DOI:
10.1039/C5RA12686J
(Paper)
RSC Adv., 2015,
5, 81356-81361
Two reversible transformable mercury(II) coordination polymers as efficient adsorbents for removal of dibenzothiophene†
Received
30th June 2015
, Accepted 11th September 2015
First published on 14th September 2015
Abstract
Three new compounds of Hg(II) have been synthesized, [Hg(quinoxaline)2(NO2)2] (1), [Hg3(μ-quinoxaline)2(μ-SCN)6]n (2) and [Hg(μ-quinoxaline)(μ-CN)2]n (3) through the reaction between a quinoxaline ligand and mercury(II) salts which have then been analyzed using IR spectroscopy and thermal gravimetric (TGA) analysis. Furthermore, these compounds have also been characterized using single-crystal X-ray crystallography. The single-crystal X-ray data of compound 1 shows the complex to be a monomer of mercury(II). The structural studies of compounds 2 and 3 show that these structures are two- and three-dimensional coordination polymers of mercury(II). Under mild reaction conditions, the mentioned compounds exhibited high efficiency and reusability in desulfurization removal of model oil which was prepared by dissolving dibenzothiophene in n-hexane. Furthermore, the DBT removal in the solution obeys first order reaction kinetics and the activation energy of the adsorption process was found to be 16.57 kJ mol−1. Finally, solid state crystal to crystal transformation of these compounds was investigated.
Introduction
Crystal engineering – the planning and construction of crystalline supramolecular architectures from modular building blocks – permits the rational design of functional molecular materials that exhibit technologically useful behavior1–7 such as conductivity and superconductivity, ferromagnetism, and nonlinear optical properties. To date, many supramolecular synthons (defined as structural units which can be formed or assembled by known or conceivable synthetic operations involving intermolecular interaction)8–11 were designed and recognized to organize molecules into one-, two-, or three-dimensional networks.12–16 Many attempts have been made to prepare a variety of transitional metal complexes using different spacers, and their structures and properties have been physically and chemically determined.17–21
Various types of bridging ligands have been used for the construction of coordination polymers.22–24 In contrast to coordination polymers of transition metal ions, formation of polymers with heavy metal ions such as mercury(II) seems to be surprisingly sparse, and, despite attractive properties of mercury(II) compounds in terms of their potential applications in paper industry, paints, cosmetics, preservatives, thermometers, manometers, fluorescent lamps and mercury batteries25 (although somehow limited due to its toxicity), there have been until recently only very few reports on Hg(II) complexes with rigid or flexible organic nitrogen donor-based ligands.26–28
The removal of sulfur from petroleum prior to its combustion could lead to considerable reduction in the emissions of acid rain precursors. Consequently, it constitutes an environmental problem; however, deep sulfur cleaning of most oils requires more than physical processes since they remove only part of the organic sulfur.29,30 To reduce diesel engine's harmful emissions, many countries have issued environmental regulation on the specifications of sulfur level in oil to below 10 ppm.31 Dibenzothiophene (DBT) in fuel oil can be easily removed subsequently by conventional separation operations (e.g. distillation, solvent extraction, bio-desulfurization, oxidation and adsorption) because their properties are significantly different from those of the hydrocarbons that constitute most of the fuel oils.32
In this study, we synthesized three new compounds of Hg(II) with N-donor ligand, quinoxaline and the possibility of solid state crystal to crystal transformation of these compounds has also been investigated. The compounds were applied in removal of dibenzothiophene from a model oil containing 500 mg L−1 of sulfur.
Experimental section
Materials and physical techniques
All reagents for the synthesis and analysis were commercially available from Aldrich and Merck Company and used as received. Melting points were measured on an Electrothermal 9100 apparatus. IR spectra were recorded using Thermo Nicolet IR 100 FT-IR. The thermal behaviour was measured with a PL-STA 1500 apparatus with the rate of 10 °C min−1 in a static atmosphere of argon. X-ray powder diffraction (XRD) measurements were performed using a Philips X'pert diffractometer with mono chromated Cu-Kα radiation.
