DOI:
10.1039/C5RA08796A
(Communication)
RSC Adv., 2015,
5, 54605-54612
Initial-stage oriented-attachment one-dimensional assembly of nanocrystals: fundamental insight with a collision–recrystallization model†
Received
11th May 2015
, Accepted 12th June 2015
First published on 12th June 2015
Abstract
Oriented attachment (OA) growth has been a promising method for the synthesis of one dimensional (1D) anisotropic nanocrystals (NCs). An unresolved fundamental issue is to understand the growth mechanism at the initial stage of an OA nanorod (NR) growth. In this report, a collision–recrystallization model is proposed to investigate the initial OA growth of NRs. The repulsive electrical double layer (EDL) interaction and the attractive van der Waals (vdW) interaction at the initial OA stage are derived by the accurate surface element integration (SEI) technique and the classical Hamaker equation, respectively. Our results show that the self-recrystallization of nanochains increases the collision activation energy of NPs dramatically as their surface potentials and Hamaker constants increase. Under a specific electrolyte concentration, the collision activation energy reaches the maximum, indicating that the growth rate of OA can be controlled by adjusting the electrolyte concentration.
1. Introduction
Oriented attachment (OA) has evolved to be a fundamental growth mechanism for nanocrystals (NCs).1 In OA growth, nanoparticles (NPs) in a colloidal dispersion collide and attach together along a specific crystalline orientation. OA growth has exhibited great versatility and governed the synthesis of various functional materials and structures.2–5 Compared to the traditional Ostwald ripening (OR) mechanism, OA exhibits unprecedented advantage in synthesizing anisotropic NRs of various materials.6–8 In particular, the thermodynamics and kinetics of NR growth has been investigated extensively for the rational design of synthetic parameters in an OA growth.9–15 The initial stage of an OA NR growth is of fundamental significance in the OA growth of NRs since it determines the following elongation of NRs. Several experimental observations via state-of-art in situ techniques, such as in situ high-resolution transmission electron microscopy (in situ HR-TEM) have directly revealed the details of the initial OA assembly of NRs. For instance, Li et al. observed the dynamic collision and attachment process of two iron oxyhydroxide (FeOOH) NPs to form a dimer, and the subsequent collision of a primary NP with a dimer to form a tri-mer.16 Such repeated collisions result in the formation of an N-mer (N is an integer), noted as a nanochain. Meanwhile, the collision interfaces of dimers or N-mers undergo continuous recrystallization and were completely eliminated near the completion of the OA growth. Similar process was also observed in the assembly of Pt3Fe NPs by Liao et al., showing the NR evolution from the initial nucleation in the molecular precursor solution to subsequent elongation of NRs via OA.17 Such in situ experiments demonstrate two primary processes at the initial stage of OA growth of NRs, which are collision and self-recrystallization. Collisions give rise to the elongation of NRs, while self-recrystallizations result in the elimination of collision interfaces and the smooth side-surfaces of NRs.
Although the initial processes have been explored experimentally, the driving forces associated with the collision and recrystallization at the initial stage of the anisotropic OA growth has remained rarely explored. A characteristic of this stage is the assembly between a primary NP and a dimer or a short nanochain. Modeling the initial stage of NR growth and investigating the interactions among different assembling NPs provide quantitative basis for studying the driving forces and the underlying thermodynamics and kinetics of an anisotropic OA growth. In this report, the interaction-driven collision/attachment at the initial stage of an OA nanorod growth is investigated for the first time. Two dominant inter-particle interactions in a colloidal system, the repulsive electrical double layer (EDL) and the attractive van der Waals (vdW) interaction, are evaluated quantitatively by an accurate surface element integration method (SEI) and classical Hamaker method, respectively. The effects of the major parameters on the growth of NRs at the initial stage via OA are presented, including precursor NP concentration, temperature, electrolyte concentration, surface potential of NPs and the Hamaker constant. Our work sheds new light on the rational design of synthetic parameters for anisotropic NCs with desirable physical properties.
