DOI:
10.1039/C5RA11600G
(Paper)
RSC Adv., 2015,
5, 70109-70116
In situ synthesis of multidentate PEGylated chitosan modified gold nanoparticles with good stability and biocompatibility†
Received
17th June 2015
, Accepted 11th August 2015
First published on 12th August 2015
Abstract
To realize desirable functions in the rather complex biological systems, a suitable surface coating is desirable for gold nanoparticles, which plays an important role in their colloidal stability and biocompatibility. In this work, a novel multidentate PEGylated chitosan derivative was synthesized by conjugating PEG and dithiolane lipoic acid (LA) to the chitosan backbone. Under reduction conditions, gold nanoparticles (AuNPs) could be in situ formed by the electrostatic interaction between amino groups of the chitosan derivative and gold chloride ions (AuCl4−), with their surface covalently coated by the multidentate PEGylated chitosan via the disulfide bond from LA. After the surface modification, such AuNPs exhibited remarkable colloidal stabilities under extreme conditions, including high salt conditions and complex biological media containing serum. Moreover, the multidentate PEGylated chitosan also provided AuNPs with good biocompatibility and low cytotoxicity, resistance of protein adsorption and anti-phagocytosis by RAW 264.7 cells. This kind of AuNP was expected to be a promising platform for applications in nanomedicine.
Introduction
Gold nanoparticles (AuNPs) have been widely used as a promising platform for a variety of biomedical applications, including drug/gene delivery, biosensing, imaging, diagnostics and photothermal therapy.1–10 However, in these diverse applications, some fundamental challenges will hamper the utilization of AuNPs in vivo.11 Such as the self-aggregation of AuNPs, which can lead them to be quickly cleared by phagocytic uptake and hepatic filtration.12 In addition, the non-specific protein binding onto the surface of AuNPs will also induce undesirable aggregation and further cause easy recognition by the reticuloendothelial system (RES) or mononuclear phagocytic system (MPS), in which NPs are rapidly shuttled out of circulation to the liver, spleen or bone marrow.13,14 While the passive targeting to tumor site of NPs mainly rely on the enhanced permeability and retention (EPR) effect, and the long blood circulation time is prerequisite for NPs that are possible to permeate into tumor tissues through the leaky vasculature.15,16 Therefore, the colloidal stability and biocompatibility are basic criteria of AuNPs for in vivo application. The surface modification of AuNPs is one of the most effective ways to improve the colloidal stability and biocompatibility in biological system.17,18 Ideally, a desirable coating material should not only have high affinity to the NP's surface but also provide NPs with good colloidal stability, biocompatibility and chemical functionality for further functionalization.19–21
FDA approved polyethylene glycol (PEG) was most commonly introduced to modify the NP surface. Due to its excellent hydrophilicity, biocompatibility and nonfouling ability, PEGylated NPs can effectively prevent aggregation and non-specific protein adsorption in biological environment, resulting in a “stealth” behavior and therefore reduce the RES uptake and enhance circulation time.11,22–24 The PEGylation of AuNPs was generally realized by covalent coating of thiol-terminated PEG ligands, because thiols can be anchored onto the gold surface via the chemisorption.25 Recently, multidentate PEG ligands have attracted more attention due to their ability for enhancing the affinity with gold surface and improving the performance of AuNPs in biological application.26,27 Despite these advantages of such multidentate PEG modified gold nanoparticles, their preparation was usually a multistep process with quite complex purification procedures: the nanoparticles were first synthesized using a small molecule surfactant (e.g. citrate) as a capping agent, and then subsequently exchanged the capping agent with PEG ligands as the second step. Hence, the direct synthesis of gold nanoparticles by a single step without post-synthesis manipulation is supposed to give significant advantage in terms of cost and scalability.28,29
As a natural linear polysaccharide, chitosan and its derivatives are widely employed or assessed in biomedical application due to their nontoxic, biocompatible and biodegradable properties.30–32 The rich reactive amino groups of chitosan are useful for chemical modifications. Moreover, it has been reported that chitosan modified nanoparticles could enhance the targeted accumulation and facilitate the tumor uptake due to its positively charged surface.33 Gold nanoparticles coated with chitosan are demonstrated as effective nanocarriers for drug/gene delivery and tumor imaging.34–36 However, large molecular chitosan is difficult to be dissolved under physiological conditions, and the high density amino groups of chitosan surface may also induce nonspecific interactions with serum, causing high cytotoxicity and excessive immune responsive.37 Therefore, the PEGylation of chitosan is a reasonable method to increase the water solubility and biocompatibility of chitosan.38 Lipoic acid (LA) with disulfide bond is produced naturally in the human body, which is believed to have good anti-inflammatory effect.39 In addition, Bai et al. developed LA encapsulated PEG-chitosan particles (LA/PEO/CS) which showed superior anti-inflammatory ability to free LA.40 In this study, we demonstrated a novel biopolymer derivative (PEG-CS-LA) by conjugating PEG and LA molecule with disulfide bond to the chitosan backbone for surface engineering of gold nanoparticles. Under reductive condition, gold nanoparticles were in situ synthesized by one step in the presence of PEG-CS-LA copolymers due to the electrostatic interaction between NH3+ of chitosan and AuCl4−, with simultaneous surface modification by PEG-CS-LA via disulfide bond (Scheme 1). The multidentate PEGylated chitosan surface not only afforded good colloidal stability to the AuNPs but also provide excellent biocompatibility, including low cytotoxicity, resistance of non-specific protein adsorption, and anti-phagocytosis.
