully prepared containing a combination of tangeretin-loaded zein nanoparticles and lipid nanoparticles. These systems appeared to be stable to aggregation and gravitational separation due to their high particle charge and small particle size. When these mixed colloidal delivery systems were passed through a simulated GIT the protein nanoparticles are digested by proteases thereby releasing the tangeretin molecules, whereas the lipid nanoparticles are digested by pancreatic lipases thereby forming mixed micelles. In the absence of lipid digestion products, some of the tangeretin molecules would have been solubilized in simple micelles formed by bile salts and phospholipids, whereas the rest may have formed crystals that remained in the insoluble matter. Conversely, in the presence of lipid digestion products (free fatty acids and monoacylglycerols) the solubilization capacity of the small intestinal fluids for the tangeretin molecules is increased. The absorption of the tangeretin by Caco-2 monolayers also increased in the presence of lipid nanoparticles, which was attributed to a higher level of tangeretin in the micelle phase, and a possible increase in cell permeability. The results of this study have important implications for the design and fabrication of colloidal delivery systems to increase the bioavailability of hydrophobic nutraceuticals and pharmaceuticals.
Acknowledgements
This material was partly based upon work supported by the Cooperative State Research, Extension, Education Service, USDA, Massachusetts Agricultural Experiment Station (Project No. 831) and USDA, NRI Grants (2011-03539, 2013-03795, 2011-67021, and 2014-67021) and an NSF of China grant (31428017). This project was also partly funded by the Deanship of Scientific Research (DSR), King Abdulaziz University, Jeddah, under grant numbers 330-130-1435-DSR, 299-130-1435-DSR, 87-130-35-HiCi. The authors, therefore, acknowledge with thanks DSR technical and financial support.
References
- H. Xiao, C. S. Yang, S. Li, H. Jin, C.-T. Ho and T. Patel, Monodemethylated polymethoxyflavones from sweet orange (Citrus sinensis) peel Inhibit growth of human lung cancer cells by apoptosis, Mol. Nutr. Food Res., 2009, 53(3), 398–406 CAS.
- S. P. Singh, Wahajuddin, D. Tewari, K. Patel and G. K. Jain, Permeability determination and pharmacokinetic study of nobiletin in rat plasma and brain by validated high-performance liquid chromatography method, Fitoterapia, 2011, 82(8), 1206–1214 CrossRef CAS PubMed.
- E. M. Kurowska and J. A. Manthey, Hypolipidemic Effects and Absorption of Citrus Polymethoxylated Flavones in Hamsters with Diet-Induced Hypercholesterolemia, J. Agric. Food Chem., 2004, 52(10), 2879–2886 CrossRef CAS PubMed.
-
(a) P. Qiu, P. Dong, H. Guan, S. Li, C.-T. Ho, M.-H. Pan, D. J. McClements and H. Xiao, Inhibitory effects of 5-hydroxy polymethoxyflavones on colon cancer cells, Mol. Nutr. Food Res., 2010, 54(S2), S244–S252 CrossRef CAS PubMed;
(b) M.-H. Pan, Y.-S. Lai, C.-S. Lai, Y.-J. Wang, S. Li, C.-Y. Lo, S. Dushenkov and C.-T. Ho, 5-Hydroxy-3,6,7,8,3′,4′-hexamethoxyflavone Induces Apoptosis through Reactive Oxygen Species Production, Growth Arrest and DNA Damage-Inducible Gene 153 Expression, and Caspase Activation in Human Leukemia Cells, J. Agric. Food Chem., 2007, 55(13), 5081–5091 CrossRef CAS PubMed;
(c) I. N. Sergeev, S. Li, J. Colby, C.-T. Ho and S. Dushenkov, Polymethoxylated flavones induce Ca2+-mediated apoptosis in breast cancer cells, Life Sci., 2006, 80(3), 245–253 CrossRef CAS PubMed.
- C. O. Green, A. O. Wheatley, D. A. McGrowder, L. L. Dilworth and H. N. Asemota, Citrus peel polymethoxylated flavones extract modulates liver and heart function parameters in diet induced hypercholesterolemic rats, Food Chem. Toxicol., 2013, 51, 306–309 CrossRef CAS PubMed.