2. Models and methodology
2.1 The collision–recrystallization model of 1D NR growth
A colloidal-self-recrystallization model is proposed to describe the initial stage of an OA NR growth. As shown in Fig. 1(a), at the beginning two spherical NPs with a radius of R0 attach through a specific crystallographic orientation to form a dimer. A third NP then approaches the dimer with a separation distance of D, and two processes occur as shown in Fig. 1(b). One process is the elongation of the dimer via the collision with a third precursor NP. The other process is the self-recrystallization of the dimer or nanochain. In the process of self-recrystallization, the grain boundary in the existing dimer formed in previous collision induces a decreased chemical potential. Therefore, the grain boundary merges gradually owing to the dissolution, diffusion and recrystallization of the exterior dimer atoms. As a result, the self-recrystallization of a dimer produces a smooth surface on the sides of the NPs to form an NR, as shown in Fig. 1(b) and (c). During this process it is assumed that two geometric components exist in the dimer, which are the spherical segment and the cylindrical part. The cylindrical part is located between two spherical segments, as shown in Fig. 1(b). As the self-crystallization proceeds, the thickness of the spherical segment (H0) decreases. H0 can therefore be used to measure the evolution of the self-crystallization. In addition, it is assumed that in the self-recrystallization process the dimer keeps a fixed volume and a constant radius R0 in the whole growth process. Based on the above assumptions, the length of the cylindrical part L0 in the growing dimer can be calculated through the geometric relation, as given in eqn (1), | 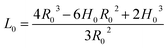 | (1) |
Notably, the length of NR L (=2L0) is equal to 8/3R0 as the self-recrystallization is completed (H0 decreases to 0). In our model, a R0 of 5 nm is used as a typical value, leading to an NR length L = 13.3 nm at H0 = 0, as illustrated in Fig. 1(d).
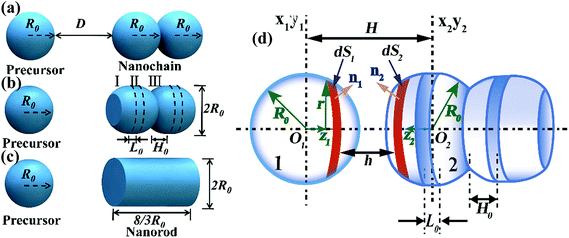 |
| Fig. 1 Configuration of a spherical precursor and a growing nanochain with three sequential growth states: (a) the initial state in which two precursors collide but the nanochain is not yet formed, and a third nanoparticle precursor approaches the nanochain; (b) the intermediate state in which the recrystallization of the nanochain occurs. The exterior atoms dissolve into solution, but diffuse and recrystallize on the interface near the collision point; (c) the final state in which the evolution of the nanochain is complete and an NR is formed; (d) a geometrical model of the intermediate state in (b) for SEI calculation of inter-particle interactions. | |
2.2 Dissolution, diffusion and recrystallization
The dissolution and recrystallization of the exterior atoms of an NP in an aqueous solution are subject to the surface energy and the surface curvature based on thermodynamics. Gibbs–Thompson relation provides the correlation between the solubility of exterior NP atoms and their relative chemical potential Δμ, as given in eqn (2),18 | 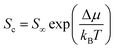 | (2) |
where Sc is the solubility of atoms on curved surfaces, S∞ represents the solubility of atoms on an infinite flat surface, kB is the Boltzmann constant and T is temperature. Δμ is the relative chemical potential of the exterior atoms on the NP, corresponding to an infinite flat surface. Δμ can be described by the Young–Laplace equation, as shown in eqn (3),18 |  | (3) |
where γ is the surface energy of the exterior atoms, Ω is the atomic volume, and R is the curvature radius. Thus, the solubility of exterior atoms can be expressed as a function of the surface energy and the curvature radius according to eqn (2) and (3). Since only the site of grain boundary has negative curvature radius, showing lower chemical potential compared with other sites, atoms from other facets, for instance, the end facets of the nanochain, dissolve and are thermodynamically favored to move to the nearby joint before the grain boundary is eliminated. For convenience, it is assumed as a hypothetical final state of recrystallization in this study that the end facets transform gradually to flat surfaces as OA growth proceeds. In fact, the recrystallization ends before H0 decreases to 0.
2.3 Theoretical calculation of inter-particle interactions
The interactions between a precursor NP and a growing nanochain of continuous self-recrystallization typically include a repulsive EDL interaction and an attractive vdW interaction.13 Accurate calculation of the two interactions is conducted with surface element integration (SEI) methods.
