DOI:
10.1039/C5RA07809A
(Paper)
RSC Adv., 2015,
5, 65627-65634
Self-assembly oxygen sensing thin film based on a Ru(II) complex covalently grafted to porous anodized alumina oxide (AAO) templates†
Received
29th April 2015
, Accepted 10th July 2015
First published on 10th July 2015
Abstract
A novel functional Ru(bpy)2(phen-NH2)(PF6)2 complex (bpy = bipyridyl, phen-NH2 = 5-amine-1,10-phenanthroline) is covalently bonded to an anodized alumina oxide (AAO) template through a “soft bridge” of 3-glycidoxypropyltrimethoxysilane (GPMS). This can form an oxygen sensor with the structure [Ru(bpy)2(phen-NH)/GPMS/AAO]. This oxygen sensing thin film was characterized using scanning electron microscopy (SEM) and fluorescence spectrophotometry. A series of cluster structures were observed on the surface of the AAO, the Ru(bpy)2(phen-NH2) fluorophore which was uniformly dispersed. The film proved to have good oxygen quenching properties owing to the great interaction between the fluorophore and molecular oxygen. Finally, a Stern–Volmer plot of the [Ru(bpy)2(phen-NH)/GPMS/AAO] thin film shows a good linearity with a fitting parameter R2 of 0.9948 when the oxygen concentration is 0%–80%, and the sensitivity rises to 10.47, which is 7.5 times greater than that of oxygen sensors based on sol–gel methods. A short response/recovery time of 7 s/15 s was achieved, which is almost 100% faster than that of sol–gel methods with the same fluorophore. When stored in the ambient air of the laboratory, there was no significant drift in intensity after 6 months. These characteristics result in the thin film of [Ru(bpy)2(phen-NH)/GPMS/AAO] being a promising candidate for sensitive oxygen sensors.
Introduction
Determination of oxygen concentration is important for environmental monitoring, chemical reactions and the food industry. The typical method using a Clark-type amperometric electrode can measure oxygen concentration quicker than iodometry. However, there are several shortcomings such as analyte consumption, the requirement for a reference electrode, short service life, high cost and sensing signals which are susceptible to electromagnetic interference. Therefore, a method is needed for detecting oxygen concentration simply, quickly and accurately.
Optical oxygen sensors which perform with a quick response speed and high accuracy have been developed, and in particular optical oxygen sensors based on the fluorescence quenching principle which have unique advantages are of particular interest to researchers.1–3 The advantages of the fluorescence quenching method include high sensitivity and selectivity, short response times, non-toxicity, no O2 consumption and no requirement for a reference electrode.4–13 To date, most fluorescent transition metal complexes have been used for detecting the concentration of oxygen. Among the fluorophores used for oxygen sensors, ruthenium(II) diamine complexes have the properties of long luminescence lifetime, efficient quenching by molecular O2, short response time, large Stokes shift, and high photochemical stability, therefore they have become some of the most widely used oxygen-sensitive indicators.9,12,14–21
The previous optical oxygen sensors include immobilization of the fluorescent indicator in a matrix by physical and chemical methods. In the physical method, due to a significant polarity difference between the fluorescent indicator and the organic polymer carrier (or matrix), the fluorescent indicator is dissolved or dispersed in solid-phase support agents or plasticizer layers through electrostatic attraction, hydrogen bonding and van der Waals’ interactions. The agglomeration of fluorescent indicator often induces fluorescence quenching, drifting from the Stern–Volmer curve, internal absorption, self-extinction and poor reproducibility.22 In the chemical method, a homodisperse and efficient loading of fluorophore in the matrix can be obtained by covalently bonding fluorescent indicator with the matrix. The chemical method can effectively overcome the drawbacks of the physical method as well as demonstrating better fluorescence quenching properties, fast response speeds, high sensitivity and reproducibility.23
In this work, we present a strategy to prepare a novel oxygen sensing thin film based on covalently grafting a Ru(II) complex to a soft bridge (GPMS) via C–NH covalent bonds. On comparing with the previous sol–gel approach, the covalent grafting method overcomes the drawback of weak interactions between the matrix and fluorophore which can result in fluorophore aggregation and dye leaching when used in liquid environments. In addition, the incorporation of oxygen sensing complexes into a porous matrix has proved to be a successful method to increase their sensitivity due to the larger contact area between the fluorophore and molecular oxygen.8 An amino functionalized complex of Ru(bpy)2(phen-NH2)(PF6)2 is chosen as the fluorescent indicator in consideration of its long excited state lifetime, one-to-one correspondence between fluorescence intensity and partial pressure of oxygen, and chemical stability.24,25 AAO is chosen as the matrix because its arrays of regular microporous structures provide a greater active facet and will benefit self-assembly of the fluorophore. A novel oxygen-sensing thin film with the structure [Ru(bpy)2(phen-NH)/GPMS/AAO] is fabricated through [Ru(bpy)2(phen-NH2)] covalently bonding to AAO through a GPMS “soft bridge”, which is shown in Fig. 1.
