DOI:
10.1039/C5RA10926D
(Paper)
RSC Adv., 2015,
5, 58663-58668
Rheological properties of the polysaccharide–protein complex from longan (Dimocarpus longan Lour.) pulp
Received
9th June 2015
, Accepted 23rd June 2015
First published on 24th June 2015
Abstract
Crude longan polysaccharide (CLP) was extracted from longan (Dimocarpus longan Lour.) pulp. Its chemical composition was determined by chemical analysis, high-performance liquid chromatography (HPLC) and gel permeation chromatography (GPC). The flow and viscoelastic behavior of a CLP solution was investigated by carrying out steady shear and small amplitude oscillatory shear (SAOS) experiments, respectively. The results showed that the CLP solution was a polysaccharide–protein complex. The rheology experiments showed that it underwent a pseudoplastic flow at various shear rates (0.1–100 s−1). Both the flow behavior and viscoelastic behavior of CLP were influenced by cations such as Na+ and Ca2+. Increases of the apparent viscosity, G′ and G′′ accompanied the addition of Na+ and Ca2+. A polysaccharide named LPB-2-M was obtained from isolation and purification of CLP; this polysaccharide was shown to consist of a single component due to the narrow molecular weight distribution, which was evident by the polydispersity index (PDI = Mw/Mn). The average molecular weight (Mw) of LPB-2-M was 80 kDa.
1. Introduction
Bioactive polysaccharides have recently attracted much attention due to their broad spectra of therapeutic properties, including anti-oxidation, immunomodulation, anti-tumour and hypoglycemic activities,1 and relatively low toxicity. Polysaccharides from animals, plants and microbial fermentation extracts are an interesting source of additives for high-value healthy foods and the pharmaceutical industry. They can promote the healthy growth and development of living organisms, and have been widely studied in recent years for their biological, chemical and physical properties.2
Longan (Dimocarpus longan Lour.), which belongs to the Sapindaceae family, is an important tropical fruit and is mainly planted in Southeast Asia, including in Vietnam, Thailand, and the south of China.3–7 Its taste, nutrition and flavor are welcomed by consumers worldwide. The fresh seed and pericarp of longan have been used as a traditional Chinese medicine since ancient times. Many reports have showed them to have highly beneficial health effects such as anti-oxidation,7,8 anti-tumor,8,9 anti-glycation,3 immunomodulatory5,10 and anticancer activities.11 Methods using various physical fields such as ultrasonic-assisted,12 microwave-assisted,13 and pressure-assisted extraction1 have been widely used to improve their extraction. The chemical structure of their polysaccharides has also been discussed. Yang et al.14 used gas chromatography (GC) and gas chromatography/mass spectrometry (GC/MS) to determine the chemical structure of longan fruit pericarp polysaccharides; they were shown to be comprised of L-arabinofuranose (L-Araf, 32.8%), D-glucopyranose (D-Glcp, 17.6%), D-galactopyranose (D-Galp, −33.7%) and D-galacturonic acid (GalpA, 15.9%), with the backbone consisting of →5)-L-Araf-(1→,→6)-D-Glcp-(1→,→3)-D-Galp-(1→,→3)-D-GalpA-(1→and→6)-D-Galp-(1→ with a molar proportion of 2
:
1
:
1
:
1
:
1. Nuclear magnetic resonance (NMR) spectra indicated that the configuration of anomeric carbon in the glucose residual was the α-form and confirmed to be (1→6)-α-D-glucan by the chemical shift of C6.11
Polysaccharides used in thickeners, stabilizers, gelling agents, emulsifiers, flavor fixation agents and texture modifiers, which perform key roles in food systems, have been evaluated by having their rheological properties measured.15 The study of the rheological properties of longan polysaccharide solutions would be helpful to stabilize longan drinks, which is important to the development and quality control of these drinks. Iagher et al.16 showed the polysaccharides from mango (Mangifera indica L.) pulp to have a weak gel behavior. Guo et al.17 studied the rheological behavior of an alkaline extracted gel fraction (AEG) of psyllium polysaccharides and found that Ca2+ has a significant influence on the gel properties. The rheological properties of acidic polysaccharides from boat-fruited sterculia seeds were also discussed by Wu et al.18 Aspects of the molecular structure of these natural polysaccharides, including the molecular weight, degree of branching, and the identities of the functional groups,19 are main factors affecting the rheological behavior. Furthermore, temperature, concentration of polysaccharide and presence of sugars or salts also affect the rheological properties of polysaccharides.20–22
To the best of our knowledge, there is no published study of the rheological properties of longan polysaccharides. The aim of this work is therefore to study the rheological properties of longan polysaccharides for further applications, such as jelly. We used ethanol precipitate to prepare water-soluble polysaccharides. The rheology properties of the polysaccharide were studied by using a dynamic shear rheometer, and structural information is also presented to explain this phenomenon.
