DOI:
10.1039/C5RA11945F
(Paper)
RSC Adv., 2015,
5, 70287-70296
Preparation of liquefied wood-based activated carbon fibers by different activation methods for methylene blue adsorption
Received
21st June 2015
, Accepted 12th August 2015
First published on 13th August 2015
Abstract
In order to study the adsorption for methylene blue (MB), five types of activated carbon fibers (ACFs) were prepared from liquefied wood by different activation methods. The ACFs activated by pure physical and chemical activation were dominantly microporous and mesoporous, respectively, which showed relatively low adsorption capacity for MB. The chemical/physical coupling activation was an effective method to improve the mesoporosity resulting in the reinforcement of MB adsorption ability. ACFs by chemical–physical reactivation even had abundant macropores in addition to well-developed micro- and mesoporosity, while physical–chemical reactivation did not seem to be an efficient one. Their potentially different mechanisms of pore formation and kinetics adsorption of MB were investigated.
1. Introduction
Dyes have been widely applied in many industries, such as textile, paper production, food technology, etc.1 The attendant industrial wastewater usually contains a great deal of organic compounds and toxic substances. For example, methylene blue (MB), commonly used for dying cotton, wood and silk, is harmful to people's eyes and inhalating it can cause eye burns and give rise to short periods of difficult breathing.2–4 Besides, ingestion via the mouth would result in a burning sensation and may cause other diseases such as nausea, mental confusion and painful micturition.5 Thus, the treatment of wastewater consisting mostly of such dye is of great importance.
Activated carbon fibers (ACFs), due to their capability for efficiently adsorbing a broad range of adsorbates and their simplicity of design, have been found to be superior to other techniques for wastewater treatment.1 However, most of them are prepared from fossil resources such as coals, which are not only expensive but also non-renewable. Currently, renewable industrial and agricultural by-products such as liquefied wood used as precursors have attracted more and more attentions on account of their advantages in environmental protection and regeneration.6,7
In our laboratory, ACFs of specific surface area more than 2000 m2 g−1 were prepared from liquefied wood by steam activation,8 but these microporous ACFs were not suitable for adsorption of large molecule substance like MB. In our previous work, ACFs with mesoporosity by KOH activation were prepared and reaction mechanisms during the activation were investigated.9 Hence, an attempt was made here to combine the two methods either simultaneously or separately to promote the generation of mesoporosity and even macroporosity for large molecule substance's adsorption. The mechanisms on pore formation during activation process as well as the adsorption isotherm and kinetics of the obtained ACFs were investigated.
2. Experimental
2.1 Preparation and characterization of ACFs
Liquefied wood-based fibers were prepared through a series of process including liquefaction, melt-spinning and curing according to our previous study,10 based on which five activation methods were employed to prepare ACFs (Fig. 1). All the ACFs were washed with hot deionized water and 0.1 M hydrochloric acid until the pH of the washing solution reached 6–7. The porosity changes were observed by a scanning electron microscope (SEM, Hitachi S-3400N, Japan) coupled with an energy dispersed X-ray analyzer (Horiba 7021-H, Japan) and analyzed by N2 adsorption at 77 K (Quantachrome, Autosorb-iQ, USA). The chemical groups of the samples were studied by a Fourier transform infrared spectrometer (FTIR, BRUKER Tensor 27, German) in the range of 4000–400 cm−1, using pellets with samples dispersed in KBr. X-ray photoelectron spectroscopy (XPS) measurements were carried out on a spectrophotometer (Thermo Scientific ESCALAB 250Xi) to determine the number of functional groups present on the surface of the ACFs with a monochromated Al Kα X-ray source (hν = 1486.6 eV) and a nonlinear least squares curve-fitting program (XPSPEAK software, Version 4.1) was employed for XPS spectral deconvolution. The determination of the pHpzc of the ACFs was carried out by using the acid base titration reported by Bouaziz et al. (2015).11 10 mL NaCl solution (0.01 M) with pH value between 2 and 12 (adjusted using either HCl or NaOH, 0.1 M) was added to 30 mg of ACFs and the final pH measured after 24 h under agitation at room temperature. The pH is the point where the curve pHfinal vs. pHinital crosses the line pHinitial = pHfinal.