2.3.1 EDL interaction.
NPs submerged in an electrolyte solution absorb potential-determining ions onto the NP surface by chemical binding, and then generate a compact charging layer, noted as the Stern layer. These anchored ions continue to attract counter ions in the electrolyte by electrostatic forces and form a diffusion layer surrounding the Stern layer. As a consequence, an electrical double layer structure is established, which ensures the stability of the colloidal dispersion by providing a mutually repulsive surface charge to prevent colloidal particles from aggregating. Due to the existence of EDL around NPs, the repulsive EDL interactions between NPs during the OA growth must be taken into consideration. On the basis of the Gouy–Chapman model and the superposition approximation, the electrical double layer interaction energy per unit area between two infinite flat surfaces is given in eqn (4),19 | E(h) = 64kBTn∞κ−1ϒ02 exp(−κh) | (4) |
where n∞ is the number of indifferent solute ions per cubic meter far from the surface of NPs, h is the separation distance between two infinite flat surfaces, and κ−1 is the Debye–Hückel length. The parameter ϒ0 is defined in eqn (5), | 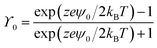 | (5) |
where ψ0 is the surface potential of the NPs which distributes uniformly on the NPs' surface, z is the valence number of the indifferent solute ions, and e is the elementary charge. κ−1 is a function of n∞ and T, as given in eqn (6),19 | 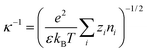 | (6) |
where ε represents the absolute permittivity of the solution. κ−1 has the unit of length and is viewed as EDL thickness.
Due to the complexity to directly solve the potential of the curved EDL from the Poisson–Boltzmann equation, Derjaguin Approximation (DA) is typically adopted to obtain the approximate solution by dividing the spherical surface into a series of circular planar faces. However, this conventional method neglects the effect of NP surface curvature and orientation giving rise to the overestimation of interactions for small NPs in dilute solution. The results is relatively accurate only if the separation between two flat surfaces is negligible compared to the size of NPs (h2/R02 ≪ 1) and the radius of NPs is 10 times larger than the EDL thickness (κR0 > 10).19,20 To overcome these limitations, we use the SEI technique, which is preferable for curved surfaces with constant potential and provides more accurate results by considering the orientation of the approximated planar faces.20 The EDL interaction between two curved surfaces based on the SEI technique is expressed in eqn (7),21
|  | (7) |
where
A1 is the
xy-plane projected area of the actual surface of particle 1,
S1, d
A1 is the
xy-plane projected area of the differential area elements on the surfaces of particle 1, d
S1.
n1 and
n2 are the unit normal vectors outward the approaching differential surface elements, respectively,
k1 and
k2 are the unit vectors parallel to
z axes in their respective coordinate system on each NP. Here
h is the separation along
z axis between d
S1 and d
S2. All these parameters are shown in
Fig. 1(f). EDL interaction is dependent on the shape of NPs according to
eqn (7). In our model, the EDL interaction between an approaching precursor NP and a growing nanochain is considered as the sum of a series of interactions among different surface pairs. The interaction of each pair can be obtained by numerical integration. The detailed theoretical derivation is provided in the ESI.
†
2.3.2 vdW interaction.
In contrast to the repulsive EDL interaction, the other dominant interaction among the NPs is the attractive van der Waals interaction. Hamaker has given the expression of the vdW interaction energy between two macroscopic bodies, as shown in eqn (8),22 | 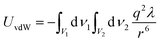 | (8) |
where dν1 and dν2 are the volume elements of macroscopic particles, V1 and V2 are the particle volumes, q represents the atom density of particles, λ is the London-van der Waals constant, and r represents the distance between dν1 and dν2. In addition, the constant π2q2λ is often substituted by A, the Hamaker constant. In this study, A is assumed to be ∼10−19 J, which is on the same order of Hamaker constants of a variety of metals and metal oxides. Similar to the calculation of EDL interactions, the total vdW interaction is divided into the interactions between separated volumes. The interaction of each pair of volumes can be calculated by numerical integration. The detailed derivation is shown in the ESI.† By taking the overall effect of two interactions into account, the total interaction energy is given in eqn (9).
EDL interaction increases exponentially while the vdW interaction increases inversely proportional to the sixth power of distance as separation distance decreases. If the overall interaction energy of the system is plotted as a function of distance between NPs, it is seen that there exists a total potential energy maximum as the spherical precursor approaches the growing nanochain as shown in Fig. 2. This maximum is defined as the activation energy Ea of OA growth since it is the energy barrier for the formation of a nanochain from NPs. Moreover, the separation between the precursor and the nanochain at Ea is defined as the critical separation dc. Based on activation energy Ea, the OA growth rate can be evaluated according to the Arrhenius equation, as given in eqn (10),23
| 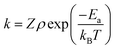 | (10) |
where
k is the rate constant of nanochain formation,
ρ is the steric factor, and
Z is the collision frequency. The lower the activation energy is, the more likely an effective collision between NPs occurs and the faster the OA growth is.