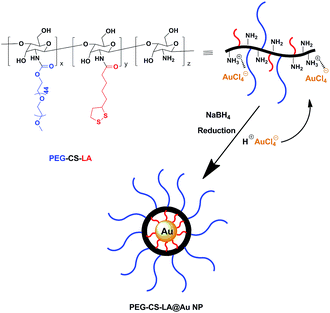 |
| Scheme 1 In situ synthesis of multidentate PEGylated chitosan modified gold nanoparticles. | |
Experimental
Materials
Chitosan (CS, Mw ∼ 10
000, 90% deacetylated degree) was purchased from Nantong Xingcheng Biological Industrial Co., Ltd (Jiangsu, China). Polyethylene glycol (PEG, Mn = 2000), 4-nitrophenyl chloroformate, lipoic acid (LA), 2,4,6-trinitrobenzene sulfonic acid (TNBS) and 1-(3-dimethylaminopropyl)-3-ethylcarbodiimide hydrochloride (EDC·HCl) were purchased from Aladdin reagent Inc. N-Hydroxysulfosuccinimide sodium (sulfo-NHS), hydrogen tetrachloroaurate hydrate (HAuCl4·4H2O), sodium borohydride (NaBH4) and other common regents were purchased from Sinopharm Chemical Reagent Co., Ltd. 3-[4,5-Dimethylthiazol-2-yl]-2,5-diphenyltetrazolium bromide (MTT) was purchased from Sigma-Aldrich. Human umbilical vein endothelial normal cells (HUVEC) and RAW 284.7 cells were purchased from China Center for Typical Culture Collection, and all reagents for the cell culture were directly used after being purchased from Gibco or Dawen Biotech (Hangzhou) Co., Ltd. All water was distilled and subsequently purified to Millipore Milli-Q quality. All glassware used was cleaned by freshly prepared aquaregia solution (HCl/HNO3, 3
:
1). Human platelet poor plasma was donated from volunteers, and the related experiments were performed in compliance with relevant laws and institutional guidelines, and has been approved by the institutional committee.
Synthesis of PEG–nitrophenyl carbonate (PEG–NO2)
The activation of PEG–OH with 4-nitrophenyl chloroformate to generate PEG–NO2, was synthesized according the literature.41 Briefly, PEG (10 g, 5 mmol) and triethylamine (1.4 mL, 10 mmol) were first added in to a three-necked flask with 100 mL acetonitrile. Then, 4-nitrophenyl chloroformate (2 g, 10 mmol) dissolved in 30 mL acetonitrile was added into the flask by a drop funnel. The mixture was reacted under 5 °C for 24 h and further freezed under 20 °C for 12 h to precipitate triethylamine salt. At last, the filtrate was precipitated in ethyl ether to obtain the final product PEG–NO2.
Synthesis of PEGylated chitosan derivatives
To synthesize the PEG-CS-LA derivatives, the CS was first modified with PEG–NO2. Briefly, 2 g of CS (0.2 mmol, ∼12 mmol –NH2) was completely dissolved in 30 mL water by adding HCl. Then, 1.2 g PEG–NO2 (0.6 mmol, ∼5% relative to the molar number of glucosamine units in CS) was added into CS solution, followed by stirring for 24 h at 30 °C. The reaction solution was dialyzed against DI water using a dialysis membrane (MWCO: 3.5 kDa) for two days, and then lyophilized to obtain a yellow powder (PEG-CS copolymer).
The PEG-CS copolymers were further conjugated with lipoic acid via the reaction with carboxyl groups of LA in the presence of EDC–NHS. Briefly, 2.7 g of PEG-CS (0.2 mmol), 0.58 g of EDC·HCl (3 mmol) and 1.3 g of sulfo-NHS (3 mmol) were dissolved in 40 mL distilled water. LA (0.5 g, 2.4 mmol) was dissolved in hot ethanol at 60 °C and then added into PEG-CS aqueous solution, followed by stirring for another 24 h. Finally, the reaction solution was dialyzed against DI water using a dialysis membrane (MWCO: 3.5 kDa) for 3 days and then lyophilized. The lyophilized product was further purified with ethanol to remove the product. The final PEG-CS-LA product was characterized by 1H NMR and FT-IR. The synthesis procedure of CS-LA copolymers was similar to that of PEG-CS-LA.
Determination of the substitution degree of PEG-CS and PEG-CS-LA
The amino substitution degree (SD) of PEG-CS and PEG-CS-LA, defined as the number of PEG or LA groups per 100 amino groups of CS, was determined by TNBS method. Firstly, a calibration curve was made. 0, 50, 100, 200, 500, 800, 1000 μL chitosan solutions (1.0 mg mL−1) were transferred to 10 mL centrifuge tubes and diluted with water to 3 mL, respectively. Every sample was incubated with 2 mL of 4% NaHCO3 and 2 mL of 0.1% TNBS under 37 °C for 2 h. Then, 2 mL of 2 mg mL−1 HCl was added. The ultra-violet absorbance of samples at 344 nm was measured by UV spectroscopy (UV-2502, Shimadzu Corporation). The calibration curve could be obtained by these samples with different CS concentrations.