2. Materials and methods
2.1. Plant materials and sample preparation
Shixia longan (Dimocarpus longan Lour. harvested from Guangdong Province) pulp was dried in hot air and then soaked four times in 80% (v/v) ethanol for 48 hours, and then dried before grinding it into a 200 mesh powder, which was subjected to further analysis.
2.2. Chemicals
Glucose, phenol and sulphuric acid were obtained from Guangzhou Reagent Co. (Guangzhou, China). Xylose (Xyl), arabinose (Ara), glucose (Glc), galactose (Gal), fructose (Fru), mannose (Man), galacturonic acid (GalA) and glucuronic acid (GlcA) standards were purchased from Sigma Chemical Co (St. Louis, MO, USA). All the other chemicals used were of analytical grade.
2.3. Quantitative extraction of longan polysaccharide
Extraction of polysaccharides by hot water and alcohol precipitation was slightly modified according to Yi's methods.23 In brief, 10 g of longan pulp powder were dispersed in 100 mL of distilled water and heated three times to 90 °C for two hours each. The extract was filtered through four-fold gauze, and then centrifuged at 3000 rpm for 10 minutes. The supernatant was concentrated to 100 mL using a rotatory evaporator (LR4002, Heidolph, Germany) at 65 °C under vacuum. Anhydrate ethanol (400 mL) was added to the concentrated extract to precipitate polysaccharides overnight at 4 °C. The pellet was gathered after centrifugation, and successively washed with anhydrate ethanol, absolute ether and acetone and then dried at 45 °C under vacuum. The total polysaccharide content was determined by using the phenol–sulphuric acid method and expressed as glucose equivalents. The hexuronic acid content was determined by using the Blumenkrantz and Asboe-Hansen method and expressed as glycuronic acid equivalents. The protein content was estimated using Folin–Ciocalteu's reagent and expressed as bovine serum albumin equivalents.23
2.4. Fractionation of the polysaccharide
The proteins in the crude longan polysaccharide (CLP) were removed using the Sevag reagent24 and decolored by H2O2, after removal of the Sevag reagent. Two kinds of chromatography (anion-exchange DEAE-cellulose chromatography and Sephacryxl S-400 HR gel chromatography) were used to purify CLP. The major fraction, named LPB-2-M, was obtained based on the elution curve for further analysis. The yield of LPB-2-M was 46.7%.
2.5. Measurement of molecular weight
The relative molecular weight of the purified polysaccharide was determined by using an Agilent HP-GPC 220 system, which was equipped with three X-stream columns, and a differential refractive index (RID) detector. All columns were maintained at a constant 40 °C with a flow rate of 1 mL min−1. The LPB-2-M sample was dissolved in pure water, and then filtered through a 0.45 μm membrane under pressure. A series of dextran standards (with molecular weights of 10 kDa, 40 kDa, 70 kDa, and 500 kDa) were used to determine the molecular weight of the sample.