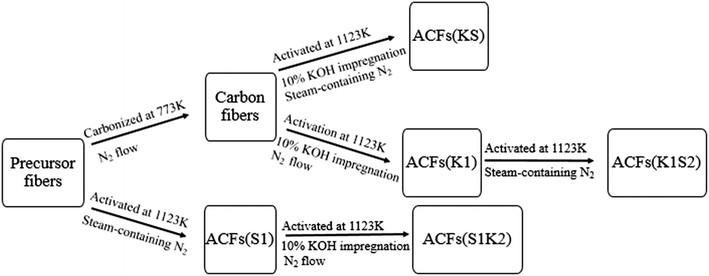 |
| Fig. 1 Scheme of process routes for preparing ACFs by different activation methods. | |
2.2 Adsorption experiments
The adsorption performance of the as-prepared ACFs was tested for the adsorption of methylene blue (MB, CAS 7220-79-3, Tianjin Jinke Fine Chemicals CO. Ltd, China). The tests were performed in a set of Erlenmeyer flasks (100 mL) where 100 mL of MB solutions with initial concentrations of 100–500 mg L−1 were placed in these flasks. About 3 mg of ACFs was added to each flask and kept in an isothermal shaker at 25 °C for 24 h to reach equilibrium. Aqueous samples were taken from the solutions and the concentrations were analyzed. Prior to analysis, all samples were filtered in order to minimize interference of carbon fines with the analysis. The concentrations of MB in the supernatant solutions before and after adsorption were determined by using a UV-vis spectroscopy (Biowave II, WPA, England) at a wavelength of 665 nm. The adsorbed MB mass per unit mass, qt (mg g−1) at adsorption time t (h) was calculated by: |
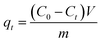 | (1) |
where C0 (mg L−1) and Ct (mg L−1) are the liquid-phase concentrations of dye at initial time t (h) the initial MB concentration. V is the volume of solution (L) and m is the mass of the adsorbent.
3. Results and discussion
3.1 Textural characterization of prepared ACFs
3.1.1 Yield of ACFs. Basically, ACFs of high specific surface area are associated with low yield. An efficient ACF production process combines a well-developed porosity with an acceptable fabrication yield. In the present study, the ACF production yields were monitored for the five activation methods.As shown in Table 1, there is a difference in the yield as a function of the activating agent for the same activating temperature. The yield of ACFs by single KOH activation (K1) was as high as 76%, while the yield of ACFs by single steam activation (S1) was 41%, suggesting the latter had a better porosity development. For the case of chemical/physical activation (KS), physical–chemical (S1K2) and chemical–physical reactivation (K1S2), the ACF production yields were quite similar (26%, 27% and 30%, respectively), which had a significant reduction compared with those of ACFs by single activation. This can be attributed to the additional activation process that resulted in more burn-off of carbon.
Table 1 Pore structural parameters of ACFs from N2 adsorption isotherms and their adsorption capacity for MB
Sample |
Total surface areaa (m2 g−1) |
Total pore volumeb (cm3 g−1) |
Micropore volumec (cm3 g−1) |
Mesopore volumed (cm3 g−1) |
Macropore volumee (cm3 g−1) |
Yieldf (%) |
MB adsorption (mg g−1) |
Calculate from the Brunauer–Emmett–Teller (BET) method. Calculate from the N2 adsorption at a relative pressure of 0.995. Calculate from the V–t method. Calculate from the BJH method. Calculate from the difference between the total pore volume and the sum of micro- and mesopore volume. Base on the weight ratio of ACFs to precursor fibers. |
S1 |
1240 |
0.64 |
0.38 |
0.214 |
0.046 |
41 |
242 |
S1K2 |
1081 |
0.558 |
0.364 |
0.177 |
0.017 |
27 |
167 |
K1 |
545 |
0.504 |
0.013 |
0.41 |
0.081 |
76 |
684 |
K1S2 |
1658 |
1.214 |
0.49 |
0.486 |
0.238 |
30 |
877 |
KS |
1651 |
1.097 |
0.418 |
0.521 |
0.158 |
26 |
920 |
3.1.2 Nitrogen adsorption behavior and porosity development. Fig. 2 shows the adsorption/desorption isotherms of N2 for the ACFs prepared by different activation methods. The shape of the isotherm curves was analyzed sing the IUPAC classification.12 The isotherms for S1 and S1K2 belong to type I based on the IUPAC classification that rose sharply at low relative pressures and approached a plateau which was parallel to the relative pressure axis, indicating these ACFs were mainly microporous. The N2 uptake of S1 is higher than that of S1K2, meaning that reactivation with KOH reduced the pore volume. However, for the K1, the absorbed volume maintains a linear upward trend from very low pressure even less than 0.1, indicating the isotherms belonged to non-microporous type. The isotherms of KS and K1S2 are characterized by both a broader knee and a clearer hysteresis loop, corresponding to a combination of type I and IV isotherm,13 suggesting the co-existence of micropores and mesopores. Meanwhile, a small tail can be observed at the end of isotherms, implying the appearance of macropores.