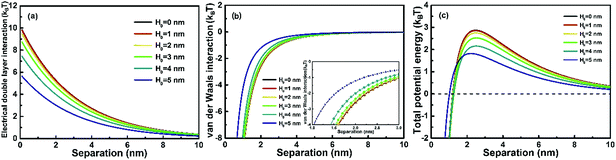 |
| Fig. 2 Plots of inter-particle (a) EDL interaction, (b) vdW interaction and (c) total interaction interactions versus surface-to-surface separation d between a spherical precursor NP and a growing nanochain. The inset in (b) shows the magnified plot in the range of D between 1.0 nm and 3.0 nm. The relative permittivity of the solution ε = 78.3, electrolyte concentration M = 0.01 mol L−1, surface potential of ψ0 = 50 mV, Hamaker constant A = 10−19 J and T = 298 K. | |
3. Results and discussion
At the initial stage of an OA NR growth, the morphology of the as-synthesized nanostructure and the growth rate are determined largely by synthetic parameters including the concentration of precursor NPs, temperature, electrolyte additives and ligands. The focus of this report is to investigate how these parameters affect the OA growth from an energetic standpoint by employing the aforementioned collision–recrystallization model.
3.1 The effect of precursor concentration
The effect of the concentration of precursor NPs on the inter-particle interaction between an NP and a self-recrystallizing nanochain with different H0 is investigated first. The concentration of precursor NPs in the solution is considered to be inversely proportional to the mean inter-particle separation distance (D), and D is then employed in the calculation instead of the concentration. The plots of EDL, vdW and total interaction versus D are shown in Fig. 2. As shown in Fig. 2(a), EDL interaction increases exponentially as D decreases regardless of H0. This means that as the concentration of precursor NPs increases, the average inter-particle repulsive EDL interaction increases. On the other hand, in a colloidal system with a specific precursor concentration where the average inter-particle separation D is fixed, EDL interaction increases as H0 decreases, indicating that a nanochain with a higher extent of self-recrystallization experiences a larger EDL repulsive interaction. Fig. 2(b) shows inter-particle vdW interactions versus D. vdW varies little once D is larger than 5 nm. However, as D is below 5 nm, the attractive vdW increases significantly as D decreases for nanochains with any H0. In addition, vdW increases as H0 decreases at fixed D. For example, the inset of Fig. 2(b) shows that the nanochain without any self-recrystallization (H0 = 5 nm) experiences smaller vdW forces with a nearby NP compared to a nanochain with a small extent of self-recrystallization (H0 = 4 nm). However, the vdW interaction varies little as H0 further decreases below 4 nm. Therefore, there exists an optimized recrystallization state of nanochains, at which the attractive vdW driving force for OA assembly reaches the maximum. The total interaction between a nanochain and an NP is shown in Fig. 2(c) by considering both repulsive EDL and attractive vdW interactions. The interaction energy maximum Ea, defined as the collision energy barrier, increases as H0 decreases, suggesting that the OA growth of nanochains experiences increased energy barrier as self-recrystallization proceeds. The critical separation distance dc, defined as the separation where the energy maximum is reached, increases as H0 decreases. These results illustrate that a lower precursor concentration is beneficial for the OA growth of nanochains with either partial or complete self-recrystallization, whereas a higher concentration of precursors is required for the fast OA growth of nanochains without self-recrystallization. It is noted that as particle size increases, only the absolute values such as collision energy barrier and critical separation vary. The main conclusions made in this study are not affected by particle size.
3.2 The effect of temperature
The effect of temperature on the self-recrystallization and collision is shown in Fig. 3. Fig. 3(a) shows Ea variation with H0 at different temperatures. Ea decreases as H0 increases, and such a decrease becomes abrupt as H0 > 3. At a fixed H0, Ea decreases as the temperature increases. The changes of Ea at different temperatures become larger as H0 is smaller than 2 nm, which indicates that the collision process is accelerated as temperature increases. This effect is more prominent at temperatures below 328 K, as evidenced by steeper curve. The critical distance versus H0 at different temperatures is plotted in Fig. 3(b). dc increases initially as H0 decreases but becomes stable at smaller H0. As temperature increases, dc increases, indicating that a more dilute precursor concentration can be used for the OA growth of 1D nanochain/NR. It should be noted that the effects of temperature on Ea and dc in the collision and self-recrystallization process at the initial OA stage arise from the different responses of EDL and vdW to the temperature change; EDL decreases significantly as temperature increases, while vdW does not change as much with temperature. According to the eqn (5), the EDL interaction is a function of temperature. Temperature affects EDL interaction via the surface charge density of the nanoparticles, σ*, and the thickness of EDL, κ−1. Eqn (11) provides an approximate correlation between the charge density on the nanoparticles and the temperature of the solution.19 | σ* = (2n∞εkBT)1/2[exp(zeψ0/2kBT) − exp(−zeψ0/2kBT)] | (11) |
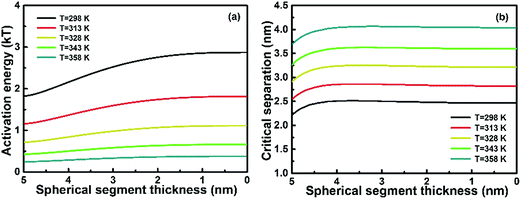 |
| Fig. 3 Plots of (a) activation energy and (b) critical separation versus the spherical segment thickness of a growing nanochain at different temperatures. The electrolyte concentration is kept at M = 0.01 mol L−1, surface potential at ψ0 = 50 mV and Hamaker constant at A = 10−19 J. | |
Moreover, the surface potential of the nanoparticles is determined by the Nernst equation as shown in eqn (12),19
| 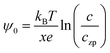 | (12) |
where
x is the valence number of the potential-determining ions M
x+,
c is the concentration of the potential-determining ions M
x+, and
czp represents a concentration point of the potential-determining ions corresponding to the nanoparticle surface with zero charge, abbreviated as pzc (point of zero charge). Hence, the nanoparticle surface is positively charged as
c is larger than pzc. Combining
eqn (11) and (12), it can be deduced that
σ* decreases as pzc increases. pzc is found to increase with increasing temperature.