2.6. Monosaccharide measurement
LPB-2-M (10 mg) was hydrolyzed by 5 mL of 3 M trifluoroacetic acid at 100 °C for 6 h. Derivatization of the released monosaccharides was then carried out by the 1-phenyl-3-methyl-5-pyrazolone reagent (PMP). The PMP derivatives were loaded onto an HPLC-2010 chromatography system (Shimadzu, Shanghai, China) equipped with an ODS-C18 capillary column and an SPD detector. HPLC chromatography analysis was carried out with a column temperature of 40 °C, detector wavelength of 245 nm, and an injection volume of 10 μL; the mobile phase was a mixed solution of acetonitrile and phosphate buffer (pH 6.8) at a ratio of 15 to 85 and at a flow rate of 1 mL min−1.25
2.7. Rheological measurement
2.7.1. Preparation of samples. Water-soluble polysaccharide solutions at different concentrations (3.0%, 5.0%, 10%, w/v) were prepared by dissolving the dried CLP powder in distilled water and the samples (5%, w/v) in different concentrations of Na+ (0 mM, 50 mM, 150 mM, 250 mM) and different concentrations of Ca2+ (0 mM, 50 mM, 150 mM, 250 mM). All of the samples were stirred for 2 h.
2.7.2. Rheological experiment. Dynamic shear rheological properties such as storage modulus (G′), loss modulus (G′′) and complex viscosity (η*) of the gels were measured under small-amplitude oscillatory shear using a parallel geometry with a diameter of 50 mm in an MCR 302 rheometer (Anton paar, Austria, DE). The dynamics measurements were performed at a strain value of 0.02 (2%) (within the linear viscoelastic regime) in a frequency range of 0.1–100 rad s−1. Steady shear tests were also carried out to assess the flow behavior of the polysaccharide samples using the same apparatus described above. Flow curves were measured at a shear rate increasing from 0.1 to 100 s−1 at 25 °C.
3. Results and discussion
3.1. Chemical composition of longan polysaccharides
Water-soluble polysaccharides were extracted from longan pulp by hot water and alcohol precipitation in this work. The crude longan polysaccharides (CLPs) were determined to consist of 80.72% polysaccharides, 5.27% uronic acids and 13.10% proteins, which is similar to the composition previously reported.5 Yi et al. have studied these polysaccharide–protein complexes, and found that they exhibit good immunomodulatory activities.23 Different ratios of carbohydrates to proteins may affect the bioactivity; for example, polysaccharide–protein complexes from L. barbarum (LBP) with high protein content are able to activate T cells, whereas those with high carbohydrate content could not.26 The effects of different carbohydrate/protein ratios on the immunomodulatory activities of longan polysaccharide–protein complexes were also discussed by Yi et al..
3.2. Major components of CLP
The CLP that was purified was named LPB-2-M. The monosaccharide component and molecular weight distribution of LPB-2-M are shown in Fig. 1 and 2, respectively. As seen in Fig. 1, LPB-2-M was observed to contain ribose (21 min), rhamnose (23 min), fructose (24 min), glucose (39 min), arabinose (43 min), galactose (51 min) and xylose (55 min). This result is different from those of other reports27 in which LPS-N was shown to consist of xylose and glucose, LPS-A1 was shown to be composed of rhamnose, xylose, arabinose and galactose, and LPS-A2 only contained rhamnose. These differences are due to different methods of extraction and fractionation.