Crystallographic measurements were made at 298(2) K for 1 using Bruker APEX area-detector, MoKα radiation (λ = 0.71073 Å). Data collection for 2 was performed at 100(2) K on a Bruker APEX II CCD area detector with graphite-monochromated Mo Kα radiation (λ = 0.71073 Å). For 3, data collection was performed at 180(2) K on a Bruker SMART APEX II X-ray diffractometer with graphite-monochromated Mo Kα radiation (λ = 0.71073 Å), operating at 50 kV and 30 mA over 2θ ranges of 4.32–52.00°. No significant decay was observed during the data collection.
The structures were solved by direct methods and refined by refinement of F2 against all refiections. Structure solution and refinement were accomplished using SIR97, SHELXL97 and WinGX.33,34
Synthesis of [Hg(quinoxaline)2(NO2)2] (1)
Quinoxaline (1 mmol, 0.130 g), sodium nitrite (1 mmol, 0.0689 g) and mercury(II) nitrate (0.1713 g, 0.5 mmol) were placed in the main arm of the branched tube. Methanol was carefully added to fill the arms, the tube was sealed and the ligand- and mercury salt containing arm immersed in an oil bath at 60 °C while the other arm was kept at ambient temperature. After 7 days, pale yellow crystals (mp = 130 °C), had deposited in the cooler arm which were isolated, filtered off, washed with acetone and air dried (yield 40%), found; C, 34.85; H, 2.21; N, 15.25%. Calcd for C16H12HgN6O4; C, 34.76; H, 2.19; N, 15.20%. IR (cm−1) selected bands: 609(m), 750(vs), 862(s), 949(s), 1033(s), 1127(s), 1252(vs), 1351(s), 1488(s), 3050(w).
Synthesis of [Hg3(μ-quinoxaline)2(μ-SCN)6]n (2)
Quinoxaline (1 mmol, 0.130 g) and mercury(II) thiocyanate (0.5 mmol, 0.159 g) were placed in the main arm of the branched tube. Ethanol was carefully added to fill the arms, the tube was sealed and the ligand- and mercury salt containing arm immersed in an oil bath at 60 °C while the other arm was kept at ambient temperature. After 5–6 days, brown crystals (d.p. = 130 °C), had deposited in the cooler arm which were isolated, filtered off, washed with acetone and air dried (yield 45%), found; C, 21.95; H, 1.10; N, 11.63%. Calcd for C22H12Hg3N10S6; C, 21.83; H, 1.00; N, 11.57%. IR (cm−1) selected bands: 713(w), 752(m), 863(w), 952(w), 1026(w), 1130(w), 1204(w), 1357(w), 1379(w), 1495(w), 2095(vs), (s), 3060(w).
Synthesis of [Hg(μ-quinoxaline)(μ-CN)2]n (3)
Quinoxaline (1 mmol, 0.130 g) and mercury(II) cyanide (0.5 mmol, 0.138 g) were heated in ethanol for 50 minutes and the filtered solution was maintained undisturbed. Yellow crystals of 3 were obtained after 10 days (d.p. = 250 °C, yield 60%). Found; C, 31.90; H, 1.72; N, 14.83%. Calcd for C10H6HgN4; C, 31.83; H, 1.58; N, 14.64%. IR (cm−1) selected bands: 428(m), 758(s), 865(w), 952(w), 1028(w), 1129(w), 1204(w), 1360(w), 1496(m), 3055(w).
Solid state crystal to crystal transformation
For crystal to crystal conversion, solid of each compound was grinding by hand for 20 minutes with salt of anion of other compounds and then washed with water to remove any unreacted materials.
Removal of sulfur
In a typical run, solution of dibenzothiophene in n-hexane was used as simulated diesel oil, containing 500 mg L−1 sulfur. The desulfurization experiment was performed in a three-necked 100 mL round-bottomed flask including 25 mL of dibenzothiophene solution and desired amount of absorbant. The reaction was done by stirring mixture at a constant speed (1000 rpm) by a magnetic agitator at 30 and 60 °C. Samples for analyses were taken from the reaction suspension at specified reaction times and immediately centrifuged at 6000 rpm for 10 min to remove the particles. After that, the oil phase was analyzed by UV-vis spectroscopy (UV-vis 2100 spectrophotometer (Shimadzu)). To examine reusability of the catalyst, it was several times washed by methanol, then dried at 100 °C for 6 h and then was used in the next run.
The data gathered from the experiments were used to calculate the removal percentage of dibenzothiophene as follows:
DBT removal (%) = ((C0 − Ct)/C0) × 100 |
where
C0 is the initial concentration of sulfur (DBT) in the hexane solution and
Ct is the sulfur concentration of the oil phase after reaction time (
t).