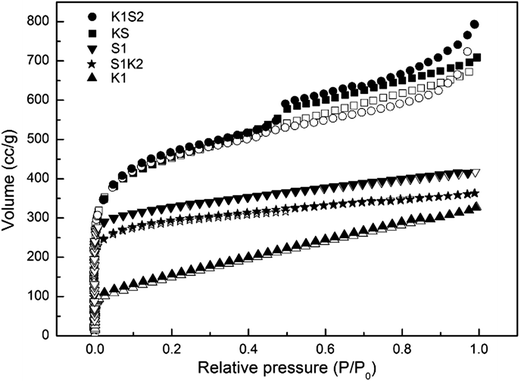 |
| Fig. 2 N2 adsorption isotherms for the ACFs. Open symbols: adsorption branch. Solid symbols: desorption branch. | |
The pore size distributions (PSDs) of the ACFs obtained by applying Density Functional Theory (DFT) method to N2 adsorption data are presented in Fig. 3 and their pore structure parameters are summarized in Table 1. The PSDs of S1 and S1K2 mainly concentrate in micropore region where the contour of their PSDs are very similar, but micropore accumulation of S1K2 is lower than that of S1 (Fig. 3a). And mesopore accumulation of S1K2 has a slight decrease rather than an anticipated increase, except for the peak at ca. 6.8 nm. This states that reactivation with KOH did not produce mesoporosity but reduced the microporosity on the contrary. The possible reaction between KOH and carbon could be described as follows:
|
6KOH + 2C → 2K + 3H2 + 2K2CO3
| (2) |
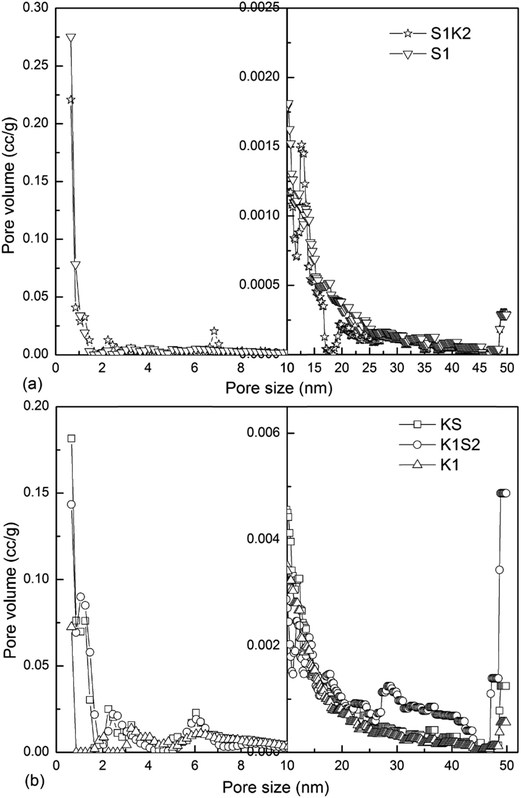 |
| Fig. 3 DFT pore size distributions for the ACFs. | |
It can be inferred that, after impregnation, KOH mainly concentrated in the micropores which were created by single steam activation. The concentration of evolved H2 would reach very high value due to the confined space (Fig. 4a), which could inhibit the forward reaction (1).14 Thus, KOH cannot efficiently serve as an activator. Furthermore, the remaining KOH and reaction products such as K2CO3 blocked the pores and decreased microporosity. This phenomenon was very similar to the result of Miyamoto et al. (2005),15 who produced ACFs by K2CO3 reactivation from pith-based ACFs. Their result showed reactivation with K2CO3 did not produce mesoporosity and reduced the microporosity.