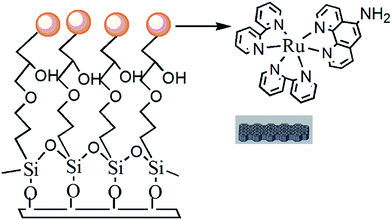 |
| Fig. 1 The structure of the [Ru(bpy)2(phen-NH)/GPMS/AAO] film. | |
Experimental section
Materials
Dipyridyl (95.0%), 1,10-phenanthroline (99.0%), anhydrous lithium chloride, ruthenium chloride hydrate (Ru > 38.0%), ammonium hexafluorophosphate (95.0%), and alumina (Al2O3, chromatographic pure and 75 μm) were purchased from Sinopharm Chemical Reagent Co. Ltd (Shanghai, China). 3-Glycidoxypropyltrimetho-xysilane (GPMS, 98%) was purchased from Sigma (USA). Anodized alumina oxide (AAO) templates (pore diameter, 200–300 nm; film diameter, 10 mm) were purchased from Puyuan Nano Technology Co. Ltd (Anhui, China). All chemical solvents were purchased from Sinopharm Chemical Reagent Co. Ltd (Shanghai, China) and were used without further purification. The ligand 5-amine-1,10-phenanthroline (phen-NH2) was synthesized and purified as described in the literature.26,27 The complex bis(2,2′-bipyridyl) ruthenium(II)chloride (Ru(bpy)2Cl2·2H2O) was synthesized and purified as described in the literature.28 Deionized water was used.
Synthesis of [Ru(bpy)2(phen-NH2)]
The synthetic procedure for [Ru(bpy)2(phen-NH2)] is shown in Scheme 1. Ru(bpy)2Cl2 (0.41 mmol) and phen-NH2 (0.41 mmol) were dissolved in ethanol (40 mL) and refluxed for 12 h at 80 °C under a nitrogen atmosphere. After the mixture was cooled to room temperature, filtered and concentrated, deionized water (10 mL) and saturated NH4PF6 solution were added to the residue. The orange-colored product precipitate was obtained in 76.3% yield via recrystallization from an EtOH/acetone (1
:
1) mixture. IR (KBr tablet, cm−1): 3454, 3388, 1612, 1593, 1522, 1468, 1409, 1179, 1079, 833, 767. 1H NMR (DMSO-d6) δ (ppm): 8.95 (d, J = 8.1 Hz, 1H), 8.88–8.83 (t, J1 = J2 = 7.5 Hz, 4H), 8.35–8.32 (d, J = 7.2 Hz, 1H), 8.21 (s, 2H), 8.13–8.10 (d, J = 8.7 Hz, 3H), 7.86 (s, 3H), 7.59 (s, 6H), 7.42–7.40 (d, J = 5.1 Hz, 2H), 7.12 (s, 1H), 6.33 (s, 2H). 13C NMR (DMSO-d6) δ (ppm): 157.16, 156.96, 152.07, 151.70, 151.46, 148.02, 146.58, 145.66, 140.87, 138.13, 138.01, 133.65, 132.95, 132.28, 128.11, 126.31, 125.15, 124.68, 123.70, 101.95. Anal. calcd for RuC32H25N7P2F12: C 42.56, H 2.91, N 10.87; found: C 42.67, H 2.87, N 10.79.