Results and discussion
Characterization of compounds
The IR spectrum of complex 1 has the absorption bands with strong intensity in the frequency range 1252–1175 cm−1 corresponding to vibrations of the nitrite anions. In the IR spectrum of compound 2 very strong bands centered at 2095 and 752 cm−1 characterize the ν(CN) and ν(CS) vibrations of the bridging SCN− anions, respectively.35 Also in the IR spectrum of compound 3 weak absorption band at 1496 cm−1 characterize the ν(CN) vibration of the bridging CN− anions. The lower frequency of CN− (respect to nitrile groups ∼2250 cm−1) is also attributed to coordination to Hg(II) atoms (Fig S1, ESI†).
Single X-ray crystal analysis reveals that [Hg(quinoxaline)2(NO2)2] (1) is a monomeric complex which is crystallized in a triclinic system with space group of P
(Table S1†). As shown in Fig. 1a, Hg(II) has coordination number of six and a distorted octahedral environment in which four oxygen atoms from two different NO2− and two nitrogen atoms from two quinoxaline ligand are coordinated to the mercury center (Table S2†). In this complex, NO2− ions are bonded to the mercury(II) through two oxygen atoms in a bifunctional mode. In complex 1 there are interactions between one hydrogen atom of pyrazine ring and nitrogen atom of neighboring nitrite anion with a distance of 2.680 Å. Also, the nitrogen atom of the pyrazine ring which has no connections with Hg(II) ion, has a weak interaction with a hydrogen atom of benzene ring of the neighboring molecule with a distance of 2.695 Å (Fig. 1b and Fig S2†).
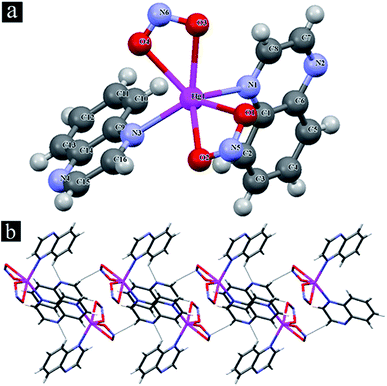 |
| Fig. 1 (a) Structural unit of compound [Hg(quinoxaline)2(NO2)2] (1), (b) packing diagram of 1 in which Hpyrazine⋯NO2 and Npyrazine⋯Hbenzene interaction are shown. Color code: O: red; N: blue; C: gray and Hg: pink. | |
Determination of the structure of the compound [Hg3(μ-quinoxaline)2(μ-SCN)6]n (2) by X-ray crystallography reveals that it is a two-dimensional coordination polymer which is crystallized in triclinic system with space group of P
(Table S1†). In the structure of this compound there are three types of Hg(II) ions with different coordination environments. Hg(1) is five-coordinated with a Hg(1)N3S2 chromophore in a distorted square pyramidal environment. Two other mercury(II) centers are six-coordinated, with Hg(2)N3S3 and Hg(3)N4S2 chromophores and the geometry around Hg(II) can be best regarded as a distorted octahedron (Fig. 2a and Table S3 and S4†). In the polymeric network all the SCN− anions are bridged through their S and N atoms between Hg(II) ions and form one-dimensional chains of Hg(1)-(μ-SCN)2-Hg(2) and Hg(3)-(μ-SCN)2-Hg(3). These one-dimensional chains are further connected to each other through quinoxaline ligand. Finally through this connection as well as the connection between two Hg(2) ions and two S atoms from SCN− anions (planar dimeric Hg(2)2S2 units) a two-dimensional coordination polymer is formed (Fig. 2b).