In Fig. 2, a single peak at 24 min was observed, which means that LPB-2-M is a single species with a constant molecular weight. The range of the molecular weights was 23 kDa to 410 kDa, and the polydispersity (Mw/Mn) obtained for LPB-2-M was 1.03. The average molecular weight (Mw) was 80 kDa, which accounted for approximately 90% of the total. Molecular weights of polysaccharides extracted from longan according to other reports were 13.8 kDa for neutral longan polysaccharide LPS-N, and 1382 kDa and 571 kDa for two acid polysaccharides (LPS-A1 and LPS-A2) from longan pulp.27 The weight-average molar mass of LPI was also reported to be 14 kDa, and its polydispersity (Mw/Mn) was 1.36.5,6
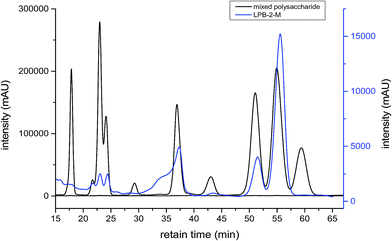 |
| Fig. 1 HPLC chromatograph of derivate of mixed monosaccharide and LPB-2-M. | |
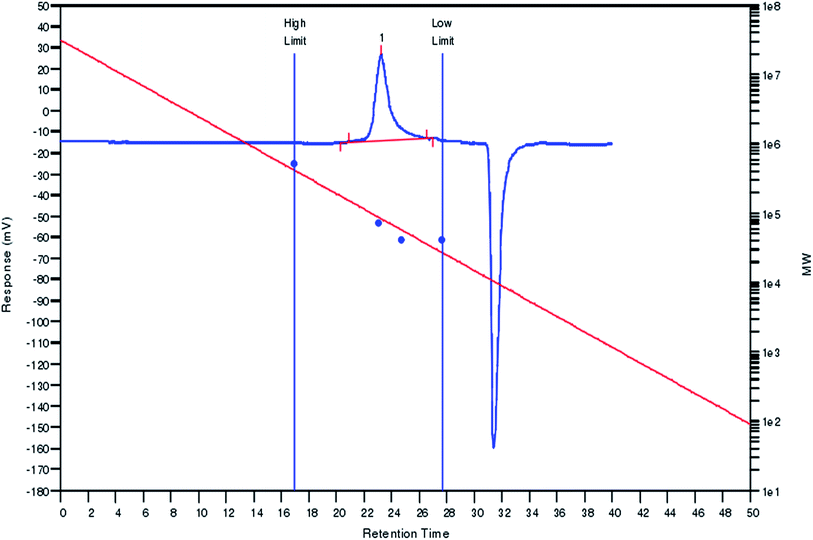 |
| Fig. 2 GPC chromatograph of LPB-2-M. | |
3.3. Rheological properties
3.3.1. Effect of CLP concentration. Steady shear and small amplitude oscillatory shear (SAOS) measurements of CLP at concentrations of 3, 5 and 10% (w/v) at 25 °C were taken to study its rheological behavior. The dispersion at low concentration always shows erratic signals because of its very low viscosity or due to the parallel plate geometry limit. The mechanical spectrum (frequency dependence of G′, G′′ and η*) of crude longan polysaccharides (CLP) (5% w/w) at 25 °C is shown in Fig. 3. For this sample, the complex viscosity η* was observed to decrease linearly with increasing frequency with a slope of −0.92 on a double logarithmic scale, showing a strong shear thinning behavior with a power-law index of 0.08. This slope is steeper than the −0.76 value described by Morris28 and it was used to describe the “weak gel” characteristics of a polysaccharide gel formed by overlapping and entangled flexible random coil chains. G′ was consistently about 5 times higher than G′′ in the frequency range studied, and both moduli showed little frequency dependency (G′ ∼ ω0.07, G′′ ∼ ω0.17), exhibiting a weak gel-like behavior. Steady shear viscosity (η) determined from rotational measurements was substantially lower than η* from SAOS measurements. This violation of the Cox–Merz rule29 implies a structure that remains intact under small-amplitude oscillations, but may be degraded by the shear applied in the rotational measurements; this explanation should be further verified by recovery experiments in future.
