DOI:
10.1039/C5RA08300A
(Paper)
RSC Adv., 2015,
5, 46935-46940
Synthesis of hollow PdRuCo nanoparticles with enhanced electrocatalytic activity
Received
5th May 2015
, Accepted 22nd May 2015
First published on 22nd May 2015
Abstract
Herein, a simple one-pot method for the synthesis of trimetallic PdRuCo hollow nanocrystals is developed. PdRuCo hollow nanocrystals with different compositions can be obtained through a wet-chemical route, just by using a sacrificial Co nanoparticle template. The prepared nanomaterials have been analyzed by transmission electron microscopy, X-ray diffraction and X-ray photoelectron spectroscopy, etc. The prepared PdRuCo nanospheres possess an alloyed nanostructure and with an average diameter of about 49 nm. The prepared nanocrystals show much better catalytic activity for ethylene glycol and glycerol electrooxidation than that of a commercial Pd/C catalyst. The reduced cost and enhanced catalytic activity of the as-prepared Pd-based materials allow them to possess great potential applications in direct fuel cells.
Introduction
A growing amount of research attention has been paid to the development of direct polyhydric alcohol fuel cells such as direct ethylene glycol (EG) and glycerol fuel cells in the past few years, due to their particular advantages such as higher boiling points, much less volatility and higher theoretical energy density than that of methanol.1–3 Moreover, alkaline fuel cells can obtain enhanced reaction kinetics and are less corrosive materials compared with their acidic counterparts.4–6 Due to the fact that electrocatalytic oxidation of EG and glycerol are indispensable half-cell reactions in direct fuel cells, consequently, tremendous efforts have been devoted to the development of various anode electrocatalysts with high activity and low cost.7–9
Pioneering studies revealed that Pt is an effective component for the polyhydric alcohol oxidation reactions (PAOR).10–12 However, their highly expensive price and limited supply severely hider their wide use. Recently, Pd-based nanomaterials have been demonstrated to be promising electrocatalysts for PAOR due to their relatively cheaper price and similar catalytic properties.7,8,13,14 Despite of this facts, it is still of great significance to further improve the Pd utilization efficiency, their catalytic activity and reduce their cost because Pd is also a precious metal with high price and scarce reserve. To achieve this goal, significant advances have been made in the synthesis of Pd-based nanomaterials through precise control over their size, shape and composition by various synthetic methods.15–17 Combined with additional element can be an effective way to improve the catalytic activity of Pd-based materials due to synergetic effect between different components.18–20 Materials such as PdAu,21,22 PdRh23 and Pt–Pd/Ru24 nanomaterials have been prepared for PAOR, and enhanced catalytic activity has been achieved. By contrast, Ru and Co with cheaper price, have been studied relatively less despite that they are more favorable to lower the cost of Pd-based nanocrystals. And as far as we know, there are still no reports about the use of trimetallic PdRuCo for PAOR, thus their catalytic activity still remains unknown. On the other hand, hollow nanoparticles can save materials, offer more surface area and higher metal utilization efficiency thus improve their catalytic activity compared with their solid counterparts.25–27 Therefore, the synthesis of trimetallic Pd-based nanocrystals (such as PdRuCo) with hollow structure is highly desired for PAOR.
In this work, by using the galvanic replacement between Co nanoparticles and noble metal precursors (Pd and Ru), we developed a simple route for the synthesis of alloyed trimetallic PdRuCo hollow nanoparticles (PdRuCo HNPs) with tunable compositions in an aqueous solution. The method is very simple and efficient. The as-prepared PdRuCo HNPs exhibit superior catalytic activity for PAOR in alkaline condition.
Experimental
Materials
CoCl2, RuCl3, PdCl2 and trisodium citrate (TSC) were obtained from Beijing Chemical Corp (China). Nafion ethanol solution (5 wt%) was obtained from Aldrich. NaBH4 and commercial Pd/C (10 wt%) were purchased from Aladdin Chemistry Co. Ltd (Shanghai, China). All chemicals used were of analytical grade and used without further purification steps. Milli-Q ultrapure water (Millipore, ≥18.2 MΩ cm) was used in the experiments.