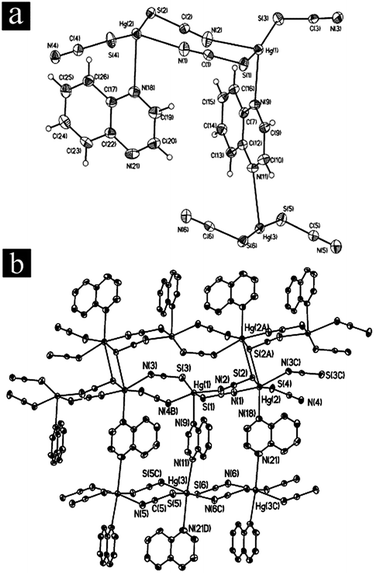 |
| Fig. 2 (a) Independent part of [Hg3(μ-quinoxaline)2(μ-SCN)6]n (2) in representation of atoms by of atoms by thermal ellipsoids (p = 50%), (b) two-dimensional coordination polymer in the 2. | |
Structural determine of [Hg(μ-quinoxaline)(μ-CN)2]n (3) by X-ray crystallography reveals that there are 2D inorganic chains which built of Hg(II) centres doubly bridged by CN− anions. These 2D chains are connected by quinoxaline ligand and form a 3D coordination polymer (Fig. 3a). In the structure of this compound there are two crystallographically independent six-coordinated Hg(II) centres Hg(1)N4C2 (N1, N3, N7, and N8 with Hg–N distances of 2.832 Å, 2.851 Å, 2.716 Å and 2.839 Å, respectively and C17 and C18 with Hg–C distances of 2.061 Å and 2.036 Å, respectively) and Hg(2)N4C2 (N2, N4, N5, and N6 with Hg–N distances of 2.826 Å, 2.793 Å, 2.821 Å and 2.862 Å, respectively and C19 and C20 with Hg–C distances of 2.044 Å and 2.011 Å, respectively) (Fig. 3b and Table S5, ESI†). Interestingly, in this compound CN− anions bind to mercury(II) centres via carbon and nitrogen atoms and make metal carbon bonds.36
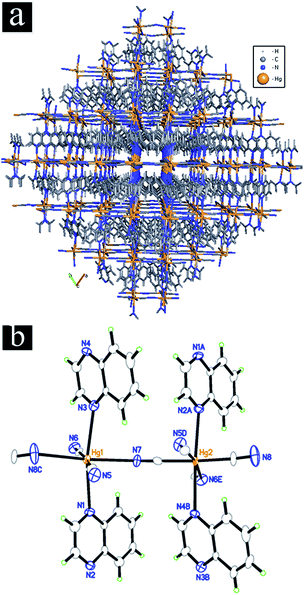 |
| Fig. 3 (a) Representation of 3D framework of [Hg(μ-quinoxaline)(μ-CN)2]n (3), (b) molecular structure of 3 (displacement ellipsoids for non-H atoms are shown at the 50% probability level and H atoms are represented by circles of arbitrary size. Symmetry codes: (A) x + 1, y, z; (B) x, y − 1, z; (C) 1 − x, 1 − y, 2 − z; (D) 1 − x, 1 − y, 1 − z; (E) x − 1, y + 1, z.). | |
The possibility of crystal to crystal transformation between these three compounds was investigated by grinding each compound along with the salt of anion of other compounds. Powder X-ray diffraction pattern (PXRD) analysis shows that only compound 2 and 3 can convert to each other and this crystal–crystal conversion is reversible (Fig. 4). PXRD pattern that obtained from sample after grinding compound 2 with KCN matches with those of compound 3, as calculated from the single-crystal X-ray data (Fig. 4c). PXRD patterns also conform that the sample regains the original structure (compound 2) upon grinding with KSCN (Fig 4d). FE-SEM images show that compounds 2 and 3 have agglomeration of nanoparticles morphologies (Fig. 5).
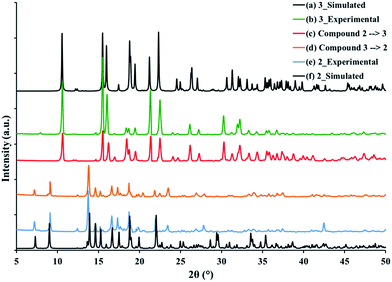 |
| Fig. 4 The XRD patterns of (a) simulated from single crystal X-ray data of compound 3, (b) bulk materials as synthesized of compound 3, (c) bulk materials obtained by solid state anion-replacement of compound 2, (d) the reversed species obtained by solid state anion-replacement of compound 3, (e) bulk materials as synthesized of compound 2 and simulated from single crystal X-ray data of compound 2. | |
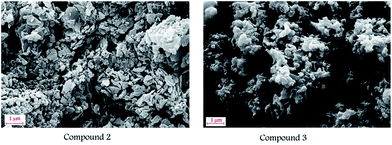 |
| Fig. 5 FE-SEM images of compounds 2 and 3. | |
In order to examine the thermal stabilities of compounds 1–3, thermal gravimetric analysis (TGA) and differential thermal analysis (DTA) were carried out between 30 and 600 °C. The TGA–DTA curves of compounds 1–3 indicate that decomposition of these compounds take place after 130 °C, 100 °C and 145 °C, respectively with two endothermic effects at 130 and 173 °C, three exothermic effects at 236, 317 and 480 °C for 1 (Fig. S3, ESI†), two endothermic effects at 113 and 140 °C and one exothermic effect at 520 °C for 2 (Fig. S4, ESI†), two endothermic effects at 180 and 240 °C and three exothermic effects at 320, 388 and 493 °C for 3 (Fig. S5, ESI†).