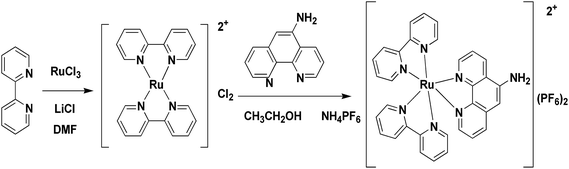 |
| Scheme 1 The synthetic route to form the [Ru(bpy)2(phen-NH2)](PF6)2 complex. | |
Self-assembly of fluorescent thin films
The procedure described in the literature with some modifications29 was used for the surface hydroxylation and epoxidation of AAO. The surface hydroxylation was carried out by soaking AAO in a mixture of H2O2 and H2SO4 (VH2O2
:
VH2SO4 = 1
:
2) for 6 h, then the substrate was washed with deionized water and dried under N2. A solution was prepared by dissolving GPMS (2.36 g) in anhydrous toluene (46 g). The aforementioned substrate was added into the solution and kept stirring at room temperature for 12 h. Finally, the substrate was washed 3 times with absolute ethanol and dried under vacuum. The active AAO with GPMS was obtained. A mixture of [Ru(bpy)2(phen-NH2)](PF6)2 (0.0009 g) and alumina was dispersed in dichloromethane (CH2Cl2, 100 mL) solution. The purpose of using alumina was to provide an acidic environment for the fluorophore [Ru(bpy)2(phen-NH2)] molecular linking to GPMS through a ring-opening reaction. After that, the reaction system was heated to 60 °C, put in preprocessed active AAO/GPMS (0.1420 g) and rotary stirred for 6 h. Then the round-bottomed flask was removed from the temperature-controlled oil bath and cooled to room temperature under ambient conditions. The substrates were then removed and placed in a Soxhlet extractor for 48 h with tetrahydrofuran (THF) and CH2Cl2 respectively in order to remove residual fluorescent complexes and free GPMS monomer. Finally, a solvent treatment can also be performed by exposing the surface to CH2Cl2 vapor for 20 min in a container. After solvent annealing, a self-assembled [Ru(bpy)2(phen-NH)/GPMS/AAO] film (0.1442 g) was formed. The amount of Ru(II) complex immobilized on the film was 32.5 mmol m−2. The fabrication of this fluorescent film is shown in Scheme 2.
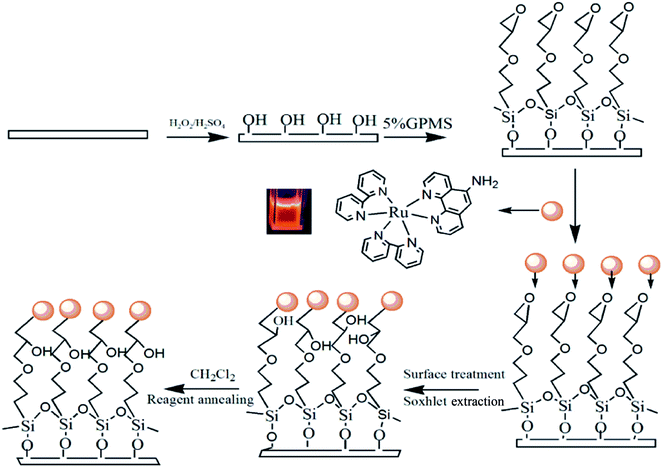 |
| Scheme 2 The fabrication process of [Ru(bpy)2(phen-NH)/GPMS/AAO] film. | |
Characterization
A HORIBA FluoroMax-4 fluorescence photometer was used for fluorescence measurements (excitation spectra, emission spectra, absolute quantum yields and excited-state lifetimes). UV-vis diffuse reflectance spectroscopy (UV-DRS) was performed with a Shimadzu UV 1700 UV-visible spectrometer. The 1H and 13C spectra were recorded on a Bruker AVANCE AV300 spectrometer in DMSO-d6 with tetramethylsilane as the internal standard. Infrared spectra (FTIR) of the complex were recorded on a Nicolet MAGNA-IR 750 spectrophotometer. The apparent morphology and energy dispersive X-ray analysis (EDX) of the [Ru(bpy)2(phen-NH)/GPMS/AAO] film were characterized and quantified with a Quanta x50 FEG scanning electron microscope (SEM). The samples were excited at 466 nm with an excitation and emission slit width of 5 nm. Oxygen and nitrogen were mixed at different concentrations using gas flow meters and stored in a mixing chamber, forming a sealed testing chamber. All measurements were performed at room temperature.