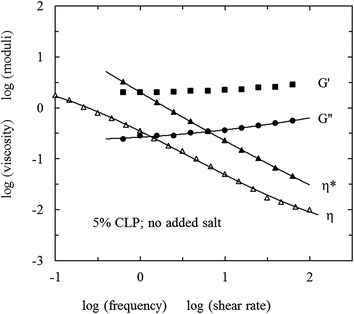 |
| Fig. 3 Frequency dependence of G′ (■), G′′(●) and η* (▲) and shear-rate dependence of η (△) for 5% CLP in water at 25 °C. | |
The samples at the relatively low (3%) and high (10%) concentrations exhibited qualitatively similar rheological behavior, but a large quantitative difference in viscosity and the two moduli. Fig. 4 shows the double logarithmic curve of log
G′ and log
G′′ versus log
C. Comparisons were made at 1 rad s−1 (log frequency = 0). Fig. 4 shows a linear increase of G′ and of G′′ with C on a double logarithmic scale, with the slope of G′ being higher than that of G′′, leading to an increase in the separation between G′ and G′′ with increasing concentration. tan
δ represents G′′ normalized by G′ (i.e., G′′/G′), which is a sensitive indicator of crosslinking. For an ideal network, tan
δ = 0, and a decrease in tan
δ indicates network formation and the elimination of inhomogeneities.30 In fact, the value of tan
δ decreases from 0.42 to 0.15 with increasing concentration in the range measured, which indicates an improved network being forming. This improvement is due to the stronger interactions between the molecules with increasing CLP concentration. In addition, both slopes are much higher than the typical values of normal gelling polysaccharides.31
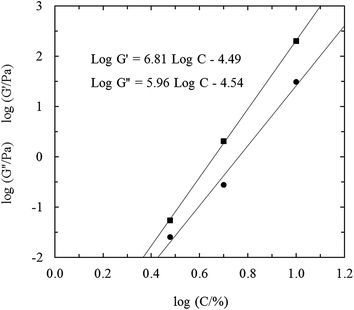 |
| Fig. 4 Variation of log G′ (■) and log G′′ (●), measured at 1 rad s−1 and 25 °C, with log C for CLP in water at concentrations of C = 3, 5 and 10%. | |
The double logarithmic curves of log
η* and log
η versus log
C are shown in Fig. 5. The separation between log
η* and log
η decreased with decreasing concentration, extrapolating to zero at ∼3% (i.e., progressively smaller departure from Cox–Merz superposition as the concentration is lowered). Although the slope of log
η versus log
C (∼4.9) is much lower than the corresponding value of ∼6.7 for η*, it is still substantially higher than the slope of ∼3.4 normally observed for solutions of entangled polysaccharide coils.32
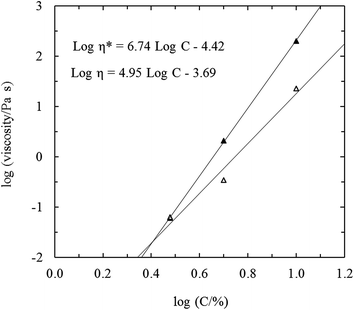 |
| Fig. 5 Variation of log η* (▲) at 1 rad s−1 and log η (△) at 1 rad s−1 with log C for CLP in water (25 °C) at concentrations of 3, 5 and 10%. | |
3.3.2. Effect of Na+/Ca2+ concentration. Addition of salt to 5% CLP increased both the moduli, depending on salt concentration and type (Fig. 6). The monovalent ion Na+ increased G′ and G′′ at concentrations above 50 mM, but decreased the moduli slightly at concentrations below 50 mM. However, this was not the case for divalent ions. Immediate sharp increases in the moduli were observed at low concentrations (<50 mM) of Ca2+, and they continued to increase with Ca2+ concentration (>50 mM), but more gradually than at low Ca2+ concentrations. The moduli were observed to be higher with Ca2+ than with Na+ at any given concentration, and specifically two orders of magnitude higher at 250 mM. Interestingly, tan
δ almost didn't change with salt concentration and type, indicating that the network structure does not change or at least not significantly with addition of salt.