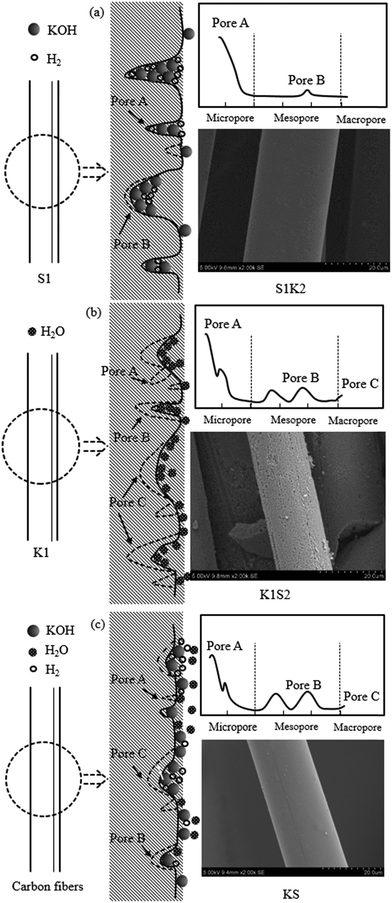 |
| Fig. 4 Possible mechanisms of pore formation during the reactivation and coupling activation. | |
As for K1, there are few micro- and mesopores mainly accumulate in a narrow range around 2–4 nm and 5–10 nm, indicating that KOH helped to produce mesopores when the concentration of KOH solution was low (10%). When K1 was reactivated to K1S2, microporosity had a great development. In addition, mesopores accumulated to a deeper extent and macropore volume had a sharp increase simultaneously. This can be interpreted that carbon atom at the graphite layers was further eroded by steam during the reactivation. This process resulted in the formation of dominant micropores and contributed to the enlargement of some micro- and mesopores into meso- and macropores, respectively (Fig. 4b). Relatively speaking, pure KOH activation is width activation for mesopore formation, while steam reactivation can be recognized as depth activation which is responsible for micropore generation and of pore expansion.
Compared with K1 and K1S2, KS has similar PSDs but presents more mesopore accumulation around 2–15 nm, and the macropore volume falls in the range between K1 and K1S2. This phenomenon can be ascribed to several reasons as follows. During the coupling activation, when the temperature reached the activation temperature (1123 K), a little KOH attached on the fibers had already reacted with carbon, thereby some mesopores formed. The introducing of steam improved the micropore reaction and this steam flow could decrease the intra-pore gas phase concentrations, thereby promoting the reaction between KOH and carbon to generate mesopores. Furthermore, less reaction points were provided for steam activation compared with reactivation process of K1S2 (Fig. 4c) owing to parts of the fiber surface taken up by KOH as well as the effect of the evolved H2 flow. Therefore, less mesopores could be enlarged to macropores. Accordingly, the corresponding micropore accumulation of KS should be lower than that of K1S2. This is just verified by the data of micropores in Table 1.
3.1.3 Chemical structure characteristics. The 4000–400 cm−1 infrared spectral region of the liquefied wood-based ACFs prepared by different activation methods is shown in Fig. 5. The spectra of S1 and K1 exhibit similar bands and shoulders which are assigned to various vibration modes in atomic groups and structures except two peaks in 1300–1000 cm−1 region, where the band is quite difficult to be identified because it may be ascribed to C–O in acids, alcohols, phenols, ethers or ester groups.16 The peaks at 1089 and 1049 cm−1 in the spectrum of K1 indicated various types of C–O groups existed in KOH-activated ACFs. Nevertheless, the two peaks become weak in the spectra of K1S2, S1K2 and KS, suggesting the decrease of oxygen containing functional groups caused by further or coupling activation. Moreover, two bands at 880 and 798 cm−1 appear in the spectrum of each sample, which are attributed to the out-plane bending vibration of C–H in the aromatic rings.17 This demonstrated low substituted aromatic rings still existed in the structure of these ACFs.
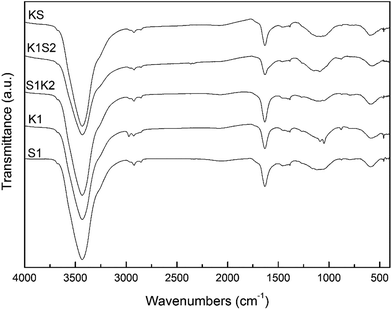 |
| Fig. 5 FTIR spectra of liquefied wood-based ACFs prepared by different activation methods. | |
The elemental compositions of the ACFs by EDX are listed in Table 2. Basically, the oxygen content of ACFs by reactivation or coupling activation had a slight decrease compared to those by single activation. However, it is noteworthy that the oxygen content of S1K2 is even higher than any other ACFs, which is in agreement with the above porosity analysis. It can be attributed to the high concentration of KOH in the micropores by using this reactivation method. The XPS analysis was further used to evaluate the changes in the chemical bonding states and concentrations of the surface functional groups. For all ACF samples, the C1s signals exhibited an asymmetric tailing (Fig. 6), which was partially due to the intrinsic asymmetry of the graphite peak or to the contribution of oxygen surface complexes. Thus, the C1s spectrum has been deconvoluted into six components with binding energies corresponding to: (I) graphite type (284.7 eV); (II) amorphous carbon present in phenol, alcohol, ether or C
N groups (285.6–285.8 eV); (III) carbonyl or quinine groups (286.6–286.9 eV); (IV) carboxyl, lactone, or ester groups (287.7–289.9 eV); (V) carbonate groups (289.3–290.9 eV) and (VI) a peak corresponding to π
π* transitions in aromatic rings (291.3–291.7 eV).18 These functional groups might exercise a profound impact on the surface properties of ACFs and thus affected their adsorption characteristics.