Synthesis of PdRuCo HNPs
To synthesize PdRuCo HNPs (type I), 0.5 mL of CoCl2 (0.2 mol L−1), 1 mL of TSC (0.2 mol L−1) were mixed into 100 mL of water, and purged with N2 for more than 30 min. Then, 10 mL of aqueous NaBH4 (0.049 mol L−1) was slowly added into the above solution under stirring. After aged for 15 min, a mixture containing 600 μL of H2PdCl4 (0.0564 mol L−1) and 440 μL of RuCl3 (0.0772 mol L−1) was diluted into 10 mL of water and added dropwise to the above reaction solution and stirred for 30 min. Another two types (type II and III) of PdRuCo HNPs were also prepared just by reducing the volume of H2PdCl4 and RuCl3 to 300 and 220 μL respectively (other conditions are same to type I). The final products were washed several times with water and collected by centrifugation. The exact composition and concentration of PdRuCo were determined by inductively coupled plasma mass spectrometer (ICP-MS).
Instruments
A TECNAI G2 high-resolution transmission electron microscope (TEM) was use to obtain the TEM images, high-resolution transmission electron microscopy (HRTEM) and high-angle annular dark-field scanning (HAADF-STEM) of PdRuCo HNPs, with a voltage operating at 200 kV. The elemental mapping and energy dispersive X-ray spectrum (EDS) measurements were also made on the same TEM. The definitely compositions of PdRuCo HNPs were determined using ICP-MS (X Series 2, Thermo Scientific USA). A D8 ADVANCE (BRUKER, Germany) diffractometer using Cu-Kα radiation with a Ni filter (λ = 0.154059 nm at 30 kV and 15 mA) was used to record the XRD patterns of PdRuCo HNPs. XPS results of PdRuCo HNPs were carried out on an ESCALAB-MKII spectrometer (VG Co., United Kingdom) with Al Kα X-ray radiation as the X-ray source for excitation.
Electrocatalytic measurements
Before the electrocatalytic test, a glassy carbon electrode (GCE) was cleaned very carefully by using alumina powder to polish it, and followed by clean in water and ethanol ultrasonically. All the tests were performed with a CHI 832B electrochemical workstation (Chenhua Instruments Corp, Shanghai, China). A home-made calibrated Ag/AgCl (saturated KCl) electrode was used as the reference electrode, while a platinum wire used as the counter electrode during the measurements. For electrocatalytic oxidation tests, certain amount of commercial Pd/C or the as-prepared PdRuCo HNPs catalyst solution was deposited on the surface of the GCE using a pipette (with a volume of 10 μL) and dried by an infrared lamp, the Pd loading mass was 21.2 μg cm−2. Then, 5 μL of Nafion (0.02%) was coated on the surface of GCE and dried before electrocatalytic experiments.
Results and discussion
Structure and composition characterizations
The shape and size of the final products were firstly examined by transmission electron microscopy (TEM). As shown in Fig. 1(A and C), all the nanoparticles exhibit a sharp contrast between their shell and inner structure, indicating the products are definitely composed of hollow nanospheres. The average diameter of PdRuCo HNPs is observed to be about 49 nm (Fig. 1B). High-angle annular dark-field scanning TEM (HAADF-STEM) image (Fig. 1E) was also used to confirm the hollow structure of PdRuCo HNPs. Fig. 1D presents the high-resolution transmission electron microscopy (HRTEM) images of the PdRuCo HNPs. The lattice spacing is measured to be 0.224 nm, which is slightly lower than 0.226 nm of the pure Pd (111) plane due to the introduction of Ru and Co atoms. The HRTEM image also indicates that the hollow nanomaterials are not single-crystalline. Elemental mapping patterns of the prepared samples (Fig. 1F–I) demonstrate that all of the three elements are uniformly distributed throughout the whole hollow nanostructure, thus proving their homogeneous alloyed structure.