24,25 Thus
σ* decreases as temperature increases. In addition, according to
eqn (6), increasing temperature compresses the EDL thickness, since the product
εT decreases with increasing temperature. Thus, the coverage of the EDL shrinks as temperature increases and the EDL interaction attenuate rapidly as the separation between two nanoparticles is larger than
κ−1. Therefore,
UEDL/
kBT decreases as temperature increases.
vdW interaction almost does not change as temperature changes according to eqn (8), since the Hamaker constant or the atom density q of nanoparticles is reduced subtly with increasing temperature. In addition, temperature cannot influence molecular instantaneous dipoles in nanoparticles, as generated by the fluctuation of electron cloud. Therefore, the influence of temperature on vdW interaction is negligible. The variation of total inter-particle interaction with temperature mainly arises from the variation of EDL interaction. In conclusion of this section, increasing temperature decreases largely the collision energy barrier regardless of self-recrystallization, increases the critical distance, and thus facilitates an increased OA growth rate, especially for a dilute precursor dispersion.
3.3 The effect of electrolyte concentration
Electrolyte concentration is also an important factor in the OA growth of NRs.26 Varying electrolyte concentration typically affects EDL interactions by varying Debye length and surface potential on the surface of NPs. Fig. 4(a) shows that at any give H0 the activation energy Ea increases as the electrolyte concentration increases until a peak is reached at ∼0.01 mol L−1. According to eqn (4), EDL interaction is a function of electrolyte concentration. σ* increases as electrolyte concentration increases according to eqn (11) and (12), and the electrostatic repulsion between precursors and nanochains thus increases considerably, leading to the elevation of Ea. However, the Debye–Hückel length κ−1 decreases with increasing electrolyte concentration according to eqn (6), which indicates that more indifferent counter ions move close to nanoparticle surfaces and screen the EDL force. Therefore, there must exist a critical concentration, which is ∼0.01 mol L−1 with the parameters employed in this study. Below the critical concentration, EDL interaction increases with increasing electrolyte concentration due to increasing surface charge. Above the critical concentration, EDL interaction decreases due to the screen effect. The critical electrolyte concentration is correlated to the temperature, surface potential and nanoparticle precursor concentration since EDL interaction is affected largely by these factors. In a synthetic experiment, to minimize the Ea of an OA growth, the critical electrolyte concentration should be avoided. Fig. 4(b) shows that dc decreases rapidly as the electrolyte concentration increases below the critical value and above the critical value, such a decrease becomes gradual. For instance, for an NP with a nanochain of H0 = 5 nm, as shown in the inset graph dc decreases from ∼3.8 nm at 0.002 mol L−1 to ∼2.2 nm at 0.01 mol L−1, but only decreases from ∼2.2 nm at 0.01 mol L−1 to 1.7 nm at 0.04 mol L−1. This suggests that increasing electrolyte concentration facilities the controlled OA growth in a media with higher concentration of precursor nanoparticles. For nanochains even with a small portion of self-recrystallization, dc increases notably, especially as the electrolyte concentration is above 0.01 mol L−1. Such results indicate that the stability of the colloidal dispersion increases as the self-crystallization proceeds in an OA growth.