Table 2 Elemental compositions (wt%) of ACFs as determined by EDX and pHpzc value of ACFs
Sample |
Elemental analysis |
pHpzc |
C (wt%) |
O (wt%) |
N (wt%) |
S1 |
90.7 |
8.9 |
0.4 |
6.2 |
K1 |
87.1 |
12.3 |
0.6 |
6.4 |
S1K2 |
82.6 |
16.8 |
0.6 |
6.5 |
K1S2 |
90.3 |
9.2 |
0.5 |
6.2 |
KS |
89.7 |
9.8 |
0.5 |
6.1 |
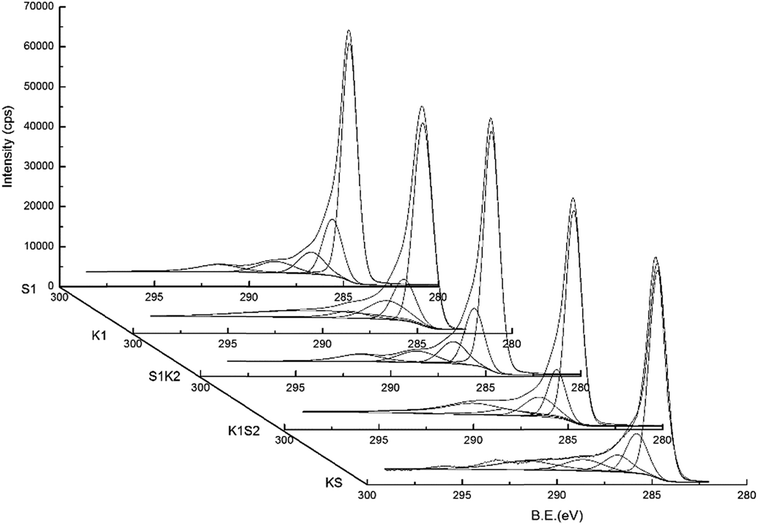 |
| Fig. 6 XPS spectra of C1s region of the liquefied wood-based ACFs prepared by different activation methods. | |
3.2 Equilibrium and kinetics adsorption of ACFs
From Table 2, the pHpzc values of all the ACFs were found to be 6–7, which indicated that the surface of the ACFs were nearly neutral. The original pH of the MB solution was found to be 6.6, which is above the pHpzc of the ACFs. According to previous literature, at pH > pHpzc, the ACFs' surfaces are negatively charged favouring the adsorption of cationic dyes such as MB.11 Therefore, this pH of the MB solution was very suitable for higher removal from aqueous solution.
3.2.1 Effect of contact time and initial MB concentration on adsorption equilibrium. Fig. 7 shows the adsorption capacity of the ACFs versus the adsorption time at various initial MB concentrations at 25 °C, where the amount of adsorbed MB increases with time until reaching a plateau phase for all concentrations. Nevertheless, the contact time needed for MB solution to reach equilibrium varies according to the initial MB concentration. As for the lower concentrations (i.e. 100 and 200 mg L−1), about 5 h were necessary to reach equilibrium while 12 h were required for the higher concentrations (300–500 mg L−1). According to previous literature,1 the mass transport associated with the adsorption of solute form solution by porous adsorbent was divided into three steps including the adsorbate migrating through the solution, solute movement from particle surface into interior site and adsorbate being adsorbed into the active sites at the interior of the adsorbent particle, which takes relatively long contact time. A similar phenomenon was observed for the adsorption of dyes onto ACs from Mediterranean Posidonia oceanica (L.) fibers19 and the equilibrium time was 8–10 h for higher MB concentrations.