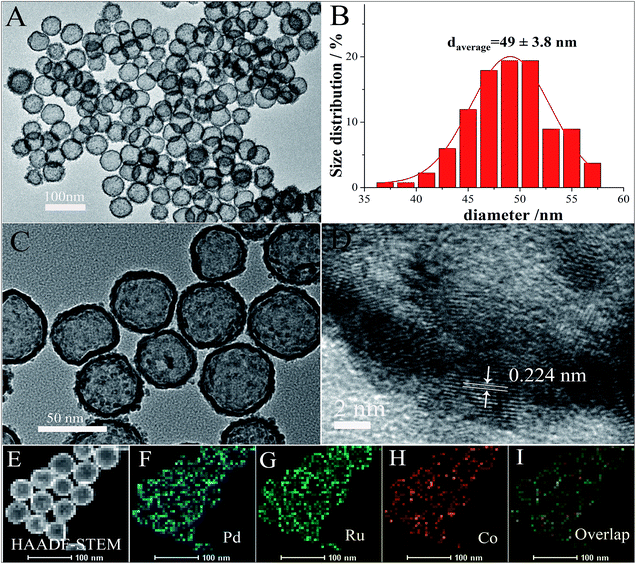 |
| Fig. 1 (A and C) Typical TEM images of the prepared PdRuCo HNPs with different magnifications. (B) Size distribution histogram, (D) HRTEM images and (E–I) HAADF-STEM and elemental mapping of PdRuCo HNPs. | |
The crystalline nature of the obtained samples was also analyzed by X-ray diffraction (XRD). As presents in Fig. 2a, the strongest diffraction peak is located at 2 theta values of 40.43°, which shifts positively of 0.36° compared with that of the pure Pd (111) plane (JCPDS no. 65-2867). Such phenomenon can also be an evidence for the formation of alloyed nanostructure. The products were further analyzed by X-ray photoelectron spectroscopy (XPS). As shown in Fig. 2b–d, the binding energy (BE) value of 335.3 eV and 340.6 eV are corresponding to the Pd 3d5/2 and 3d1/2 spectra; the peak at BE of 462.4 eV is ascribe to the presence of Ru (0), while the peaks at BE of 464.3 and 466.1 eV are attributed to the Ru (4+) species;28,29 the peaks in Co 2p3/2 signals are attributed to two cobalt species, namely, Co (0) (at BE value of 778 eV) and Co(OH)2 (2+) (at BE of 781.2 eV). XPS result also give the composition of PdRuCo HNPs as Pd63Ru13Co8. The exact compositions of the prepared PdRuCo HNPs was determined by the ICP-MS, and calculated to the mole ratios, denoted as Pd58Ru11Co7 hereafter, this result was consistent with the XPS result. The atomic ratio between Pd and Ru is higher than the molar ratio of the original precursor. This result may be due to the slow kinetics and uncompleted reduction of Ru during the reaction. For comparison, another two types of PdRuCo HNPs with different compositions were also prepared just by simply adjusting the precursors concentration (see Experiment section).
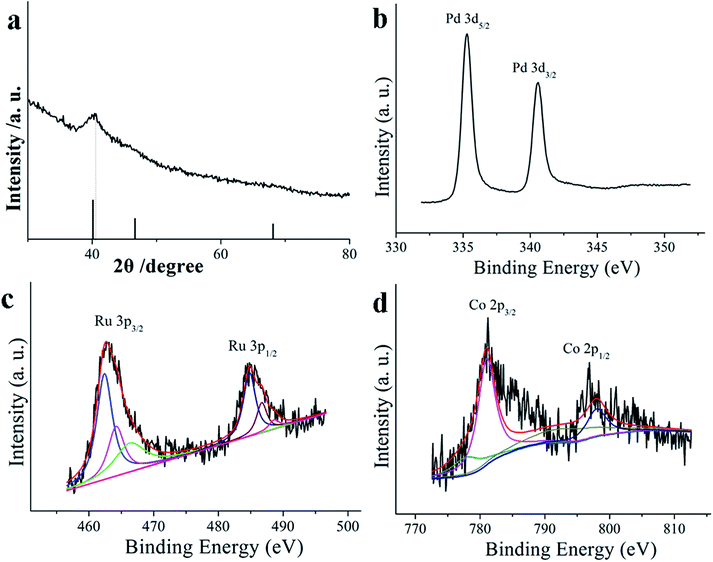 |
| Fig. 2 (a) XRD of the as-prepared PdRuCo HNPs; and XPS spectra of as-prepared PdRuCo HNPs (b) Pd 3d, (c) Ru 3p and (d) Co 2p. | |
Electrocatalytic measurements
The catalytic activity of PdRuCo HNPs for PAOR was studied with an electrochemical system, using cyclic voltammetry (CV) technique. Fig. 3A shows the CV measurement results of the prepared PdRuCo HNPs and a commercial Pd/C catalyst for ethylene glycol (EG) electrooxidation in a solution containing 0.5 mol L−1 ethylene glycol + 0.5 mol L−1 sodium hydroxide, with a scan rate of 50 mV s−1. Note: all the current densities have been normalized by the real surface area of the electrode and the loading mass of Pd similar to the reported literature.30–32 It can be noted that all the prepared PdRuCo HNPs present a higher peak current value than that of the Pd/C, especially, Pd61Ru9Co50 HNPs show the highest forward peak current of 20.7 A cm−2 mgPd−1 which is 5.9 times higher than that of Pd/C (3.5 A cm−2 mgPd−1). The forward peak current densities of the three types PdRuCo HNPs follow the order: Pd61Ru9Co50 (20.7 A cm−2 mgPd−1) > Pd58Ru11Co7 (18.3 A cm−2 mgPd−1) > Pd59Ru18Co49 (16.2 A cm−2 