Results and discussion
FT-IR spectra of [Ru(bpy)2(phen-NH2)](PF6)2 and [Ru(bpy)2(phen-NH)/GPMS/AAO]
The FT-IR spectra of the [Ru(bpy)2(phen-NH2)](PF6)2 complex (a) and the final [Ru(bpy)2(phen-NH)/GPMS/AAO] (b) are shown in Fig. 2. It proves the successful formation of covalent grafting between the Ru(II) complex and GPMS. In Fig. 2a, the absorption bands at 1612–1409 cm−1 correspond to the C
C group of the benzene ring, while the peaks at 3454 and 3388 cm−1 originate from the NH2 group. In Fig. 2b, the formation of Si–O–Si is evident from the bands at 1079 cm−1 (νas, Si–O) and 790 cm−1 (νs, Si–O) (ν represents stretching; as, asymmetric vibrations and s, symmetric), indicating that GPMS has been successfully grafted onto AAO. An obvious observation is the change in the peak for NH2, the double peaks disappeared, exhibiting a very weak single peak in the region of 3300–3400 cm−1, which indicates that the primary amine is translated into a secondary amine, and the Ru(II) is successfully grafted onto GPMS.
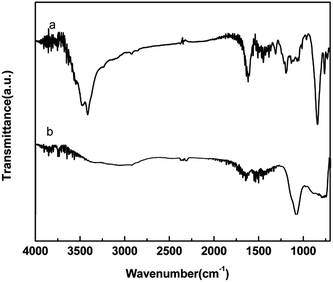 |
| Fig. 2 The FT-IR spectra of the [Ru(bpy)2(phen-NH2)](PF6)2 complex (a) and [Ru(bpy)2(phen-NH)/GPMS/AAO] (b). | |
Apparent morphology of the [Ru(bpy)2(phen-NH)/GPMS/AAO] film
After soxhlet extraction and solvent annealing, the [Ru(bpy)2(phen-NH)/GPMS/AAO] film emitted a bright orange light (shown in the inset of Fig. 3a) under ultraviolet light similar to that of [Ru(bpy)2(phen-NH2)] in solution (Scheme 2), and its morphology has been investigated through SEM (shown in Fig. 3). Fig. 3b shows a typical top view of the AAO template with an ordered honeycomb structure and a uniform pore diameter. In Fig. 3a, a series of ordered cluster structures are observed on the surface of the AAO. This reveals that, by means of a ring-opening reaction, the [Ru(bpy)2(phen-NH2)] fluorophore with an appended amino group was grafted to the terminal epoxy group of GPMS, thus an orderly thin film was self-assembled on the surface of the AAO matrix. In this film, GPMS can improve the covalent grafting efficiency and create an ordered fabrication, acting as a “soft bridge” between the fluorophore and block AAO matrix.
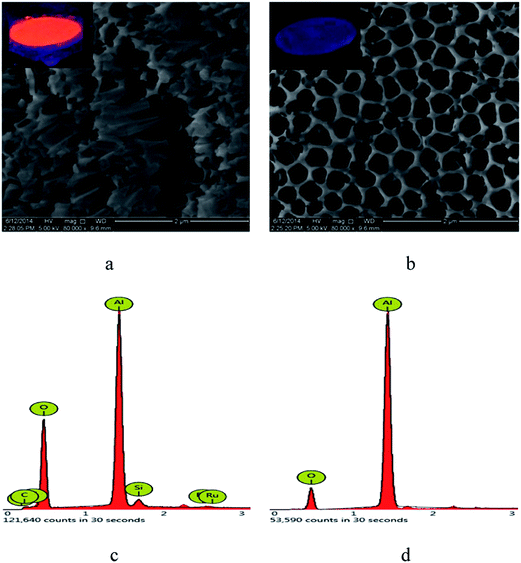 |
| Fig. 3 SEM and EDX images of the [Ru(bpy)2(phen-NH)/GPMS/AAO] film (a and c) and the AAO matrix (b and d). The insets are images of the [Ru(bpy)2(phen-NH)/GPMS/AAO] film and AAO matrix under ultraviolet light. | |
In addition, energy-dispersive X-ray spectroscopy (EDX) of AAO and the self-assembled [Ru(bpy)2(phen-NH)/GPMS/AAO] film was performed (shown in Fig. 3c and d), inferring the existence of a Ru(II) complex.