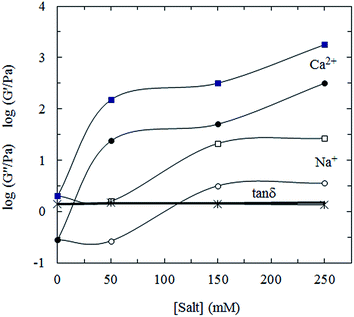 |
| Fig. 6 Variation of log G′ (squares) and log G′′ (circles), measured at 1 rad s−1, with molar concentrations of added NaCl (open symbols) or CaCl2 (filled symbols) for 5% CLP at 25 °C. | |
Considering the components of CLP, it is reasonable to suggest that the related polysaccharide is negatively charged due to the presence of uronic acids. The anionic polysaccharide may form a strong interaction with salt, which causes a change in the polysaccharide conformation; this subject, however, is beyond the scope of this paper and the detailed mechanism will be further studied by dynamic light scattering and use of a zetasizer, and reported in another paper. As inferred from the effects of salt on rheological behavior, CLP in solution may have ordered helical structures, as many polysaccharides (xanthan, gellan, carrageenan, etc.) can adopt.33 For disordered polymers, addition of salt would decrease the dimensions of the coils, leading to reduction in viscosity and the dynamic moduli.31 It is well known that charged polysaccharides and proteins could form complexes by using electrostatic interactions. The properties of the complexes, such as their gelling properties, can be improved by having two types of biopolymers interact.34–40 This could be the reason that the increase in the moduli is much higher than for some previously reported polysaccharides, as was mentioned in the last section. On the other hand, the electrostatic repulsion between the polysaccharide molecules can act as a barrier preventing the association between the molecules. This barrier, however, can be destroyed by carrying out charge screening when adding salt, thus promoting the association between the polysaccharide molecules. Such charge screening, however, also weakens the electrostatic interactions between polysaccharides and proteins, which explains the slight decrease in the moduli when NaCl was added (<50 mM). A further increase of the concentration of the NaCl added promotes the association between the polysaccharides, leading to the increase in the moduli. As a divalent ion, Ca2+ could link the molecular structure by possible ion bridging, making the association between the polysaccharide molecules stronger. As a result, the addition of Ca2+ leads to higher moduli and viscosity than does the addition of Na+.
The response of CLP to salt is broadly similar to that of gellan,31 which is anionic and forms double helices at room temperature. Monovalent (Group I) metal ions promote gelation by binding to individual double helices and thus suppressing the electrostatic barrier to helix–helix association. Binding occurs only above a minimum critical cation concentration, which for Na+ is ∼65 mM (the concentration at which the moduli and viscosity of CLP increase steeply on progressive addition of NaCl). Ca2+ (and other divalent metal ions) promotes gelation by binding between helices, and there is an immediate sharp increase in moduli on progressive addition of CaCl2 to gellan solutions (as seems also to occur with CLP).
These apparent similarities may be coincidental, but it would be worth exploring the effect of salts on CLP in greater detail, by studying a few more concentrations in and around the regions of steep increase and inflexions in Fig. 6.
4. Conclusion
Crude longan polysaccharide (CLP) was extracted from longan (Dimocarpus longan Lour.) pulp. In order to promote the wide use of CLP, the chemical composition and rheological properties have been described. The chemical composition was determined by chemical analysis, high-performance liquid chromatography (HPLC) and gel permeation chromatography (GPC). The flow and viscoelastic behaviors of a CLP solution was investigated by carrying out steady shear and small amplitude oscillatory shear (SAOS) experiments, respectively (Fig. 7).
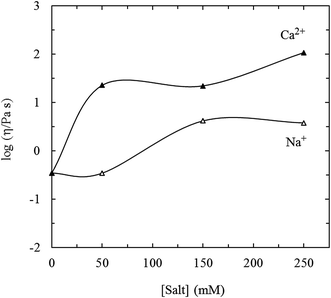 |
| Fig. 7 Variation of log η (measured at 1 rad s−1) with molar concentrations of added NaCl (△) and of CaCl2 (▲) for 5% CLP at 25 °C. | |
Water-soluble polysaccharides (CLP, and LPB-2-M) were isolated from Dimocarpus longan Lour. All of them contain major sugars and uronic acid while CLP also contains protein. The monosaccharide analysis confirmed that LPB-2-M consists of monosaccharides such as ribose, rhamnose, fructose, glucose, arabinose, galactose and xylose. The CLP solution shows a weak gel-like behavior, but the structure can be degraded by application of a steady shear. An ordered structure was inferred based on the response of the rheological properties to the addition of salt. Na+ and Ca2+ can increase both moduli and viscosity, but Ca2+ works more efficiently.