For determining the SD of PEG-CS and PEG-CS-LA, 3 mL EG-CS and PEG-CS-LA solution (0.1 mg mL−1) was incubated with 2 mL of 4% NaHCO3 and 2 mL of 0.1% TNBS under 37 °C for 2 h. Then, 2 mL of 2 mg mL−1 HCl was added. The absorbance of samples at 344 nm was measured by UV spectroscopy. The substitution degree of PEG-CS and PEG-CS-LA was calculated using the calibration curve.
In situ synthesis of PEG-CS-LA modified AuNPs
The PEG-CS-LA protected AuNPs were prepared directly via only one step. Briefly, 48 mg PEG-CS-LA copolymers were dissolved in 10 mL mixture of THF and water (v/v, 1
:
1). Under the stirring, 6 mL HAuCl4 aqueous solution (1 mM) was dropwise added into the mixture solution, followed by adding 40 mg NaBH4. After stirring at 37 °C for 12 h, the reaction solution was dialyzed against DI water using a dialysis membrane (MWCO: 14 kDa) for 2 days and then purified by centrifuging twice at 15
000 rpm for 10 min to obtain PEG-CS-LA protected AuNPs (PEG-CS-LA@AuNPs). In this work, PEG-CS and CS-LA protected AuNPs were also prepared by the above similar methods.
Instrumentation and measurements
1H NMR analysis was performed on a Bruker DMX500 instrument. FT-IR analysis was performed on a Bruker Vector2 spectrometer. UV-vis analysis was carried out with a UV-vis Shimadzu UV-2505 spectrometer using 1 cm path length quartz cuvettes. Transmission Electron Microscopy (TEM) analysis was performed on a JEM-1230EX TEM operating at 80 kV in bright field mode. Dynamic light scattering (DLS) and zeta potential measure-ments were performed on a Delsa Nano C Particle Analyser (Beckman Coulter Ireland Inc.).
Stability of PEG-CS-LA@Au NPs
The stability of PEG-CS-LA@AuNPs at various situations was tested by DLS and UV-vis spectra collected within a range of 400–800 nm. To determine Au NPs with respect to various NaCl concentrations, the AuNPs were redispersed in normal PBS solution at pH 7.4 which contained 150 mM NaCl and PBS solution with high salt concentration (1000 mM NaCl), respectively. To determine nanoparticles stability in cell growth medium, AuNPs were cultured in cell growth medium DMEM supplemented with and without 10% fetal bovine serum (FBS), respectively.
Protein adsorption resistance ability of PEG-CS-LA@AuNPs
The interaction of AuNPs with various media containing protein was measured by gel electrophoresis. The experiments were done by mixing 5 μL AuNPs with 5 μL of Bovine Serum Albumin (BSA), lysozyme and blood plasma, and 5 μL PBS solution was used as control. After incubation at room temperature for 10 min, the mixture was loaded on a 1% agarose gel buffered with 0.5 × TBE (Tris–borate–EDTA, pH 7.4). Gel electrophoresis was also run in 0.5 × TBE buffer at 120 V constant voltage for 15 min. Gel shift bands were recorded by a digital camera directly.
Cell culture
RAW 264.7 were cultured with regular growth medium consisting of high-glucose DMEM, and HUVEC were cultured with RIPM 1640. All cell growth media were supplemented with 10% fetal bovine serum (FBS), 100 U mL−1 penicillin, and 100 mg mL−1 streptomycin, and cultured at 37 °C in a 5% CO2 humidified environment.
Cytotoxicity assay
Cytotoxicity was performed by the standard MTT assay. To determine cell viability, the human umbilical vein endothelial normal cells were plated at a density of 5 × 10−3 cells per well in a 96-well plate and cultured for 24 h. The medium was replaced with fresh medium containing the AuNPs of varying concentrations (the Au atomic concentration was determined by ICP-MS). After treatment for 24 h, the wells were washed with PBS and the medium was replaced with fresh medium, 20 μL MTT (5 mg mL−1) was added to each well and the cells were further cultured at 37 °C for 4 h. The dark blue formazan crystals generated by the mitochondria dehydrogenase in the live cells were dissolved with 150 μL dimethyl sulfoxide to measure the absorbance at 570 nm by a microplate reader (MODEL 550, Bio Rad).
Cellular uptake of PEG-CS-LA@AuNPs
The cellular uptake of the AuNPs was determined by ICP-MS quantitatively. To determine the Au NPs uptake amount quantitatively, the cells were seeded on a 24-well plate at a certain density (12 × 104 cells per well for RAW 264.7 cells) and cultured for 24 h, and then were incubated with AuNPs (added 20 μL AuNP solution into 480 μL fresh culture medium) of varying concentrations for 12 h with 10% FBS. At a determined time, the cells were washed five times with PBS and harvested by trypsinization. The cell number was calculated by a hemocytometer. The cells were collected by centrifugation, then treated by aquaregia (HCl
:
HNO3 = 1
:
3, volume ratio) for 2 h. The treated solution was diluted to determine the Au concentration by ICP-MS. Dividing by the number of cells, the Au amount per well was calculated.