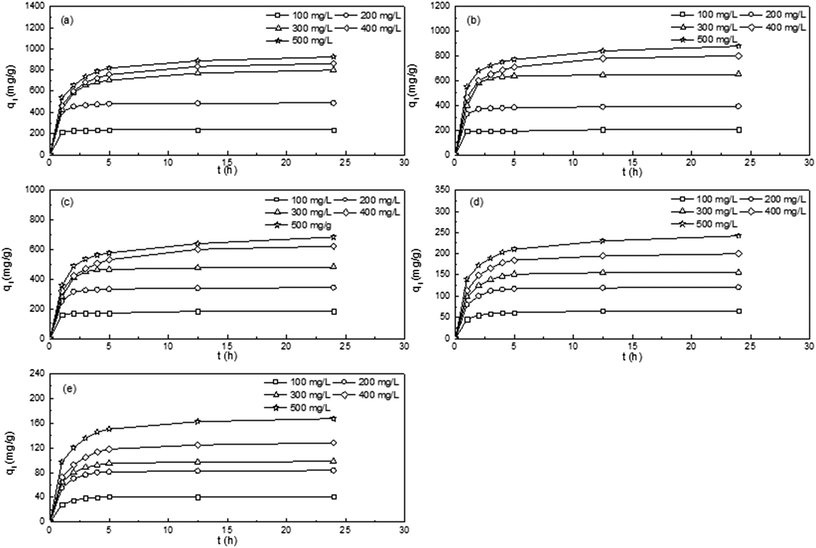 |
| Fig. 7 The variation of adsorption capacity of ACFs with adsorption time at various initial MB concentrations at 25 °C: (a) KS; (b) K1S2; (c) K1; (d) S1; (e) S1K2. | |
The amount of dye adsorbed at the equilibrium time reflects the maximum adsorption capacity of the adsorbent under those operating conditions. The adsorption capacity of KS at equilibrium (qe) (obtained by qt at 24 h) increased from 234 to 920 mg g−1 with increasing the initial concentrations from 100 to 500 mg L−1. This can be attributed to the increase in the concentration gradient or higher concentration of MB in solution which leads to enhanced adsorption capacity of ACFs, further being conformed by the Le Chatelier's principle which suggests that equilibrium position shifts towards adsorption with the increment in adsorbate concentration in solution. As seen from Table 1, under the same initial concentrations (500 mg L−1) and operating conditions, KS shows the highest capacity for MB followed by K1S2. K1 has a MB adsorption amount of 684 mg g−1, while S1 only exhibits 242 mg g−1, demonstrating that physical–chemical reactivation or chemical/physical coupling activation really improved the adsorption ability for MB. S1K2 with the lowest Vmeso also presents the weakest adsorption properties, indicating MB adsorption was strongly related to Vmeso.
3.2.3 Adsorption kinetics. Adsorption kinetics is capable to provide information about the mechanism of adsorption and potential rate controlling steps such as mass transfer and chemical reaction, which is important for designing a fast and effective model adsorption system in practice,22 so three simplified kinetic models including pseudo-first-order,23 pseudo-second-order24 and intraparticle diffusion model25 were applied to investigate the mechanism of the adsorption process. Based on the adsorption data of KS, these three kinetics models were plotted in Fig. 8 and all the calculated parameters are summarized in Table 4. It was found that all of the correlation coefficients are greater and the sum of error squares (SSE) are smaller for pseudo-second-order kinetic model compared to those for the other two kinetics. It also showed a good agreement between the experimental and the calculated qe values in case of pseudo-second-order kinetics, indicating the kinetic adsorption behavior of MB onto liquefied wood-based ACFs can be favorably described by pseudo-second-order model.
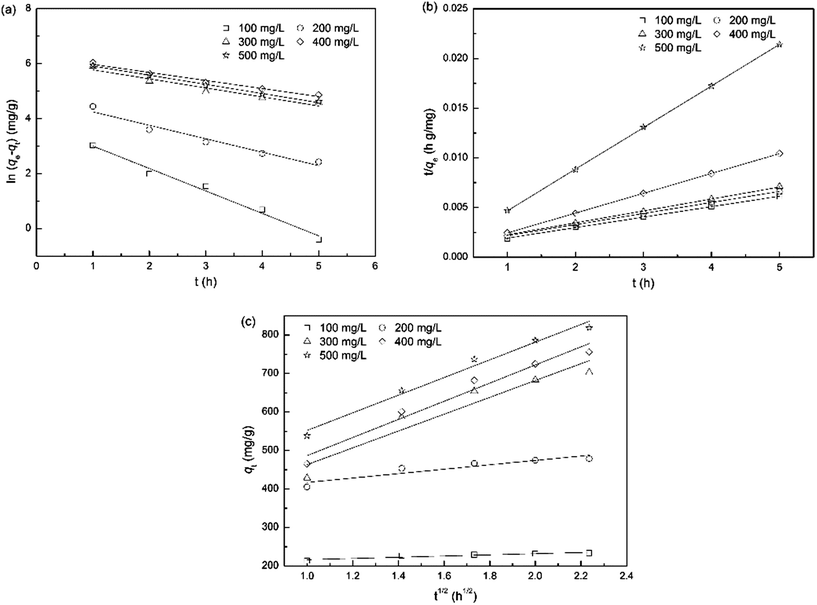 |
| Fig. 8 Kinetic models for adsorption of MB adsorption onto KS at 25 °C. (a) Pseudo-first-order kinetics; (b) pseudo-second-order kinetics; (c) intraparticle diffusion model. | |
Table 4 Comparison of the pseudo-first-order, pseudo-second-order and intraparticle diffusion model for different initial MB concentrations
Model |
|
Constants |
C0 (mg L−1) |
|
100 |
200 |
300 |
400 |
500 |
qe,exp (mg g−1) |
|
234.03 |
489.85 |
800.26 |
861.44 |
922.71 |
Pseudo-first-order: ln(qe − qt) = ln qe − k1t |
qe,cal (mg g−1) |
45.6 |
114.18 |
448.18 |
527.18 |
516.98 |
k1 (h−1) |
0.82 |
0.49 |
0.33 |
0.29 |
0.33 |
R2 |
0.984 |
0.960 |
0.947 |
0.983 |
0.996 |
SSE |
188.43 |
374.66 |
349.76 |
357.71 |
402.45 |