The fluorescence properties of the [Ru(bpy)2(phen-NH)/GPMS/AAO] film
The fluorescence properties of the [Ru(bpy)2(phen-NH)/GPMS/AAO] film were measured and compared to those of [Ru(bpy)2(phen-NH2)](PF6)2 in CH2Cl2 solution, which is shown in Fig. 4. The peak of the excitation spectra lies at 466 nm. There is no overlap with the emission spectra. The emission maximum wavelength of the film lies at 599 nm, close to that of [Ru(bpy)2(phen-NH2)](PF6)2 in CH2Cl2 solution, which might indicate that the Ru(II) complex has been loaded onto the surface of the AAO. The 8 nm blue shift of the emission of the film is due to the Franck–Condon excited state of the complex being unrelaxed and restricting the mobility of the fluorescent complex, which is caused by the complex being covalently grafted to AAO. Hence the emission occurs from a higher energy level than that in solution. This caused a blue shift in the emission of the Ru(II) complex when the surrounding media changed from fluid to solid.30,31
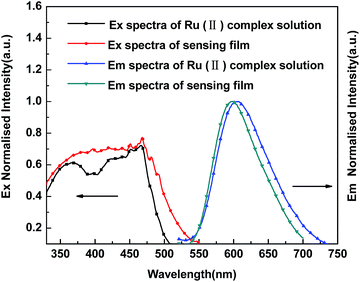 |
| Fig. 4 Excitation and emission spectra of [Ru(bpy)2(phen-NH)/GPMS/AAO] thin film and [Ru(bpy)2(phen-NH2)](PF6)2in CH2Cl2 solution. | |
The solid state fluorescence of fluorophores may encounter serious self-quenching. Therefore, the fluorescence quantum yield was measured (shown in Fig. 3S†) and the results are shown in Table 1. We found that fluorophores encounter solid state fluorescence self-quenching compared with the solution state. However, the quantum yield in the solid state is still very high, up to 9.26%, compared with oxygen sensors based on Ru(II).32,33 In most analytical situations, working with solutions of these luminophores is not practical, therefore, a film is still promising as a sensor.
Table 1 The quantum efficiency of [Ru(bpy)2(phen-NH2)](PF6)2 in CH2Cl2 solution (I) and [Ru(bpy)2(phen-NH)/GPMS/AAO] film (II)
|
λex (nm) |
λem (nm) |
Ø (%) |
I |
466 |
607 |
24.52 |
II |
466 |
599 |
9.26 |
Oxygen sensing properties
Molecular triplet oxygen is not the only quencher of the fluorophores, which we have demonstrated with various common environmental interferents. As shown in Fig. 5, when CO2, NO2, NH3, and H2 were injected into the sensor chamber, no obvious quenching was observed. However, when O2 was injected, the fluorescence intensity was quenched to 89% of the initial level, which demonstrates that a distinct quenching effect occurs upon the [Ru(bpy)2(phen-NH)/GPMS/AAO] film detecting oxygen gas, while the other gases induced much smaller fluorescence decrements. This experiment confirms the noteworthy selectivity of the [Ru(bpy)2(phen-NH)/GPMS/AAO] film for oxygen.
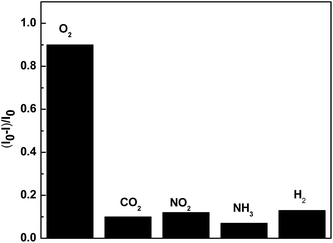 |
| Fig. 5 The relative fluorescence responses to various gases. The black bars reveal the interference effects of the gases. | |
The fluorescence emission spectra of the [Ru(bpy)2(phen-NH2)/GPMS/AAO] film under different oxygen concentrations at room temperature are shown in Fig. 6, in which the oxygen concentration varies from 0%–100% with an increment of 10%. From Fig. 6, the emission peak of the [Ru(bpy)2(phen-NH)/GPMS/AAO] film displays a board band ranging from 550 nm to 700 nm centered at 599 nm, and the fluorescence emission intensity of the [Ru(bpy)2(phen-NH)/GPMS/AAO] film displays a decreasing trend as the oxygen concentration was increased, suggesting that the emissive state of the [Ru(bpy)2(phen-NH2)] fluorophore is quenched by molecular oxygen. The relative fluorescence intensities of the [Ru(bpy)2(phen-NH2)/GPMS/AAO] film decreased by 89% upon changing the atmosphere from pure N2 to pure O2.