Results and discussion
Synthesis of PEG-CS-LA amphiphilic copolymers
The detailed synthesis procedure of PEG-CS-LA copolymers is shown in Scheme 2. First, hydrophilic and biocompatible PEG was activated to generate PEG–NO2 which can react with the amino groups of the chitosan, and the chemical structure of PEG–NO2 was confirmed by 1H NMR and IR analysis (Fig. S1†). Further, the PEGylation of chitosan was formed through the reaction of PEG–NO2 with amino groups on chitosan chains. During the process of reaction, the nitrophenol droped from PEG and amide bonds formed between PEG and CS. After the conjugation, the water solubility of chitosan was obviously improved and the PEGylated chitosan can be easily dissolved in aqueous solution. 1H NMR spectroscopy was employed to prove the successful synthesis of the copolymer, as shown in Fig. 1A. Compared to the 1H NMR spectra of chitosan, the peak of PEG-CS at δ 3.5 ppm was assigned to the protons of methylene of PEG. Moreover, the characteristic peaks at δ 7.4 and δ 8.3 ppm of nitrobenzene on PEG–NO2 (Fig. S1A†) could not be observed anymore, indicating the successful PEGylation of chitosan.
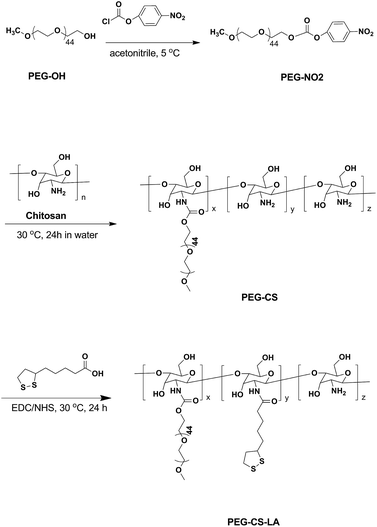 |
| Scheme 2 Schematic synthesis route of PEG-CS-LA amphiphilic copolymers. | |
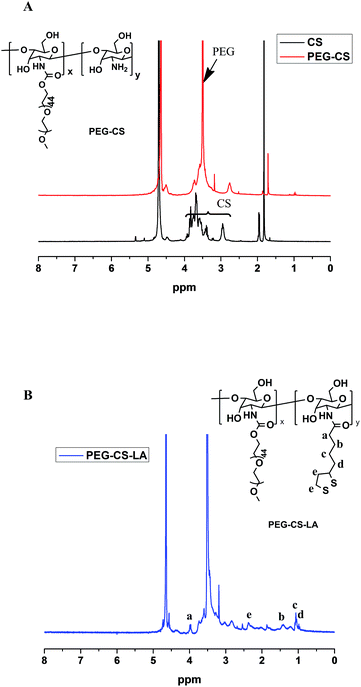 |
| Fig. 1 (A) 1H NMR spectra of CS and PEG-CS in D2O, and (B) 1H NMR spectra of PEG-CS-LA copolymer in mixture of D2O/DMSO-d6 (1 : 1, v/v). | |
Lipoic acid (LA) with a dithiolane was then conjugated to PEG-CS via carbodiimide chemistry, which yielded a multidentate PEGylated chitosan derivative (PEG-CS-LA) as described in Scheme 2. Fig. 1B showed the 1H NMR result of PEG-CS-LA in the mixture of D2O and DMSO-d6. Signals at δ 1.0, δ 1.5, δ 2.4 and δ 4.0 ppm were attributed to the protons of the LA groups. In the FTIR spectra (Fig. S2†), the bands at 1640 cm−1 and 2888 cm−1 were corresponded to the antisymmetric stretching of CO–NH and the stretching of C–O–C on the PEG chain, respectively, while the band at 520 cm−1 was due to the stretching of S–S on LA moieties. All the results above demonstrated the successful synthesis of PEG-CS-LA copolymers. The amino substitution degree (SD) of the PEG-CS-LA copolymer at each step was determined by using a TNBS test. Based on the calculation, the SD of PEG and LA was about 3% and 18%, respectively, which means that the PEG-CS-LA copolymer has ca. 2 PEG and 11 LA groups per molecule. Chitosan derivative modified with a similar substitution of lipolic acid (CS-LA, 9 LA molecules per polymer) but without PEG was also prepared.
In situ synthesis of PEG-CS-LA protected AuNPs
As showed in Scheme 1, the PEG-CS-LA protected AuNPs were directly prepared by only one step. Once the chloroauric acid was added into the PEG-CS-LA solution, the anions of AuCl4− were supposed to bind to the amino groups on the chitosan through the electrostatic interaction. Under the reductive condition, gold nanoparticles were formed and PEG-CS-LA was covalently coated on the surface by the disulfide bond. The UV-vis spectroscopy was first used to confirm the formation of PEG-CS-LA@AuNPs. As showed in Fig. 2A, the characteristic absorption peak at 530 nm indicated the formation of the gold nanoparticles. While the digital image in Fig. 2A displayed a transparent red color of PEG-CS-LA@AuNPs all the time, suggesting the good dispersion of PEG-CS-LA protected AuNPs. TEM was further used to prove the excellent dispersibility of AuNPs after the modification of PEG-CS-LA. Fig. 2B showed the PEG-CS-LA@AuNPs dispersed as single particles without obvious aggregates, demonstrating that PEG-CS-LA can stabilize gold nanoparticles well. The good stability and well dispersibility of AuNPs can be attributed to the hydrophilic PEG-CS surface and its stable modification by the disulfide bond. The average size of gold nanoparticle measured by TEM was about 18 nm. However, DLS result in Fig. 2C showed that PEG-CS-LA@AuNPs had a hydrodynamic diameter of 33 ± 2.1 nm, which was larger than that determined by TEM. These results revealed the core–shell structure of such PEG-CS-LA protected AuNPs, with the gold core size of 18 nm and the PEG-CS shell thickness of about 7.5 nm. It was noted that, after the modification, the zeta potential of such AuNPs was only about +1.4 mV, which was slightly positive and much lower than 35.4 mV of PEG-CS-LA copolymers in water (Fig. S3†). It was probably due to the interaction between amino groups of chitosan and the gold nanoparticle surface, along with the shielding effect by PEG chains. However, such PEGylated surface with weak positive charge is suitable for protein resistance and exhibiting prolonged circulation time for in vivo application. The surface conjugation of gold nanoparticles with PEG-CS shell by LA functional groups was also confirmed by FT-IR analysis. After purification and lyophilisation, the chemical constituents of the AuNPs surface were measured by FT-IR and the spectra in Fig. 2D displayed a similar result as showed in Fig. S2,† suggesting the successful surface modification of PEG-CS-LA copolymers.