Acknowledgements
This work was financially supported by NSFC (31301554) and the national tropical crops engineering development of the Reconstruction Project of the Chinese National Engineering Research Center (no. 2011FU125Z09).
References
- K. N. Prasad, J. Hao, J. Shi, T. Liu, J. Li, X. Wei, S. Qiu, S. Xue and Y. Jiang, Innovative Food Sci. Emerging Technol., 2009, 10, 413–419 CrossRef CAS PubMed.
- K. Zhong and Q. Wang, Carbohydr. Polym., 2010, 80, 19–25 CrossRef CAS PubMed.
- B. Yang, M. Zhao and Y. Jiang, Food Chem., 2009, 114, 629–633 CrossRef CAS PubMed.
- B. Yang, M. Zhao, K. N. Prasad, G. Jiang and Y. Jiang, Food Chem., 2010, 118, 364–368 CrossRef CAS PubMed.
- Y. Yi, M. W. Zhang, S. T. Liao, R. F. Zhang, Y. Y. Deng, Z. C. Wei, X. J. Tang and Y. Zhang, Carbohydr. Polym., 2012, 87, 636–643 CrossRef CAS PubMed.
- Y. Yi, M. W. Zhang, S. T. Liao, R. F. Zhang, Y. Y. Deng, Z. C. Wei and B. Yang, Carbohydr. Polym., 2012, 87, 1311–1317 CrossRef CAS PubMed.
- G. Jiang, L. Wen, F. Chen, F. Wu, S. Lin, B. Yang and Y. Jiang, Carbohydr. Polym., 2013, 92, 758–764 CrossRef CAS PubMed.
- K. Zhong, Q. Wang, Y. He and X. He, Int. J. Biol. Macromol., 2010, 47, 356–360 CrossRef CAS PubMed.
- Y. Yi, F. Huang, M. W. Zhang, R. F. Zhang, Y. Y. Deng, Z. C. Wei and J. R. He, Molecules, 2013, 18, 11601–11613 CrossRef CAS PubMed.
- Y. Yi, S. T. Liao, M. W. Zhang, R. F. Zhang, Y. Y. Deng, B. Yang and Z. C. Wei, Molecules, 2011, 16, 10324–10336 CrossRef CAS PubMed.
- Q. Zhu, Y. Jiang, S. Lin, L. Wen, D. Wu, M. Zhao, F. Chen, Y. Jia and B. Yang, Biomacromolecules, 2013, 14, 1999–2003 CrossRef CAS PubMed.
- B. Yang, M. Zhao and Y. Jiang, Food Chem., 2008, 110, 294–300 CrossRef CAS PubMed.
- Y. Pan, K. Wang, S. Huang, H. Wang, X. Mu, C. He, X. Ji, J. Zhang and F. Huang, Food Chem., 2008, 106, 1264–1270 CrossRef CAS PubMed.
- B. Yang, Y. Jiang, M. Zhao, F. Chen, R. Wang, Y. Chen and D. Zhang, Food Chem., 2009, 115, 609–614 CrossRef CAS PubMed.
- F. Danalache, P. Mata, M. Moldão-Martins and V. D. Alves, LWT--Food Sci. Technol., 2015, 62, 576–583 CrossRef CAS PubMed.
- F. Iagher, F. Reicher and J. L. M. S. Ganter, Int. J. Biol. Macromol., 2002, 31, 9–17 CrossRef CAS.