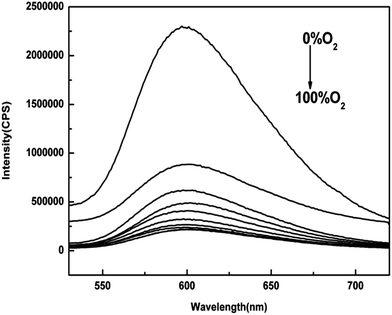 |
| Fig. 6 The fluorescence emission spectra of the [Ru(bpy)2(phen-NH)/GPMS/AAO] film under different oxygen concentrations with an interval of 10%. | |
The quenching mechanism34 is described by formulae (1) and (2), where “*” denotes an excited state and M represents [Ru(bpy)2(phen-NH)/GPMS/AAO].
|
M* + 3O2 → M(S0) + 1O2 + energy (quenching)
| (2) |
The oxygen in air is mainly triplet oxygen. When under optical excitation, fluorescent molecules change from the ground state to the excited state. 3O2 collides with the excited fluorophore absorbing the energy and causing the ground state of O2 to migrate to the excited state, forming singlet oxygen (1O2) and causing the fluorescent material to revert back to the ground state, which means that the energy of the fluorescent indicator transfers to the oxygen and creates its own luminescence quenching.
For convenience of comparison, I0/I100 is defined as oxygen sensitivity, where I0 is the emission intensity under a 100% N2 atmosphere, and I100 is that under a 100% O2 atmosphere. The sensitivity value for the [Ru(bpy)2(phen-NH)/GPMS/AAO] film is then calculated to be 10.47 (I0/I100), which is 7.5 times greater than that of oxygen sensors based on Ru(bpy)2(phen-NH2) with supporting matrices of silica molecular sieves or sol–gel. This can be attributed to the relative ordered cluster structures formed on the surface of the AAO which increases the contact ratio between the Ru(bpy)2(phen-NH2) fluorophore and molecular oxygen. There was no significant drift in intensity after the substrates were stored under the ambient air of the laboratory for 6 months.
The Stern–Volmer plot is another important measure of oxygen sensor performance. In the case of oxygen sensing, the intensity from the Stern–Volmer equation with dynamic quenching can be described by formula (3), where I is the fluorescence intensity under different oxygen concentrations, I0 is the value in the absence of the quencher, Ksv is the Stern–Volmer constant, and [O2] denotes the O2 concentration.35
It is known that a linear relation between I0/I versus [O2] indicates a good oxygen sensor. The Stern–Volmer plot for the [Ru(bpy)2(phen-NH)/GPMS/AAO] film is shown in Fig. 7 and displays a good linearity when the oxygen concentration is between 0% and 80% (shown in the inset of Fig. 7). The fitting parameters for Fig. 7 when the oxygen concentration is 0–80% are as follows: R2 = 0.9948 and KSV = 0.1193. The good linear Stern–Volmer plot for [Ru(bpy)2(phen-NH)/GPMS/AAO] should be attributed to the uniform distribution of luminophore and the direct contact between oxygen and the fluorophore. However, the fluorescence quenching of the sensor film by oxygen decreases and drifts from the Stern–Volmer curve when the oxygen concentration reaches over 80%. This might be attributed to a saturation effect between fluorescent indicators and oxygen molecules after the oxygen concentration reaches 80%.