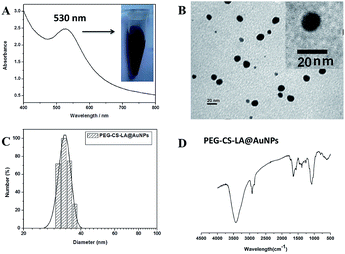 |
| Fig. 2 (A) The UV-vis spectra with a digital image (right, bright red color indicates well dispersion) of PEG-CS-LA modified AuNPs in water; (B) TEM images of PEG-CS-LA@AuNPs; (C) DLS result of PEG-CS-LA@AuNPs in water; (D) FT-IR spectra of lyophilized gold nanoparticles with the modification of PEG-CS-LA. | |
In this study, as comparison, PEG-CS and CS-LA copolymers (showed in Table S1†) were also prepared to modify gold nanoparticles by the same in situ synthesis method. For PEG-CS copolymers showed in Fig. S4,† the color of the reaction solution was dark red and the characteristic absorption peak at 530 nm was also detected by UV spectrum, indicating the formation of gold nanoparticles. However, after a period of time, these AuNPs would gradually precipitate to the bottom of the tube. The result implied the PEG-CS copolymers without functional LA groups could not provide the stable modification and enough water solubility for gold nanoparticles, due to the weak interactions of amino groups with the Au surface. While for the CS-LA, the final color of the reacted solution was grey brown without the observation of characteristic absorption peak from the UV spectra, which indicated failed formation of gold nanoparticles. Without the hydrophilic PEG, CS-LA more tended to self-assemble into polymeric nanoparticles with LA moieties as the hydrophobic domain (average size of 135 nm measured by DLS, data not shown), rather than the formation of AuNPs during the preparation process. Based on the above results, both hydrophilic PEG and LA groups contributed to the stability of PEG-CS-LA@AuNPs. Since the stability of nanoparticles is a prerequisite for most biomedical applications, the next studies were mainly focused on the stable PEG-CS-LA modified NPs.
Colloidal stability of PEG-CS-LA modified AuNPs
For biomedical application, the resistance of aggregation, precipitation, or clearance are essential to the use of NPs for diagnostics or therapy, which allow them to circulate in vivo and accumulate at a specific site via passive or active targeting.42 Therefore, the colloidal stability of PEG-CS-LA modified AuNPs needs to be evaluated. In nanomedicine, conditions and times used to demonstrate the stability of nanoparticles vary widely. Generally, a period of about one day is enough to cover most imaging and therapeutic requirements for targeted and/or diagnostic nanoparticles. Thus, the 24 h time point for stability test in media approaching serum as closely as possible should be used in screening the effective surface modification designed to promote circulation.
The stability of PEG-CS-LA@AuNPs was first studied in normal physiological phosphate buffered saline (PBS, ∼150 mM NaCl, pH 7.4) and PBS solutions with high NaCl concentration of 1000 mM, respectively. As shown in Fig. 3A and B, the UV-vis spectra of PEG-CS-LA@AuNPs were similar to a typical plasmon peak at 530 nm during 24 hours. The result indicated that the PEGylated chitosan surface of these gold nanoparticles had strong resistance to high salinity. The excellent stability in saline solution is attributed to the hydrophilic PEG which can adsorb water molecules via hydrogen bond and form hydration layer that is less susceptible to the ionic strength effect. The stability of AuNPs in biological media containing serum was also investigated by UV-vis spectroscopy, by incubating particles in cell culture medium (DMEM) with or without 10% FBS (Fig. 3C and D). The result revealed that the UV-vis spectrum of the AuNPs kept their shape with the typical peak at 530 nm even after 24 h. Furthermore, the absorbance value of the typical peak at 530 nm were summarized in Fig. S5A† based on the above UV-vis test, which visually showed the no change of the absorbance with the increased incubation time. DLS was also used to follow the size changes of AuNPs to check whether any aggregation had occurred, and the result is displayed in Fig. S5B.† For all four media, there were no big sizes or obvious size changes of PEG-CS-LA@AuNPs within 24 h, which implied the non-aggregation properties of the nanoparticles and the PEG-CS surface could effectively reduce the nonspecific adhesion of proteins in the cell culture medium. Moreover, the AuNPs under these various conditions remained their average diameter with the value ranged from 20 nm to 40 nm. Therefore, such PEG-CS-LA modified gold nanoparticles with sizes less than 100 nm were supposed to evade scavenging by the mononuclear phagocyte system (MPS) in blood circulation and attain an appreciable enhanced permeability and retention (EPR) effect in the site of a solid tumor.43