Pseudo-second-order: t/qt = 1/(k2qe2) + t/qe |
qe,cal (mg g−1) |
238.66 |
500.75 |
832.64 |
899.28 |
948.77 |
k2 (g mg−1 h−1) |
0.0014 |
0.0012 |
0.0015 |
0.009 |
0.038 |
R2 |
0.999 |
1.000 |
0.999 |
1.000 |
1.000 |
SSE |
0.59 |
2.32 |
9.11 |
4.23 |
11.50 |
Intraparticle diffusion: qt = kpt1/2 |
qe,cal (mg g−1) |
235.57 |
487.80 |
739.92 |
772.25 |
835.58 |
kp (mg g−1 h−1/2) |
15.44 |
57.31 |
218.55 |
235.90 |
229.28 |
R2 |
0.877 |
0.877 |
0.915 |
0.968 |
0.980 |
SSE |
2.51 |
9.36 |
28.93 |
18.76 |
13.22 |
4. Conclusions
The porosity of ACFs could be designed and well controlled for MB adsorption by different combination scheme of physical and chemical activation, which was probably attributed to different mechanisms on pore formation. Because KOH activation provided more reaction points for the subsequent steam activation, ACFs rich in macropores with developed micro- and mesoporosity can be prepared by steam-KOH reactivation and showed excellent adsorption ability, which was not true if the two-step activation was reversed. Chemical/physical coupling activation is also an effective method to improve MB adsorption capacity as a result of enhancing mesoporosity as well as ensuring high specific surface area. Equilibrium data were fitted to Langmuir and Freundlich isotherms and the former was seemed to be better. The kinetics of the adsorption process was found to follow the pseudo-second-order kinetic model.
Acknowledgements
The research was financially supported by Specialized Research Fund for the Doctoral Program of Higher Education (No. 20130014130001).
References
- I. A. W. Tan, B. H. Hameed and A. L. Ahmad, Equilibrium and kinetic studies on basic dye adsorption by oil palm fibre activated carbon, Chem. Eng. J., 2007, 127, 111–119 CrossRef CAS PubMed.
- D. Ghosh and K. G. Bhattacharyya, Adsorption of methylene blue on kaolinite, Appl. Clay Sci., 2002, 20, 295–300 CrossRef CAS.
- B. H. Hameed, A. T. M. Din and A. L. Ahmad, Adsorption of methylene blue onto bamboo-based activated carbon: kinetics and equilibrium studies, J. Hazard. Mater., 2002, 141, 819–825 CrossRef PubMed.
- A. F. Hassan, A. M. Abdel-Mohsen and M. M. G. Fouda, Comparative study of calcium alginate, activated carbon, and their composite beads on methylene blue adsorption, Carbohydr. Polym., 2014, 102, 192–198 Search PubMed.
- J. Avom, J. K. Mbadcam, C. Noubactep and P. Germain, Adsorption of methylene blue from an aqueous solution onto activated carbon from palm-tree cobs, Carbon, 1997, 35, 365–369 CrossRef CAS.
- M. E. Fernandez, G. V. N. Pablo, P. R. Bonelli and A. L. Cukierman, Activated carbon developed from orange peels: batch and dynamic competitive adsorption of basic dyes, Ind. Crops Prod., 2014, 43, 617–622 Search PubMed.
- X. Ma, F. Zhang and J. Zhu, Preparation of highly developed mesoporous activated carbon fiber from liquefied wood using wood charcoal as additive and its adsorption of methylene blue from solution, Bioresour. Technol., 2014, 164, 1–6 CrossRef CAS PubMed.
- W. Liu and G. Zhao, Effect of temperature and time on microstructure and surface functional groups of activated carbon fibers prepared from liquefied wood, BioResources, 2012, 4, 5552–5567 Search PubMed.