mgPd−1), indicating their catalytic activity is related to their compositions. To investigate the contribution of Ru in PdRuCo for the EG electrooxidation, Pd53Co8 HNPs was also prepared just without adding RuCl3 precursors. As presented in Fig. 3A, Pd53Co8 HNPs display a lower forward peak current value than that of Pd58Ru11Co7 HNPs and as well as another two types of PdRuCo HNPs. The results prove that Ru may played an important role for improving the catalytic activity for EG oxidation, because the composition of Pd53Co8 is close to the Pd58Ru11Co7, just without the existence of Ru. Moreover, all of the PdRuCo HNPs display a lower oxidation onset potential, indicating their enhanced electrooxidation kinetics.33 The tolerance of PdRuCo HNPs toward ethylene glycol electrooxidation was investigated utilizing current–time (I–t) method. As shown in Fig. 3B, all of the prepared PdRuCo HNPs present a higher residue current than that of the Pd/C during the whole 1000 second test, which demonstrates their superior electrochemical stability for ethylene glycol electrooxidation. It can also be learned from Fig. 3B, that Pd59Ru18Co49 exhibits a slower current decay compared with that of Pd61Ru9Co50 HNPs, which imply that the addition of Ru may can also improve the tolerance for the EG oxidation, since the content of Pd and Ru are very closely, but the content of Ru are different. These studies prove the superior catalytic properties of PdRuCo HNPs for the EG electrooxidation reaction.
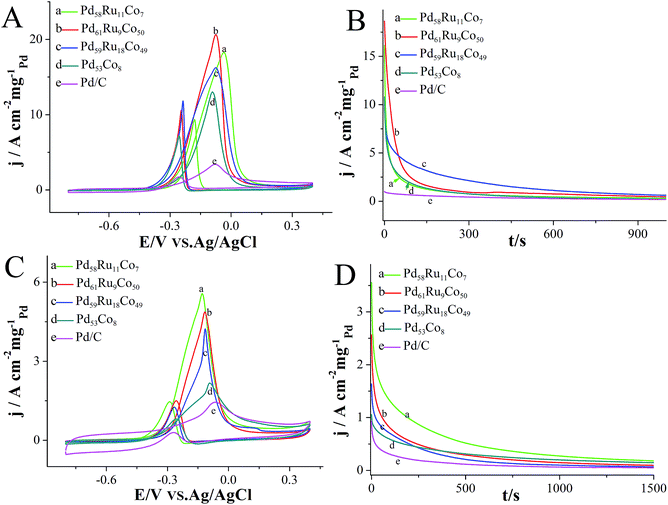 |
| Fig. 3 (A) CV and (B) I–t curves recorded at −0.1 V for ethylene glycol electrooxidation in a solution containing 0.5 mol L−1 ethylene glycol + 0.5 mol L−1 sodium hydroxide; (C) CV and (D) I–t curves recorded at −0.2 V for glycerol electrooxidation in a solution containing 0.1 mol L−1 glycerol + 1 mol L−1 potassium hydroxide. | |
Furthermore, the catalytic activity of PdRuCo HNPs toward glycerol electrooxidation was also explored similarly. The electrooxidation test was performed in a solution containing 1 mol L−1 potassium hydroxide and 0.1 mol L−1 glycerol, using CV technique, measured in the potential range from −0.8 V to 0.4 V with a scan rate of 50 mV s−1. Fig. 3C shows CV catalytic curves of the different catalysts toward glycerol electrooxidation. Different from the catalytic results of EG electrooxidation, Pd58Ru11Co7 HNPs present the highest peak oxidation current, which the forward peak current density is 5.6 A cm−2 mgPd−1, 4 times higher than that of commercial Pd/C (1.4 A cm−2 mgPd−1). The peak current in the forward scan of Pd61Ru9Co50 and Pd59Ru18Co49 is 4.8 and 4.2 A cm−2 mgPd−1, respectively. Among all of the prepared hollow nanoparticles, Pd58Ru11Co7 HNPs exhibit the lowest onset potential and peak current potential for glycerol electrooxidation, which demonstrates their superior oxidation kinetics. Based on the ICP-MS results, the mass activity (evaluated by forward peak current value) of