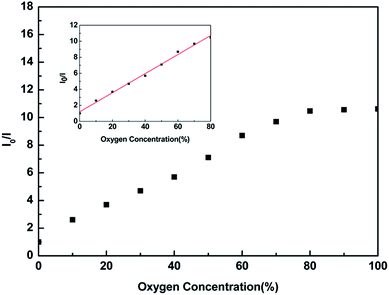 |
| Fig. 7 The Stern–Volmer plot of the [Ru(bpy)2(phen-NH)/GPMS/AAO] film. | |
To accurately evaluate the quenching mechanism of the Ru(II) complex by molecular oxygen, the excitation spectrum, absorption spectrum and emission lifetime of the Ru(II) complex in the presence of molecular oxygen were measured. There is no change in the excitation spectrum and absorption spectrum, but the emission lifetime of the Ru(II) complex decayed in the presence of molecular oxygen. This may be explained by the process of oxygen quenching through dynamic collisions between molecular (triplet) oxygen and the excited electronic state of the oxygen-sensitive probes. Dynamic (collisional) quenching by oxygen is a photophysical (rather than a photochemical) process. It is fully reversible, does not alter the optical probe, and thus has no effect on the absorption spectrum or excitation spectra. However it can lead to a reduction of the emission intensity and decay time. Fig. 8 shows the excited-state lifetimes of the [Ru(bpy)2(phen-NH)/GPMS/AAO] film, which is responsible for the Stern–Volmer plot shown in Fig. 7, measured in the presence of pure N2 (τ = 475 ns) and under an ambient atmosphere (τ = 130 ns). It is observed that the oxygen sensor exhibits a single-exponential excited-state decay process. An explanation for this is that Ru(II) complex molecules with a soft bridge (GPMS) occupy the same microenvironment sites and are distributed uniformly and homogeneously. These results are similar to those previously reported by Bright et al.10
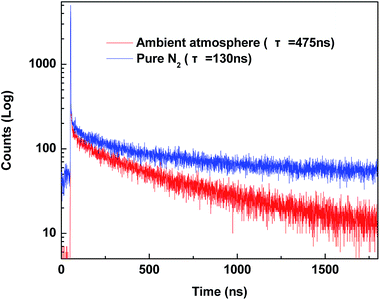 |
| Fig. 8 Typical excited-state intensity decay profile for the covalently grafted [Ru(bpy)2(phen-NH)/GPMS/AAO] in the presence of pure N2 and under ambient atmosphere. | |
For oxygen sensing materials, short response/recovery times are very important to consider for practical applications, especially for the continuous determination of oxygen concentrations. Fig. 9 shows the dynamic response of the [Ru(bpy)2(phen-NH)/GPMS/AAO] film when periodically varied from a 100% N2 to a 100% O2 atmosphere. It can be seen that when the [Ru(bpy)2(phen-NH)/GPMS/AAO] film is exposed to pure O2 the emission intensity drops rapidly, and when the atmosphere is changed to pure N2, the emission intensity recovers to its initial value. Accordingly, the [Ru(bpy)2(phen-NH)/GPMS/AAO] film exhibits a reversible emission response towards a periodically varied atmosphere. The response time (tQ) is 7 s and the recovery time (tR) is 15 s. The response speed of the [Ru(bpy)2(phen-NH)/GPMS/AAO] film is almost 100% faster than that of the sol–gel method with the same fluorophore. The shorter response time suggests that the [Ru(bpy)2(phen-NH)/GPMS/AAO] film is highly sensitive to O2 due to the direct interaction between the fluorophore and molecular oxygen, compared to oxygen quenching based on sol–gel or physical blending that always needs the diffusion and penetration process of oxygen molecules in the polymer matrix before the oxygen molecules get to the fluorophore. In addition, the recovery time is longer than the response time, which is distinctly similar to the results obtained from oxygen sensing systems using Ru(II) complexes as the probe and mesoporous molecular sieves as the supporting matrix.36
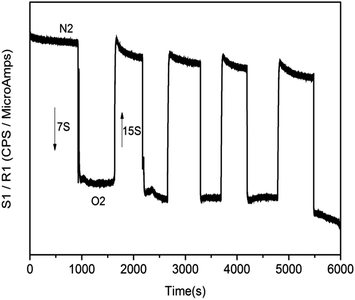 |
| Fig. 9 Response time of the [Ru(bpy)2(phen-NH)/GPMS/AAO] film when exposed to periodically varied 100% N2 and 100% O2 atmospheres. | |
Conclusions
A novel fluorescent [Ru(bpy)2(phen-NH)/GPMS/AAO] film for oxygen sensing has been self-assembled and investigated. The structure of the film with a sensitive fluorophore covalently grafted to the terminal epoxy group of GPMS on the surface of an AAO matrix can efficiently improve the self-assembly performance. It has proved to be an available strategy for molecular oxygen sensing. In addition, the short response time is attributed to the direct contact between the molecular fluorophore and molecular oxygen, compared to traditional oxygen quenching which always needs the diffusion and penetration of oxygen molecules in the polymer matrix before oxygen molecules reach the fluorophore. The high sensitivity and short response/recovery times suggest that the fluorescent film with an ordered microporous cluster structure has a satisfactory oxygen quenching performance. Therefore, this film is a promising candidate for optical oxygen sensing applications in environmental monitoring.
Acknowledgements
The authors wish to acknowledge the financial support from the Jiangsu Province Transformation of Scientific and Technological Achievements Program (BA 2014123) and the Fundamental Research Funds for the Central Universities (CXLX13_106).