Evaluation of sulfur removal
Optimizing the removal reaction conditions. Type, amount of absorbent and temperature are crucial factors in the adsorption reactions. Table 1 reveals the role of these parameters on the removal efficiency. The results show that the percentage of the adsorption for compound 2 is more than that compound 3. This phenomenon is probably related to different coordination environments in two compounds. Unsaturated coordination number around Hg(1) in compound 2 may be responsible for more adsorption efficiency in comparison with the other compound. In addition, the removal efficiency is enhanced by increasing the amount of the catalyst. However, with further increasing in catalyst weight (>100 mg), the sulfur removal was not increased significantly. Furthermore, upon adding more dosage of adsorbent to reaction, the adsorption capacity is decreased.
Table 1 Effect of amount of adsorbents and adsorption capacity on DBT removal of model oil at various temperatures for 75 min
Compound |
Adsorbent dosage (mg) |
DBT remained (ppm) |
DBT removal (%) |
Adsorption capacity (mg g−1) |
30 °C |
60 °C |
30 °C |
60 °C |
30 °C |
60 °C |
2 |
0 |
500 |
500 |
0 |
0 |
— |
— |
2 |
50 |
375 |
285 |
25 |
43 |
63 |
108 |
2 |
100 |
240 |
130 |
52 |
74 |
65 |
93 |
2 |
150 |
240 |
120 |
52 |
76 |
43 |
63 |
2 |
200 |
235 |
112.5 |
53 |
77.5 |
33 |
48 |
3 |
0 |
500 |
500 |
0 |
0 |
— |
— |
3 |
50 |
427.5 |
410 |
14.5 |
18 |
36 |
45 |
3 |
100 |
325 |
290 |
35 |
42 |
44 |
53 |
3 |
150 |
325 |
285 |
35 |
43 |
29 |
36 |
3 |
200 |
325 |
282.5 |
35 |
43.5 |
22 |
27 |
The effects of the reaction time and temperature on the sulfur removal over adsorbent of 2 are indicated in Fig. 6. As the results show, the sulfur removal is increased along with the reaction time after 75 minutes.
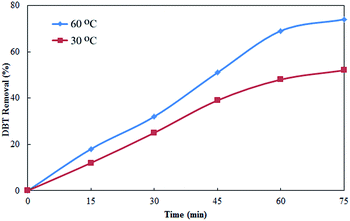 |
| Fig. 6 Removal of DBT versus time at different temperatures over 100 mg adsorbent of 2. | |
The reusability of the catalyst was studied as an important factor in the DBT removal. After each catalytic run, the catalyst was recovered by simple washing with methanol several times, and drying at 100 °C for 6 hours. During the second cycle of the reaction in presence of the recovered 2 adsorbent, a slight reduction in the DBT removal is observed (Fig. 7a); however, the catalytic activity is nearly retained during the later runs. Also, the adsorbent of 2 is very stable during four repeated experiments. The XRD pattern of the adsorbent before and after repeating the reaction four times is shown in Fig. 7b which clearly indicates that the structure remained the same and no change was observed.