Pd61Ru9Co50 is calculated to be 0.37 A mgPd−1 (scan rate 0.1 V s−1), the result is also better than Pd2Bi1 catalysts.34 To examine the stability of PdRuCo HNPs toward glycerol electrooxidation, I–t measurement technique was carried out at −0.2 V. As it can be learned from Fig. 3D, through the whole test time, the retained residue current density for all the PdRuCo HNPs was higher than that of Pd/C, which proving their superior tolerance for glycerol electrooxidation. All the above results demonstrate that the prepared PdRuCo HNPs hold superior catalytic activity for EG and glycerol electrooxidation. The enhanced electrocatalytic activity may be due to the following reasons. (i) The unique hollow structure of the prepared nanocrystals can provide more surface area and higher atom utilization efficiency due to their interior void space, thus contribute to the enhancement of their catalytic activity. (ii) As implied by the pioneered studies2,11,24 and the catalytic results in this work, the co-metal Ru and Co can also play an important role for enhancing the catalytic activity of the catalyst for PARO through the synergetic effect.
Conclusions
In summary, we have successfully developed a simple and efficient one-pot method to synthesize PdRuCo alloyed trimetallic nanocrystals with tunable compositions. The developed method can not only provide a new kind of catalysts with enhanced catalytic activity for ethylene glycol and glycerol electrooxidation, but also establish a successful example for the effective improvement of noble metal utilization efficiency and catalytic activity of a catalyst. Their unique nanostructure enables the prepared hollow nanoparticles may also find a wide application in catalysis and sensors, etc.
Acknowledgements
The authors acknowledge the financial support of National Natural Science Foundation of China with Grant Nos. 21190040, 91227114.
Notes and references
- A. Falase, M. Main, K. Garcia, A. Serov, C. Lau and P. Atanassov, Electrochim. Acta, 2012, 66, 295–301 CrossRef CAS PubMed.
- V. Bambagioni, C. Bianchini, A. Marchionni, J. Filippi, F. Vizza, J. Teddy, P. Serp and M. Zhiani, J. Power Sources, 2009, 190, 241–251 CrossRef CAS PubMed.
- A. Serov and C. Kwak, Appl. Catal., B, 2010, 97, 1–12 CrossRef CAS PubMed.
- C. Bianchini and P. K. Shen, Chem. Rev., 2009, 109, 4183–4206 CrossRef CAS PubMed.
- E. Antolini and E. R. Gonzalez, J. Power Sources, 2010, 195, 3431–3450 CrossRef CAS PubMed.
- E. Habibi and H. Razmi, Int. J. Hydrogen Energy, 2012, 37, 16800–16809 CrossRef CAS PubMed.
- Z.-P. Sun, X.-G. Zhang, Y.-Y. Liang and H.-L. Li, J. Power Sources, 2009, 191, 366–370 CrossRef CAS PubMed.
- M. Simões, S. Baranton and C. Coutanceau, Appl. Catal., B, 2011, 110, 40–49 CrossRef PubMed.
- L. Su, W. Jia, A. Schempf and Y. Lei, Electrochem. Commun., 2009, 11, 2199–2202 CrossRef CAS PubMed.
- P. S. Fernández, M. E. Martins and G. A. Camara, Electrochim. Acta, 2012, 66, 180–187 CrossRef PubMed.