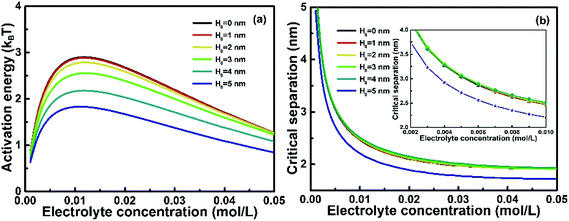 |
| Fig. 4 Plots of (a) activation energy and (b) critical separation versus electrolyte concentration of the solution at different spherical segment thicknesses H0 of a growing nanochain. The relative permittivity of solution ε = 78.3, surface potential ψ0 = 50 mV, Hamaker constant A = 10−19 J and T = 298 K. | |
3.4 The effect of NP surface potential
Surface potential of NPs in a colloidal dispersion, which is adjustable by manipulation of the dispersion pH and temperature, is another factor influencing the growth rate of OA.27,28 Unlike zeta potential, surface potential cannot be measured directly, but it can be easily determined with eqn (12). Fig. 5 shows the plots of Ea and dcversus surface potential. As shown in Fig. 5(a), Ea increases as surface potential increases, and this increase become predominant as ψ0 > 30 mV, a value believed typically to be the minimum to keep a stable colloidal dispersion.19 For nanochains of different H0, Ea increases as H0 decreases at fixed surface potential. At higher surface potentials, the increase of Ea with H0 becomes more obvious, indicating that at lower surface energy, the self-recrystallization of nanochain has less effect on the collision process compared with higher surface potentials. Fig. 5(b) shows that dc decreases as surface potential increases, and dc varies little as H0 varies. As shown in the inset graph of Fig. 5(b), only ∼0.5 nm difference in dc is found as H0 changes from 5 nm to 4 nm and dc keeps almost constant as H0 decreases further. The result indicates that the dispersion stability increases with increasing surface potential, and a higher Ea should be overcome for inter-particle collisions as the self-crystallization proceeds in an OA growth.
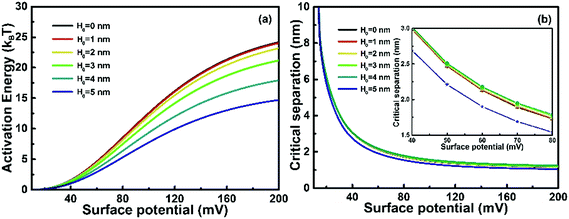 |
| Fig. 5 Plots of (a) activation energy and (b) critical separation versus surface potential at different spherical segment thicknesses of a growing nanochain. Inset in (b): the magnification graph of (b) as surface potential locates in the range of 40–80 mV. The relative permittivity of solution ε = 78.3, electrolyte concentration M = 0.01 mol L−1, Hamaker constant A = 10−19 J and T = 298 K. | |
3.5 The effect of Hamaker constant
Hamaker constant (A) is correlated directly with the inter-particle vdW according to eqn (8). The investigation of the effect of Hamaker constant on Ea and dc facilitates our understanding on the interactions of NPs of a specific material. As shown in Fig. 6, Ea decreases monotonically as A increases. At fixed A, Ea increases as H0 decreases, and such an increase becomes subtle as A increases. Fig. 6(b) shows that dc increases as A increases. At a specific A, dc for nanochains of H0 = 5 nm is ∼0.25 nm for H0 in the range of 0–4 nm, as shown in the inset of Fig. 6(b). The result indicates that nanochains with a small portion of self-recrystallizations and with a larger A experience low Ea and relatively small dc and, thus, are featured with a high rate of OA growth. In addition, solvent also influences the Hamaker constant, and thus inter-particle vdW interaction. Considering that nanoparticles interact with solvent molecules, the Hamaker constant A is typically developed into the following form as shown in eqn (13),22 | A = π2(q12λ11 + q02λ00 − 2q1q0λ01) | (13) |
where the subscripts 1 and 0 represent nanoparticle and solvent, respectively, q1 and q0 are the atomic densities of nanoparticle and solvent, respectively. λ11, λ00 and λ01 are London-van der Waals constants for the pairs of the substances noted by the subscripts. The London-van der Waals constant λ is expressed by eqn (14),22 | 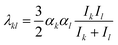 | (14) |
where αk and αl represent the dipole polarizabilities of materials k and l, respectively. Ik and Il are the first ionization energies of materials k and l, respectively. Based on eqn (13) and (14), nanoparticles prefer to attach at a high atom density plane with a strong dipole polarizability and a sufficient ionization energy in a low atom density solvent, such as ethanol solution. For metal nanoparticles such as gold nanoparticles, the Hamaker constant of which varies from 16.2 × 10−20 J to 45.5 × 10−20 J, oriented aggregation is more likely to occur compared with oxide nanoparticles of Hamaker constants from 10.5 × 10−20 J to 15.5 × 10−20 J.29