- Y. Huang, E. Ma and G. Zhao, Thermal and structure analysis on reaction mechanisms during the preparation of activated carbon fibers by KOH activation from liquefied wood-based fibers, Ind. Crops Prod., 2015, 69, 447–455 CrossRef CAS PubMed.
- Y. Huang and G. Zhao, Preparation and characterization of activated carbon fibers from liquefied wood by KOH activation, Holzforschung, 2015 DOI:10.1515/hf-2015-0051.
- F. Bouaziz, M. Koubaa, F. Kallel, F. Charri, D. Driss, R. E. Ghorbel and S. E. Chaabouni, Efficiency of almond gum as a low-cost adsorbent for methylene blue dye removal from aqueous solutions, Ind. Crops Prod., 2015, 74, 903–911 CrossRef CAS PubMed.
- K. S. W. Sing, D. H. Everett, R. A. W. Haul, L. Moscou, R. A. Piepotti, J. Rouquérol and T. Siemieniewska, Reporting physisorption data for gas/solid systems with special reference to the determination of surface area and porosity, Pure Appl. Chem., 1985, 57, 603–619 CrossRef CAS.
- X. Du, W. Zhao, Y. Wang, C. Wang, M. Chen, T. Qi, C. Hua and M. Ma, Preparation of activated carbon hollow fibers from ramie at low temperature for electric double-layer capacitor applications, Bioresour. Technol., 2013, 149, 31–37 CrossRef CAS PubMed.
- D. Lozano-Castelló, J. M. Calo, D. Cazorla-Amorós and A. Linares-Solano, Carbon activation with KOH as explored by temperature programmed techniques, and the effects of hydrogen, Carbon, 2007, 45, 2529–2536 CrossRef PubMed.
- J. Miyamoto, H. Kanoh and K. Kaneko, The addition of mesoporosity to activated carbon fibers by a simple reactivation process, Carbon, 2005, 43, 855–857 CrossRef CAS PubMed.
- M. Benadjemia, L. Millière, L. Reinert, N. Benderdouche and L. Duclaux, Preparation, characterization and Methylene Blue adsorption of phosphoric acid activated carbons from globe artichoke leaves, Fuel Process. Technol., 2011, 92, 1203–1212 CrossRef CAS PubMed.
- J. C. Domínguez, M. Oliet, M. V. Alonso, E. Rojo and F. Rodríguez, Structural, thermal and rheological behavior of a bio-based phenolic resin in relation to a commercial resol resin, Ind. Crops Prod., 2013, 42, 308–314 CrossRef PubMed.
- X. Ma, F. Zhang and L. Wei, Effect of wood charcoal contents on the adsorption property, structure, and morphology of mesoporous activated carbon fibers derived from wood liquefaction process, J. Mater. Sci., 2015, 50, 1908–1914 CrossRef CAS.
- M. C. Ncibi, R. Ranguin, M. J. Pintor, V. Jeanne-Rose, M. Sillanp and S. Gaspard, Preparation and characterization of chemically activated carbons derived from Mediterranean Posidonia oceanica (L.) fibres, J. Anal. Appl. Pyrolysis, 2015, 109, 205–214 CrossRef PubMed.
- R. Yang, G. Liu, X. Xu, M. Li, J. Zhang and X. Hao, Surface texture, chemistry and adsorption properties of acid blue 9 of hemp (Cannabis sativa L.) bast-based activated carbon fibers prepared by phosphoric acid activation, Biomass Bioenergy, 2011, 35, 437–445 CrossRef CAS PubMed.
- T. W. Weber and R. K. Chakravorti, Pore and solid diffusion models for fixed-bed adsorbers, AIChE J., 1974, 20, 228–238 CrossRef CAS PubMed.
- S. Senthikumaar, P. R. Varadarajan, K. Porkodi and C. V. Subbhuraam, Adsorption of methylene blue onto jute fiber carbon: kinetics and equilibrium studies, J. Colloid Interface Sci., 2005, 284, 78–82 CrossRef PubMed.
- S. Langergren and B. K. Svenska, Zur Theorie der sogenannten Adsorption gelöster Stoffe, Vetensk. Akad., Handl., 1989, 24, 1–39 Search PubMed.
- Y. S. Ho and G. McKay, Sorption of dye from aqueous solution by peat, Chem. Eng. J., 1988, 70, 115–124 CrossRef.
- K. K. H. Choy, J. F. Porter and G. Mckay, Intraparticle diffusion in single and multicomponent acid dye adsorption from wastewater onto carbon, Chem. Eng. J., 2004, 103, 133–145 CrossRef CAS PubMed.
|
This journal is © The Royal Society of Chemistry 2015 |