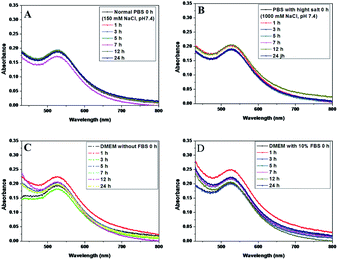 |
| Fig. 3 UV-vis spectra of PEG-CS-LA@AuNPs in (A) normal PBS, (B) PBS solutions with high salt concentrations (pH 7.4), (C) cell culture medium (DMEM) and (D) DMEM medium with 10% PBS. | |
Protein adsorption and phagocytic resistance ability of PEG-CS-LA coated AuNPs
The antifouling property of gold nanoparticles is of great importance for their bio-applications such as biosensing and bioimaging which need to minimize the non-specific background interference.44 Therefore, it is supposed that the PEG-CS-LA surface coating not only conferred colloidal stability but also give nonadhesive properties to AuNPs. The adsorption of various proteins on PEG-CS-LA@AuNPs was monitored by gel electrophoresis, since protein–NP complexes are expected to migrate differently than free AuNPs in a gel.45 In this analytical scheme, the non-specific adsorption of proteins onto the nanoparticles will change the NP's overall electrophoretic mobility which can accelerate or retard the movement of the nanoparticles in a gel.46 Bovine Serum Albumin (BSA) and lysozyme, representative of negatively and positively charged proteins at neutral pH, were selected for the single protein binding analysis. While human platelet poor plasma was chosen for the complex plasma protein adsorption test. As shown in Fig. 4, compared to free AuNPs in PBS, no obvious different band shifts were observed for nanoparticles incubated with BSA, lysozyme and blood plasma. The result demonstrated the strong resistance to nonspecific adsorption of various proteins by the PEGylated chitosan surface. Actually, this strong antifouling property was mainly contributed by the multidentate PEG chains. Despite chitosan was present on the AuNPs surface, its positive amino groups didn't induce nonspecific interactions with proteins due to the shielding effect by PEG chains. However, in cancer therapy, the amino groups from chitosan will be useful for such PEG-CS-LA@AuNPs in the potential use as gene delivery system.
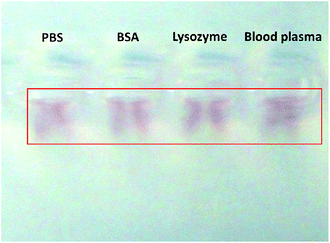 |
| Fig. 4 Gel image of protein adsorption assay. From left to right: 5 μL of PEG-CS-LA@AuNPs mixed with normal PBS; same amount of AuNPs mixed with 5 μL each of BSA and lysozyme, protein concentrations are all 10 mg mL−1 in PBS; same amount of AuNPs mixed with 5 μL of 100% human platelet poor plasma. | |
In consideration of their potential biomedical application, the “stealth” character of such AuNPs referred to as their ability of anti-phagocytosis and avoiding clearance by RES is necessary to be investigated. Here, macrophage cell line RAW 264.7 was used as a model of phagocytes in RES. The endocytosis of PEG-CS-LA@AuNPs by RAW 246.7 cells were quantified by ICP-MS with high detection sensitivity, shown in Fig. 5. After incubation for 12 h, the cell uptake was very low and only increased moderately from 226 ng to 386 ng per well as the concentration of feed AuNPs greatly increased from 55 μg mL−1 (2.75 × 104 ng per well) to 220 μg mL−1 (11 × 104 ng per well). It was noted that, even take the case with the highest concentration of 220 μg mL−1 for example, less than 1% of the fed AuNPs were uptaken by cells. It should be accounted for the inherent very low uptake of AuNPs as restricted by the multidentate PEG surface. Thus, this PEG-CS-LA coating is supposed to prevent clearance of AuNPs by RES and ensure them to circulate in vivo and accumulate at the specific site via passive targeting.
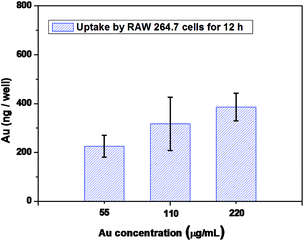 |
| Fig. 5 Cell uptake of PEG-CS-LA@AuNPs evaluated by ICP-MS after RAW 264.7 cells incubation with AuNPs for 12 h with different Au concentrations. Error bars represent mean ± SD (n ≥ 3). | |
Cytotoxicity of PEG-CS-LA modified AuNPs
For bioapplication, the low toxicity is necessary for gold nanoparticles. The in vitro cytotoxicity of these PEG-CS-LA modified AuNPs was evaluated by MTT assay, using human umbilical vein endothelial normal cells (HUVEC) as a normal cell model. As shown in Fig. 6, the PEG-CS-LA@AuNPs did not show obvious cytotoxicity to cells even at a high Au concentration (550 μg mL−1). Preliminary toxicity result suggested excellent biocompatibility of these AuNPs was attributed to the inherent biocompatibility of PEGylated chitosan surface. Such biocompatible surface will help AuNPs to lower the risk of side-effect to normal tissues during the long circulation in blood. Thus, these gold nanoparticles coated with PEG-CS-LA are promising in vivo applications with better performance.