- Q. Guo, S. W. Cui, Q. Wang, H. D. Goff and A. Smith, Food Hydrocolloids, 2009, 23, 1542–1547 CrossRef CAS PubMed.
- Y. Wu, S. W. Cui, J. Wu, L. Ai, Q. Wang and J. Tang, Carbohydr. Polym., 2012, 88, 926–930 CrossRef CAS PubMed.
- Y. Tao and D. Feng, Food Hydrocolloids, 2012, 28, 151–158 CrossRef CAS PubMed.
- M. T. Nickerson, A. T. Paulson and R. A. Speers, Food Hydrocolloids, 2003, 17, 577–583 CrossRef CAS.
- M. T. Nickerson and A. T. Paulson, Carbohydr. Polym., 2004, 58, 15–24 CrossRef CAS PubMed.
- M. T. Nickerson, A. T. Paulson and F. R. Hallett, Food Res. Int., 2008, 41, 462–471 CrossRef CAS PubMed.
- Y. Yi, S. T. Liao, M. W. Zhang, J. Shi, R. F. Zhang, Y. Y. Deng and Z. C. Wei, Molecules, 2011, 16, 6148–6164 CrossRef CAS PubMed.
- L. Navarini, R. Gilli, V. Gombac, A. Abatangelo, M. Bosco and R. Toffanin, Carbohydr. Polym., 1999, 40, 71–81 CrossRef CAS.
- L.-l. Hao, D.-r. Zhang, Z.-x. Zhao and Y. Liu, Chin. J. Biochem. Pharm., 2012, 740–743 CAS.
- Z. Chen, B. Kwong Huat Tan and S. H. Chan, Int. Immunopharmacol., 2008, 8, 1663–1671 CrossRef CAS PubMed.
- C. Yang, N. He, X. Ling, M. Ye, C. Zhang, W. Shao, C. Yao, Z. Wang and Q. Li, Sep. Purif. Technol., 2008, 63, 226–230 CrossRef CAS PubMed.
- E. R. Morris, Carbohydr. Polym., 1990, 13, 85–96 CrossRef CAS.
- W. P. Cox and E. H. Merz, J. Polym. Sci., 1958, 28, 619–622 CrossRef CAS PubMed.
- X. J. Loh and O. A. Scherman, Royal Society of Chemistry, 2012 Search PubMed.
- E. R. Morris, K. Nishinari and M. Rinaudo, Food Hydrocolloids, 2012, 28, 373–411 CrossRef CAS PubMed.
- E. R. Morris, A. N. Cutler, S. B. Ross-Murphy, D. A. Rees and J. Price, Carbohydr. Polym., 1981, 1, 5–21 CrossRef CAS.
- A. M. Stephen and G. O. Phillips, Food polysaccharides and their applications, CRC Press, 2014 Search PubMed.
- M.-E. Bertrand and S. L. Turgeon, Food Hydrocolloids, 2007, 21, 159–166 CrossRef CAS PubMed.
- M. M. Ould Eleya and S. L. Turgeon, Food Hydrocolloids, 2000, 14, 245–251 CrossRef CAS.
- C. Sanchez, C. Schmitt, V. G. Babak and J. Hardy, Food Nahrung, 1997, 41, 336–343 CrossRef CAS PubMed.
- Q. Wang and K. B. Qvist, Food Res. Int., 2000, 33, 683–690 CrossRef CAS.
- C. Michon, G. Cuvelier, B. Launay and A. Parker, Carbohydr. Polym., 1996, 31, 161–169 CrossRef CAS.
- M. M. Ould Eleya and S. L. Turgeon, Food Hydrocolloids, 2000, 14, 29–40 CrossRef CAS.
- C. M. Bryant and D. J. McClements, Food Hydrocolloids, 2000, 14, 383–390 CrossRef CAS.
|
This journal is © The Royal Society of Chemistry 2015 |
Click here to see how this site uses Cookies. View our privacy policy here.