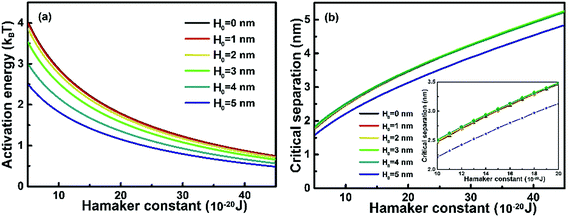 |
| Fig. 6 Plots of (a) activation energy and (b) critical separation versus Hamaker constant at different spherical segment thicknesses of a growing nanochain. Inset: the magnification of (b) in the Hamaker range of 1 × 10−19–2 × 10−19 J. The relative permittivity of solution ε = 78.3, electrolyte concentration M = 0.01 mol L−1, surface potential ψ0 = 50 mV and T = 298 K. | |
In addition, attention should also be paid to the effect of solvents. Organic solvents show typically much smaller dielectric constants than that of water. Therefore, with organic solvents the EDL thickness becomes thinner according to eqn (6), and EDL interaction becomes smaller according to eqn (4). Due to the decrease of EDL repulsive interaction, NPs trend to aggregate. For the controlled OA growth of well-defined nanostructures, organic solvents with larger viscosities should be used to suppress the Brownian motion and decrease the OA growth rate. Surfactants such as long-chain alkyl-amine/acid can also be used to induce steric effect. Despite the simplicity of the numerical models in this study to quantitatively describe the initial stage of OA growth by EDL and vdW interactions, the collision and self-recrystallization processes of NPs are influenced frequently by surface ligands, the crystallographic anisotropy, and the motion and elimination of defects such as dislocations at grain boundaries. For instance, surface ligands can induce steric force as two NPs approach. Surface polarity due to different materials and irregular-shape induced unbalanced electron density influence largely the surface charge. Charged ligands like ionic surfactants also alter the surface charge distribution of NPs and influence the EDL interaction dramatically.30–32 All these factors are not included in our numerical models. Further studies should be focused on these factors in detail from mesoscopic and atomic levels.
4. Conclusions
A collision–recrystallization model has been proposed to study the initial stage of NR formation via the OA mechanism. Based on our model, the theoretical expressions of the total interaction energy including the repulsive EDL interaction and the attractive vdW interaction are derived by the accurate SEI technique and the classical Hamaker equation, respectively. The EDL, vdW and total energy between an NP and a nanochain of continuous self-recrystallization are then calculated by investigating both the parameters of precursor NPs, including precursor concentration, precursor morphology (or the extent of self-recrystallization of a nanochain), surface potential, Hamaker constant, and the parameters of solutions, such as electrolyte concentration and temperature. The results show that the collision activation energy of NPs in the OA growth increases dramatically with the evolution of self-recrystallization, and such increase is more pronounced as NP surface potential and Hamaker constant increase. OA growth is favored in the colloidal dispersion with a high primary precursor concentration, a high temperature and a moderate electrolyte concentration. In addition, the OA growth rate can be controlled by adjusting the electrolyte concentration in the dispersion system. With a specific electrolyte concentration, the collision activation energy reaches the maximum. Our work facilitates the theoretical understanding on the role of interactions with the initial morphology evolution of nanocrystals in the OA growth.
Acknowledgements
The work is supported by UESTC new faculty startup fund, the National Natural Science Foundation (grant no. 21403031 and 11402069), the Fundamental Research Funds for the Chinese Central Universities (grant no. ZYGX2014J088) and the Shenzhen Peacock Technological Innovation Program (grant no. KQCX20140521144102503).
References
- R. L. Penn and J. F. Banfield, Science, 1998, 281, 969 CrossRef CAS PubMed.
- Q. Zhang, S. J. Liu and S. H. Yu, J. Mater. Chem., 2009, 19, 191 RSC.
- X. F. Yu, D. S. Wang, Q. Peng and Y. D. Li, Chem.–Eur. J., 2013, 19, 233 CrossRef CAS PubMed.
- C. Y. Zeng, W. X. Zhang, S. X. Ding, Z. H. Yang, H. Zeng and Z. C. Li, CrystEngComm, 2013, 15, 5127 RSC.
- H. Song, K. H. Lee, H. Jeong, S. H. Um, G. S. Han, H. S. Jung and G. Y. Jung, Nanoscale, 2013, 5, 1188 RSC.