- S. Li, M.-H. Pan, C.-Y. Lo, D. Tan, Y. Wang, F. Shahidi and C.-T. Ho, Chemistry and health effects of polymethoxyflavones and hydroxylated polymethoxyflavones, J. Funct. Foods, 2009, 1(1), 2–12 CrossRef CAS PubMed.
- J. Chen, J. Zheng, D. J. McClements and H. Xiao, Tangeretin-loaded protein nanoparticles fabricated from zein/β-lactoglobulin: preparation, characterization, and functional performance, Food Chem., 2014, 158, 466–472 CrossRef CAS PubMed.
- I. J. Joye and D. J. McClements, Production of nanoparticles by anti-solvent precipitation for use in food systems, Trends Food Sci. Technol., 2013, 34(2), 109–123 CrossRef CAS PubMed.
-
(a) S. Quispe-Condori, M. D. A. Saldaña and F. Temelli, Microencapsulation of flax oil with zein using spray and freeze drying, LWT--Food Sci. Technol., 2011, 44(9), 1880–1887 CrossRef CAS PubMed;
(b) Y. Wu, Y. Luo and Q. Wang, Antioxidant and antimicrobial properties of essential oils encapsulated in zein nanoparticles prepared by liquid–liquid dispersion method, LWT--Food Sci. Technol., 2012, 48(2), 283–290 CrossRef CAS PubMed;
(c) Q. Zhong and M. Jin, Zein nanoparticles produced by liquid–liquid dispersion, Food Hydrocolloids, 2009, 23(8), 2380–2387 CrossRef CAS PubMed.
-
(a) C. J. Porter, N. L. Trevaskis and W. N. Charman, Lipids and lipid-based formulations: optimizing the oral delivery of lipophilic drugs, Nat. Rev. Drug Discovery, 2007, 6(3), 231–248 CrossRef CAS PubMed;
(b) D. J. McClements and H. Xiao, Excipient foods: designing food matrices that improve the oral bioavailability of pharmaceuticals and nutraceuticals, Food Funct., 2014, 5(7), 1320–1333 RSC;
(c) D. J. McClements, Utilizing food effects to overcome challenges in delivery of lipophilic bioactives: structural design of medical and functional foods, Expert Opin. Drug Delivery, 2013, 10(12), 1621–1632 CrossRef CAS PubMed.
- Y. Ting, Y.-S. Chiou, M.-H. Pan, C.-T. Ho and Q. Huang, In vitro and in vivo anti-cancer activity of tangeretin against colorectal
cancer was enhanced by emulsion-based delivery system, J. Funct. Foods, 2015, 15, 264–273 CrossRef CAS PubMed.
- Y. Ting, Y. Jiang, Y. Lan, C. Xia, Z. Lin, M. A. Rogers and Q. Huang, Viscoelastic Emulsion Improved the Bioaccessibility and Oral Bioavailability of Crystalline Compound: A Mechanistic Study Using In Vitro and In Vivo Models, Mol. Pharm., 2015, 12(7), 2229–2236 CrossRef CAS PubMed.
- N. Parris, H. Cooke Peter, A. Moreau Robert and B. Hicks Kevin, Encapsulation of Essential Oils in Zein Nanospherical Particles, in New Delivery Systems for Controlled Drug Release from Naturally Occurring Materials, American Chemical Society: 2008; vol. 992, pp. 175–192 Search PubMed.
- J. Ø. Christensen, K. Schultz, B. Mollgaard, H. G. Kristensen and A. Mullertz, Solubilisation of poorly water-soluble drugs during in vitro lipolysis of medium- and long-chain triacylglycerols, Eur. J. Pharm. Sci., 2004, 23(3), 287–296 CrossRef CAS PubMed.
- Y. Li and D. J. McClements, Controlling lipid digestion by encapsulation of protein-stabilized lipid droplets within alginate–chitosan complex coacervates, Food Hydrocolloids, 2011, 25(5), 1025–1033 CrossRef CAS PubMed.