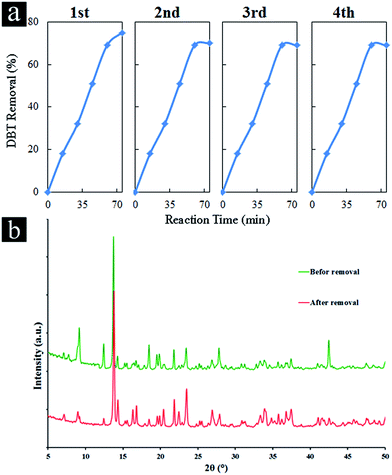 |
| Fig. 7 (a) Cycling run in the removal of DBT in presence of adsorbent 2 for 75 min and (b) XRD pattern of adsorbent 2 before and after 4 times removal test. | |
Kinetics of sulfur removal. In order to know the kinetics of DBT removal of model oil over adsorbent of 2, different kinds of kinetics orders are attempted expressing the reaction kinetics as shown in Table S6.† Each correlation coefficient was calculated from the kinetics equation, where R0, R1 and R2 represent the correlation coefficients of zero, first and second order rate equations, respectively. Comparison between these correlation coefficients shows that R1 has the best correlation for the different orders in Table S6.† Therefore, it is suggested that the DBT removal in n-hexane solution suspended on adsorbent of 2 belongs to first order reaction kinetics (Fig. S6†). The apparent rate constants in the removal of DBT were 0.0104 and 0.0188 min−1 at 30 and 60 °C, respectively. The half-times (t1/2), at which [A] = [A]0/2, were also calculated by eqn (1), were 66.6 min and 36.8 min at 30 and 60 °C, respectively.The activation energy (Ea = 16.57 kJ mol−1) for DBT removal process was also obtained by using Arrhenius equation at two different temperatures (eqn (2) and (3))
|
k = A e−Ea/RT
| (2) |
|
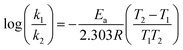 | (3) |
where
A is the frequency factor for the reaction,
R is the universal gas constant,
T is the temperature (in Kelvin), and
k is the rate constant while subscripts 1 and 2 show the reactions done at temperatures 30 and 60 °C, respectively.
Conclusions
Three new Hg(II) compounds, [Hg(quinoxaline)2(NO2)2] (1), [Hg3(μ-quinoxaline)2(μ-SCN)6]n (2) and [Hg(μ-quinoxaline) (μ-CN)2]n (3) have been synthesized using a thermal gradient approach and studied by IR spectroscopy, thermal gravimetric analysis (TGA) and characterized by single-crystal X-ray crystallography. Complex [Hg(quinoxaline)2(NO2)2] (1) is monomeric and Hg(II) has coordination number of six. But compounds [Hg3(μ-quinoxaline)2(μ-SCN)6]n (2) and [Hg(μ-quinoxaline)(μ-CN)2]n (3) are two- and three-dimensional coordination polymers and by a solid state anion replacement reversible crystal to crystal transformation was observed. Coordination polymer 2 was used as a highly efficient adsorbent in DBT removal of model oil. Removal of DBT solution in n-hexane (500 mg L−1) was carried out under optimized conditions, a pseudo-first-order model was fitted with the experimental data with a good correlation coefficient of 0.9863. Our findings may provide some insight into the preparation of adsorbents with high performance in practical applications for sulfur removal.
Acknowledgements
This work was supported by National High-tech R&D Program of China (Grant No. 201037), grant NRF-2015-002423 of the National Research Foundation of Korea and Tarbiat Modares University.
Notes and references
- E. Coronado, J. R. Galan-Mascaros, C. J. Gomez-Garcia and V. Laukhin, Nature, 2000, 408, 447–449 CrossRef CAS PubMed.
- H. Hou, Y. Song, H. Xu, Y. Wei, Y. Fan, Y. Zhu, L. Li and C. Du, Macromolecules, 2003, 36, 999–1008 CrossRef CAS.
- D. Lupo, Adv. Mater., 1995, 7, 248–249 CrossRef PubMed.
- A. Morsali and M. Y. Masoomi, Coord. Chem. Rev., 2009, 253, 1882–1905 CrossRef CAS PubMed.
- A. J. Blake, N. R. Champness, P. Hubberstey, W.-S. Li, M. A. Withersby and M. Schröder, Coord. Chem. Rev., 1999, 183, 117–138 CrossRef CAS.
- M. Y. Masoomi and A. Morsali, Coord. Chem. Rev., 2012, 256, 2921–2943 CrossRef CAS PubMed.
- M. G. Amiri, G. Mahmoudi, A. Morsali, A. D. Hunter and M. Zeller, CrystEngComm, 2007, 9, 686–697 RSC.
- E. J. Corey, Pure Appl. Chem., 1967, 14, 19–38 CrossRef CAS.
- G. R. Desiraju, Angew. Chem., Int. Ed., 1995, 34, 2311–2327 CrossRef CAS PubMed.