- H. Y. Liang, H. G. Zhao, D. Rossouw, W. Z. Wang, H. X. Xu, G. A. Botton and D. L. Ma, Chem. Mater., 2012, 24, 2339 CrossRef CAS.
- M. S. Kim and Y. M. Sung, CrystEngComm, 2012, 14, 1948 RSC.
- F. Yu, X. Xu and C. J. Baddeley,
et al.
, CrystEngComm, 2014, 16, 1714–1723 RSC.
- D. Spagnoli, J. F. Banfield and S. C. Parker, J. Phys. Chem. C, 2008, 112(38), 14731–14736 CAS.
- H. Zhang and J. F. Banfield, CrystEngComm, 2014, 16(8), 1568–1578 RSC.
- M. Raju, A. C. T. van Duin and K. A. Fichthorn, Nano Lett., 2014, 14(4), 1836–1842 CrossRef CAS PubMed.
- W. He, J. Lin, B. Wang, S. Tuo, S. T. Pantelides and J. H. Dickerson, Phys. Chem. Chem. Phys., 2012, 14, 4548 RSC.
- W. Lv, W. He, X. Wang, Y. Niu, H. Cao, J. H. Dickerson and Z. Wang, Nanoscale, 2014, 6, 2531 RSC.
- W. He, J. Lin and X. Lin,
et al.
, Analyst, 2012, 137(21), 4917–4920 RSC.
- W. He and J. H. Dickerson, Appl. Phys. Lett., 2011, 98, 081914 CrossRef.
- D. Li, M. H. Nielsen, J. R. Lee, C. Frandsen, J. F. Banfield and J. J. De Yoreo, Science, 2012, 336, 1014 CrossRef CAS PubMed.
- H. G. Liao, L. Cui, S. Whitelam and H. Zheng, Science, 2012, 336, 1011–1014 CrossRef CAS PubMed.
-
G. Cao, Nanostructures and Nanomaterials: Synthesis, Properties and Applications, World Scientific, 2004, ISBN 1-86094-415-9 Search PubMed.
-
P. C. Hiemenz and R. Rajagopalan, Principles of Colloid and Surface Chemistry, revised and expanded, CRC Press, 1997, vol. 14 Search PubMed.
- S. Bhattacharjee and M. Elimelech, J. Colloid Interface Sci., 1997, 193, 273 CrossRef CAS PubMed.
- S. Bhattacharjee, M. Elimelech and M. Borkovec, Croat. Chem. Acta, 1998, 71, 883 CAS.
- H. Hamaker, Physica, 1937, 4, 1058 CrossRef CAS.
-
E. R. Leite and C. Ribeiro, Crystallization and growth of colloidal nanocrystals, Springer, 2011 Search PubMed.
- Y. Berube and P. L. de Bruyn, J. Colloid Interface Sci., 1968, 27, 305 CrossRef CAS.
- A. Lopez Valdivieso, J. Reyes Bahena, S. Song and R. Herrera Urbina, J. Colloid Interface Sci., 2006, 298, 1 CrossRef CAS PubMed.
- N. D. Burrows, C. R. H. Hale and R. L. Penn, Cryst. Growth Des., 2012, 12(10), 4787–4797 CAS.
- K. A. Dunphy Guzman, M. P. Finnegan and J. F. Banfield, Environ. Sci. Technol., 2006, 40, 7688–7693 CrossRef CAS.
- S. Yin, F. Huang, J. Zhang, J. Zheng and Z. Lin, J. Phys. Chem. C, 2011, 115(21), 10357–10364 CAS.
-
A. C. Pierre, Introduction to Sol-Gel Processing, Kluwer, Norwell, MA, 1998 Search PubMed.
- W. He, An insight into the Coulombic interaction in the dynamic growth of oriented-attachment nanorods, CrystEngComm, 2014, 16, 1439–1442 RSC.
- W. Lv, X. Yang, W. Wang, Y. Niu, Z. Liu and W. He, An Energy Investigation into 1D/2D Oriented-Attachment Assemblies of 1D Ag Nanocrystals, ChemPhysChem, 2014, 15(13), 2688–2691 CrossRef CAS PubMed.
- W. Lv, Y. Zhu, Y. Niu, W. Huo, K. Li, G. Zhu, Y. Liang, W. Wu and W. He, RSC Adv., 2015, 5, 20783–20787 RSC.
Footnotes |
† Electronic supplementary information (ESI) available. See DOI: 10.1039/c5ra08796a |
‡ These two authors contribute equally to this work. |
|
This journal is © The Royal Society of Chemistry 2015 |