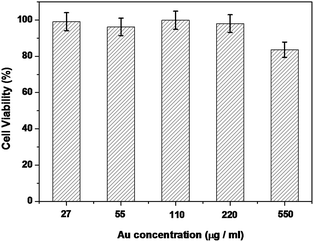 |
| Fig. 6 Cytotoxicity of HUVEC cells evaluated by MTT assays after incubation with PEG-CS-LA@AuNPs with different Au concentrations for 24 h, error bars represent mean ± SD (n ≥ 3). | |
Conclusions
In this work, we developed a facile strategy to synthesize gold nanoparticles with good stability and biocompatibility. Under reductive condition, gold nanoparticles were directly formed by one step in the presence of PEG-CS-LA copolymers due to the electrostatic interaction between NH3+ of chitosan and AuCl4−, with PEG-CS-LA covalently coating onto the surface via disulfide bond from functional LA moieties. Such PEG-CS-LA modified AuNPs displayed good colloidal stability in complex biological media and at high salt concentrations due to their multidentate PEGylated chitosan surface. The inherent excellent biocompatibility of PEG-CS-LA coating also conferred properties of low toxicity, strong protein resistance and anti-phagocytosis by macrophages to AuNPs, which were essential for NPs to escape the clearance by RES, obtain longcirculating time and have enough chance for accumulating to specific site via EPR effect. Therefore, these PEG-CS-LA@AuNPs with good stability and biocompatibility possess promising potential application in nanomedicine for diagnostics and therapy.
Acknowledgements
This work was supported by the Natural Science Foundation of China (NSFC 5103031), Sichuan Province Science and Technology Support Program (2014SZ0128, 2015SZ0122), China Postdoctoral Science Foundation Funded Project (2015M570783) and Yong teacher's research startup foundation of Sichuan University (2014SCU11026).
Notes and references
- S. S. Agasti, A. Chompoosor, C.-C. You, P. Ghosh, C. K. Kim and V. M. Rotello, J. Am. Chem. Soc., 2009, 131, 5728–5729 CrossRef CAS PubMed.
- B. Kim, G. Han, B. J. Toley, C. Kim, V. M. Rotello and N. S. Forbes, Nat. Nanotechnol., 2010, 5, 465–472 CrossRef CAS PubMed.
- N. L. Rosi, D. A. Giljohann, C. S. Thaxton, A. K. Lytton-Jean, M. S. Han and C. A. Mirkin, Science, 2006, 312, 1027–1030 CrossRef CAS PubMed.
- C. M. Jewell, J. M. Jung, P. U. Atukorale, R. P. Carney, F. Stellacci and D. J. Irvine, Angew. Chem., 2011, 123, 12520–12523 CrossRef PubMed.
- D. Zheng, D. A. Giljohann, D. L. Chen, M. D. Massich, X.-Q. Wang, H. Iordanov, C. A. Mirkin and A. S. Paller, Proc. Natl. Acad. Sci. U. S. A., 2012, 109, 11975–11980 CrossRef CAS PubMed.
- D. Liu, W. Chen, K. Sun, K. Deng, W. Zhang, Z. Wang and X. Jiang, Angew. Chem., Int. Ed., 2011, 50, 4103–4107 CrossRef CAS PubMed.
- K. Saha, S. S. Agasti, C. Kim, X. Li and V. M. Rotello, Chem. Rev., 2012, 112, 2739–2779 CrossRef CAS PubMed.
- H. Wang, T. B. Huff, D. A. Zweifel, W. He, P. S. Low, A. Wei and J.-X. Cheng, Proc. Natl. Acad. Sci. U. S. A., 2005, 102, 15752–15756 CrossRef CAS PubMed.
- G. von Maltzahn, A. Centrone, J. H. Park, R. Ramanathan, M. J. Sailor, T. A. Hatton and S. N. Bhatia, Adv. Mater., 2009, 21, 3175–3180 CrossRef CAS PubMed.
- Y. C. Yeh, B. Creran and V. M. Rotello, Nanoscale, 2012, 4, 1871 RSC.
- J. V. Jokerst, T. Lobovkina, R. N. Zare and S. S. Gambhir, Nanomedicine, 2011, 6, 715–728 CrossRef CAS PubMed.
- X. Duan and Y. Li, Small, 2013, 9, 1521–1532 CrossRef CAS PubMed.
- S.-D. Li and L. Huang, Mol. Pharmaceutics, 2008, 5, 496–504 CrossRef CAS PubMed.
- A. Albanese, P. S. Tang and W. C. W. Chan, Annu. Rev. Biomed. Eng., 2012, 14, 1–16 CrossRef CAS PubMed.
- H. Maeda, J. Wu, T. Sawa, Y. Matsumura and K. Hori, J. Controlled Release, 2000, 65, 271–284 CrossRef CAS.
- S. M. Moghimi, A. C. Hunter and J. C. Murray, Pharmacol. Rev., 2001, 53, 283–318 CAS.
- Y. Gong and F. M. Winnik, Nanoscale, 2012, 4, 360–368 RSC.
- W. Yang, L. Zhang, S. L. Wang and A. D. White, Biomaterials, 2009, 30, 5617–5621 CrossRef CAS PubMed.
- X. Liu, H. Li, Q. Jin and J. Ji, Small, 2014, 21, 4230–4242 Search PubMed.