- A. Sarkar, K. K. T. Goh and H. Singh, Colloidal stability and interactions of milk-protein-stabilized emulsions in an artificial saliva, Food Hydrocolloids, 2009, 23(5), 1270–1278 CrossRef CAS PubMed.
- Y. Li and D. J. McClements, New Mathematical Model for Interpreting pH-Stat Digestion Profiles: Impact of Lipid Droplet Characteristics on In Vitro Digestibility, J. Agric. Food Chem., 2010, 58(13), 8085–8092 CrossRef CAS PubMed.
- P. Dong, P. Qiu, Y. Zhu, S. Li, C.-T. Ho, D. J. McClements and H. Xiao, Simultaneous determination of four 5-hydroxy polymethoxyflavones by reversed-phase high performance liquid chromatography with electrochemical detection, J. Chromatogr. A, 2010, 1217(5), 642–647 CrossRef CAS PubMed.
- M. Horie, H. Kato, K. Fujita, S. Endoh and H. Iwahashi, In Vitro Evaluation of Cellular Response Induced by Manufactured Nanoparticles, Chem. Res. Toxicol., 2011, 25(3), 605–619 CrossRef PubMed.
- H. Ina, G. E. R. Eva and A. Per, Determination of drug permeability and prediction of drug absorption in Caco-2 monolayers, Nat. Protoc., 2007, 2(9), 2111–2119 CrossRef PubMed.
- D. J. McClements, Crystals and crystallization in oil-in-water emulsions: Implications for emulsion-based delivery systems, Adv. Colloid Interface Sci., 2012, 174, 1–30 CrossRef CAS PubMed.
- M. H. Vingerhoeds, T. B. J. Blijdenstein, F. D. Zoet and G. A. van Aken, Emulsion flocculation induced by saliva and mucin, Food Hydrocolloids, 2005, 19(5), 915–922 CrossRef CAS PubMed.
- F. Cuyckens and M. Claeys, Mass spectrometry in the structural analysis of flavonoids, J. Mass Spectrom., 2004, 39(1), 1–15 CrossRef CAS PubMed.
- D. J. McClements, F. Li and H. Xiao, The Nutraceutical Bioavailability Classification Scheme: Classifying Nutraceuticals According to Factors Limiting their Oral Bioavailability, Annu. Rev. Food Sci. Technol., 2015, 6, 299–327 CrossRef CAS PubMed.
- S. K. Thakkar, B. Maziya-Dixon, A. G. Dixon and M. L. Failla, Beta-carotene micellarization during in vitro digestion and uptake by Caco-2 cells is directly proportional to beta-carotene content in different genotypes of cassava, J. Nutr., 2007, 137(10), 2229–2233 CAS.
- S. Phan, S. Salentinig, E. Gilbert, T. A. Darwish, A. Hawley, R. Nixon-Luke, G. Bryant and B. J. Boyd, Disposition and crystallization of saturated fatty acid in mixed micelles of relevance to lipid digestion, J. Colloid Interface Sci., 2015, 449, 160–166 CrossRef CAS PubMed.
- G. Lafitte, K. Thuresson and O. Soderman, Diffusion of nutrients molecules and model drug carriers through mucin layer investigated by magnetic resonance imaging with chemical shift resolution, J. Pharm. Sci., 2007, 96(2), 258–263 CrossRef CAS PubMed.
- S. Schulz, S. Schmitt, R. Wimmer, M. Aichler, S. Eisenhofer, J. Lichtmannegger, C. Eberhagen, R. Artmann, F. Tookos, A. Walch, D. Krappmann, C. Brenner, C. Rust and H. Zischka, Progressive stages of mitochondrial destruction caused by cell toxic bile salts, Biochim. Biophys. Acta, Biomembr., 2013, 1828(9), 2121–2133 CrossRef CAS PubMed.
- D. Ulluwishewa, R. C. Anderson, W. C. McNabb, P. J. Moughan, J. M. Wells and N. C. Roy, Regulation of tight junction permeability by intestinal bacteria and dietary components, J. Nutr., 2011, 141(5), 769–776 CrossRef CAS PubMed.
Footnote |
† These authors contributed equally to this manuscript. |
|
This journal is © The Royal Society of Chemistry 2015 |