- A. Falase, K. Garcia, C. Lau and P. Atanassov, Electrochem. Commun., 2011, 13, 1488–1491 CrossRef CAS PubMed.
- Y. Kim, H. Kim and W. B. Kim, Electrochem. Commun., 2014, 46, 36–39 CrossRef CAS PubMed.
- X. Zhang and P. K. Shen, Int. J. Hydrogen Energy, 2013, 38, 2257–2262 CrossRef CAS PubMed.
- N. Arjona, A. Palacios, A. Moreno-Zuria and M. Guerra-Balcazar, Chem. Commun., 2014, 50, 8151–8153 RSC.
- H. Zhang, M. Jin, Y. Xiong, B. Lim and Y. Xia, Acc. Chem. Res., 2013, 46, 1783–1794 CrossRef CAS PubMed.
- W. Hong, J. Wang and E. Wang, Electrochem. Commun., 2014, 40, 63–66 CrossRef CAS PubMed.
- W. Hong, J. Wang and E. Wang, ACS Appl. Mater. Interfaces, 2014, 6, 9481–9487 CAS.
- Y. Kang, X. Ye, J. Chen, Y. Cai, R. E. Diaz, R. R. Adzic, E. A. Stach and C. B. Murray, J. Am. Chem. Soc., 2013, 135, 42–45 CrossRef CAS PubMed.
- V. Mazumder, M. Chi, M. N. Mankin, Y. Liu, O. Metin, D. Sun, K. L. More and S. Sun, Nano Lett., 2012, 12, 1102–1106 CrossRef CAS PubMed.
- S. Zhang, O. Metin, D. Su and S. Sun, Angew. Chem., Int. Ed., 2013, 52, 3681–3684 CrossRef CAS PubMed.
- M. Simões, S. Baranton and C. Coutanceau, Appl. Catal., B, 2010, 93, 354–362 CrossRef PubMed.
- W. Hong, C. Shang, J. Wang and E. Wang, Electrochem. Commun., 2014, 48, 65–68 CrossRef CAS PubMed.
- R. S. Ferreira Jr, M. Janete Giz and G. A. Camara, J. Electroanal. Chem., 2013, 697, 15–20 CrossRef PubMed.
- A. Nirmala Grace and K. Pandian, Electrochem. Commun., 2006, 8, 1340–1348 CrossRef PubMed.
- X. Yu, D. Wang, Q. Peng and Y. Li, Chem. Commun., 2011, 47, 8094–8096 RSC.
- L. Wang and Y. Yamauchi, J. Am. Chem. Soc., 2013, 135, 16762–16765 CrossRef CAS PubMed.
- W. Hong, Y. Liu, J. Wang and E. Wang, J. Power Sources, 2013, 241, 751–755 CrossRef CAS PubMed.
- C. Bock, C. Paquet, M. Couillard, G. A. Botton and B. R. MacDougall, J. Am. Chem. Soc., 2004, 126, 8028–8037 CrossRef CAS PubMed.
- J. W. Guo, T. S. Zhao, J. Prabhuram, R. Chen and C. W. Wong, Electrochim. Acta, 2005, 51, 754–763 CrossRef CAS PubMed.
- H. H. Li, S. Zhao, M. Gong, C. H. Cui, D. He, H. W. Liang, L. Wu and S. H. Yu, Angew. Chem., Int. Ed., 2013, 52, 7472–7476 CrossRef CAS PubMed.
- X. J. Liu, C. H. Cui, M. Gong, H. H. Li, Y. Xue, F. J. Fan and S. H. Yu, Chem. Commun., 2013, 49, 8704–8706 RSC.
- S. Guo, S. Dong and E. Wang, Small, 2009, 5, 1869–1876 CrossRef CAS PubMed.
- C. W. Xu, H. Wang, P. K. Shen and S. P. Jiang, Adv. Mater., 2007, 19, 4256–4259 CrossRef CAS PubMed.
- A. Zalineeva, A. Serov, M. Padilla, U. Martinez, K. Artyushkova, S. Baranton, C. Coutanceau and P. B. Atanassov, J. Am. Chem. Soc., 2014, 136, 3937–3945 CrossRef CAS PubMed.
|
This journal is © The Royal Society of Chemistry 2015 |
Click here to see how this site uses Cookies. View our privacy policy here.