- M. Y. Masoomi, A. Morsali and P. C. Junk, CrystEngComm, 2015, 17, 686–692 RSC.
- A. D. Burrows, CrystEngComm, 2011, 13, 3623–3642 RSC.
- B. Moulton and M. J. Zaworotko, Chem. Rev., 2001, 101, 1629–1658 CrossRef CAS PubMed.
- T. Kuroda-Sowa, T. Horino, M. Yamamoto, Y. Ohno, M. Maekawa and M. Munakata, Inorg. Chem., 1997, 36, 6382–6389 CrossRef CAS.
- D. R. Turner and S. R. Batten, CrystEngComm, 2008, 10, 170–172 RSC.
- M. Y. Masoomi and A. Morsali, RSC Adv., 2013, 3, 19191–19218 RSC.
- T. Friscic and L. Fabian, CrystEngComm, 2009, 11, 743–745 RSC.
- V. A. Russell, C. C. Evans, W. Li and M. D. Ward, Science, 1997, 276, 575–579 CrossRef CAS.
- D. Venkataraman, S. Lee, J. Zhang and J. S. Moore, Nature, 1994, 371, 591–593 CrossRef CAS PubMed.
- V. A. Russell, M. C. Etter and M. D. Ward, J. Am. Chem. Soc., 1994, 116, 1941–1952 CrossRef CAS.
- M. Y. Masoomi, S. Beheshti and A. Morsali, Cryst. Growth Des., 2015, 15, 2533–2538 CAS.
- M. Y. Masoomi, S. Beheshti and A. Morsali, J. Mater. Chem. A, 2014, 2, 16863–16866 CAS.
- Q.-G. Zhai, C.-Z. Lu, X.-Y. Wu and S. R. Batten, Cryst. Growth Des., 2007, 7, 2332–2342 CAS.
- M. Y. Masoomi, A. Morsali and P. C. Junk, RSC Adv., 2014, 4, 47894–47898 RSC.
- M. Y. Masoomi, K. C. Stylianou, A. Morsali, P. Retailleau and D. Maspoch, Cryst. Growth Des., 2014, 14, 2092–2096 CAS.
- S. Vaucher, J. Fielden, M. Li, E. Dujardin and S. Mann, Nano Lett., 2001, 2, 225–229 CrossRef.
- M. Y. Masoomi, G. Mahmoudi and A. Morsali, J. Coord. Chem., 2010, 63, 1186–1193 CrossRef CAS PubMed.
- A. Mahjoub and A. Morsali, J. Coord. Chem., 2003, 56, 779–785 CrossRef CAS PubMed.
- A. Morsali and R. Kempe, Helv. Chim. Acta, 2005, 88, 2267–2271 CrossRef CAS PubMed.
- V. Toteva, A. Georgiev and L. Topalova, Fuel Process. Technol., 2012, 101, 101–105 CrossRef CAS PubMed.
- H. Shang, H. Zhang, W. Du and Z. Liu, J. Ind. Eng. Chem., 2013, 19, 1426–1432 CrossRef CAS PubMed.
- V. Lam, G. Li, C. Song, J. Chen, C. Fairbridge, R. Hui and J. Zhang, Fuel Process. Technol., 2012, 98, 30–38 CrossRef CAS PubMed.
- A. Ishihara, D. Wang, F. Dumeignil, H. Amano, E. W. Qian and T. Kabe, Appl. Catal., A, 2005, 279, 279–287 CrossRef CAS PubMed.
- G. M. Sheldrick, SHELX97 program for Crystal Structure Solution and Refinement; University of Göttingen: Göttingen, Germany, 1997 Search PubMed.
- G. Sheldrick, Acta Crystallogr., Sect. A, 2008, 64, 112–122 CrossRef CAS PubMed.
- K. Nakamoto, Infrared and Raman Spectra of Inorganic and Coordination Compounds, John Wiley & Sons, Inc., New York, Sixth ed. 2008 Search PubMed.
- P. E. M. Siegbahn, J. Phys. Chem., 1995, 99, 12723–12729 CrossRef CAS.
Footnotes |
† Electronic supplementary information (ESI) available: [CCDC 1006977–1006979]. See DOI: 10.1039/c5ra12686j |
‡ These authors contributed equally to this work. |
|
This journal is © The Royal Society of Chemistry 2015 |