- X. Liu, J. Cao, H. Li, J. Li, Q. Jin, K. Ren and J. Ji, ACS Nano, 2013, 7, 9384–9395 CrossRef CAS PubMed.
- X. Liu, H. Huang, G. Liu, W. Zhou, Y. Chen, Q. Jin and J. Ji, Nanoscale, 2013, 5, 3982–3991 RSC.
- L. E. van Vlerken, T. K. Vyas and M. M. Amiji, Pharm. Res., 2007, 24, 1405–1414 CrossRef CAS PubMed.
- M. D. Howard, M. Jay, T. D. Dziublal and X. L. Lu, J. Biomed. Nanotechnol., 2008, 4, 133–148 CrossRef CAS PubMed.
- M. Zheng, F. Davidson and X. Huang, J. Am. Chem. Soc., 2003, 125, 7790–7791 CrossRef CAS PubMed.
- K. E. Sapsford, W. R. Algar, L. Berti, K. B. Gemmill, B. J. Casey, E. Oh, M. H. Stewart and I. L. Medintz, Chem. Rev., 2013, 113, 1904–2074 CrossRef CAS PubMed.
- X. Liu, N. Huang, H. Li, H. Wang, Q. Jin and J. Ji, ACS Appl. Mater. Interfaces, 2014, 6, 5657–5668 CAS.
- X. Liu, N. Huang, H. Wang, H. Li, Q. Jin and J. Ji, Biomaterials, 2013, 34, 8370–8381 CrossRef CAS PubMed.
- I. E. Dunlop, M. P. Ryan, A. E. Goode, C. Schuster, N. J. Terrill and J. V. M. Weaver, RSC Adv., 2014, 4, 27702–27707 RSC.
- G. Park, D. Seo, I. S. Chung and H. Song, Langmuir, 2013, 29, 13518–13526 CrossRef CAS PubMed.
- G. Liu, Q. Luo, H. Gao, Y. Chen, X. Wei, H. Dai, Z. Zhang and J. Ji, Biomater. Sci., 2015, 3, 490–499 RSC.
- H. Zhou, W. Yu, X. Guo, X. Liu, N. Li, Y. Zhang and X. Ma, Biomacromolecules, 2010, 11, 3480–3486 CrossRef CAS PubMed.
- F. Q. Hu, L. N. Liu, Y. Z. Du and H. Yuan, Biomaterials, 2009, 30, 6955–6963 CrossRef CAS PubMed.
- S. Mao, W. Sun and T. Kissel, Adv. Drug Delivery Rev., 2010, 62, 12–27 CrossRef CAS PubMed.
- D. R. Bhumkar, H. M. Joshi, M. Sastry and V. B. Pokharkar, Pharmaceut. Res., 2007, 24, 1415–1426 CrossRef CAS PubMed.
- L. Han, J. Zhao, X. Zhang, W. Cao, X. Hu, G. Zou, X. Duan and X. J. Liang, ACS Nano, 2012, 6, 7340–7351 CrossRef CAS PubMed.
- I. Sun, J. H. Na, S. Y. Jeong, D. E. Kim, I. C. Kwon, K. Choi, C. H. Ahn and K. Kim, Pharm. Res., 2014, 31, 1418–1425 CrossRef CAS PubMed.
- L. Casettari, D. Vllasaliu, E. Castagnino, S. Stolnik, S. Howdle and L. Illum, Prog. Polym. Sci., 2012, 37, 659–685 CrossRef CAS PubMed.
- F. Q. Hu, Y. Y. Zhang, J. You, H. Yuan and Y. Z. Du, Mol. Pharmaceutics, 2012, 9, 2469–2478 CrossRef CAS PubMed.
- R. Weerakady, P. Fagan and S. L. Kosaraju, Int. J. Pharm., 2008, 357, 213–218 CrossRef PubMed.
- M.-Y. Bai and Y.-M. Hu, J. Microencapsulation, 2014, 31, 373–381 CrossRef CAS PubMed.
- G.-Y. Liu, M. Li, C.-S. Zhu, Q. Jin, Z.-C. Zhang and J. Ji, Macromol. Biosci., 2014, 14, 1280–1290 CrossRef CAS PubMed.
- B. S. Joseph, Langmuir, 2014, 30, 9625–9636 CrossRef PubMed.
- K. Miyata, R. J. Christie and K. Kataoka, React. Funct. Polym., 2011, 71, 227–234 CrossRef CAS PubMed.
- F. Zhang, E. Lee, F. Amin, P. Rivera-Gil, F. Yang and P. Mulvaney, Small, 2011, 7, 3113–3127 CrossRef CAS PubMed.
- X. S. Liu, H. Y. Huang, Q. Jin and J. Ji, Langmuir, 2011, 27, 5242–5251 CrossRef CAS PubMed.
- R. E. Anderson and W. C. W. Chan, ACS Nano, 2008, 2, 1341–1352 CrossRef CAS PubMed.
Footnote |
† Electronic supplementary information (ESI) available: Fig. S1 and S2 shows the 1H NMR and FTIR results, Fig. S3 showed the zeta-potential results, Fig. S4 shows PEG-CS and CS-LA modified AuNPs, Table S1 shows the properties of PEG-CS-LA, PEG-CS and CS-LA copolymers, and Fig. S5 showed the changes of the UV absorbance and sizes of PEG-CS-LA@AuNPs. See DOI: 10.1039/c5ra11600g |
|
This journal is © The Royal Society of Chemistry 2015 |