References
- C. Preininger, I. Klimant and O. S. Wolfbeis, Anal. Chem., 1994, 66, 1841–1846 CrossRef CAS.
- W. Trettnak, W. Gruber, F. Reininger and I. Klimant, Sens. Actuators, B, 1995, 299, 219–224 CrossRef.
- C. Sanchez, B. Lebeau, F. Chaput and J. P. Boilot, Adv. Mater., 2003, 15, 1969 CrossRef CAS PubMed.
- L. Huynh, Z. Wang, V. Stoeva, A. Lough, I. Manners and M. A. Winnik, Chem. Mater., 2005, 17, 4765–4773 CrossRef CAS.
- M. E. Kose, A. Omar, C. A. Virgin, B. F. Carroll and K. S. Schanze, Langmuir, 2005, 21, 9110–9120 CrossRef PubMed.
- B. H. Han, I. Manners and M. A. Winnik, Chem. Mater., 2005, 17, 3160–3171 CrossRef CAS.
- M. E. Kose, B. F. Carroll and K. S. Schanze, Langmuir, 2005, 21, 9121–9129 CrossRef PubMed.
- H. Zhang, Y. Sun, K. Ye, P. Zhang and Y. Wang, J. Mater. Chem., 2005, 15, 3181–3186 RSC.
- R. M. Bukowski, R. Ciriminna, M. Pagliaro and F. V. Bright, Anal. Chem., 2005, 77, 2670–2677 CrossRef CAS PubMed.
- Y. Tang, E. C. Tehan, Z. Tao and F. V. Bright, Anal. Chem., 2003, 75, 2407–2413 CrossRef CAS.
- M. C. DeRosa, P. J. Mosher, G. P. A. Yap, K. S. Focsaneanu, R. J. Crutchley and C. E. B. Evans, Inorg. Chem., 2003, 42, 4864–4872 CrossRef CAS PubMed.
- M. T. Murtagh, M. R. Shahriari and M. Krihak, Chem. Mater., 1998, 10, 3862–3869 CrossRef CAS.
- C. McDonagh, B. D. MacCraith and A. K. McEvoy, Anal. Chem., 1998, 70, 45–50 CrossRef CAS PubMed.
- K. Matsui and F. Momose, Chem. Mater., 1997, 9, 2588–2591 CrossRef CAS.
- D. S. Tyson, J. Bialecki and F. N. Castellano, Chem. Commun., 2000, 2355–2356 RSC.
- C. McDonagh, P. Bowe, K. Mongey and B. D. MacCraith, J. Non-Cryst. Solids, 2002, 306, 138–148 CrossRef CAS.
- K. Matsui, K. Sasaki and N. Takahashi, Langmuir, 1991, 7, 2866–2868 CrossRef CAS.
- E. R. Carraway, J. N. Demas, B. A. DeGraff and J. R. Bacon, Anal. Chem., 1991, 63, 337–342 CrossRef CAS.
- P. Innocenzi, H. Kozuka and T. Yoko, J. Phys. Chem. B, 1997, 101, 2285–2291 CrossRef CAS.
- M. M. F. Choi and D. Xiao, Anal. Chim. Acta, 2000, 403, 57–65 CrossRef CAS.
- X. Chen, Z. Zhong, Z. Lia, Y. Jiang, X. Wang and K. Wong, Sens. Actuators, B, 2002, 87, 233–238 CrossRef CAS.
- X. Lu and M. A. Winnik, Chem. Mater., 2001, 13, 3449–3463 CrossRef CAS.
- H. Zhang, B. Li, B. Lei and W. Li, J. Lumin., 2008, 128, 1331–1338 CrossRef CAS PubMed.
- L. A. Sacksteder, J. N. Demas and B. A. DeGraff, Anal. Chem., 1993, 65, 3480–3483 CrossRef CAS.
- P. Hartmann, M. J. P. Leiner and M. E. Lippitsch, Anal. Chem., 1995, 67, 88–93 CrossRef CAS.
- M. G. S. Cidalia and G. Thorfinnur, Supramol. Chem., 2009, 21, 173–180 CrossRef PubMed.
- S. M. Ji, H. M. Guo, X. L. Yuan, X. H. Li, H. D. Ding, P. Gao, C. X. Zhao, W. T. Wu, W. H. Wu and J. Z. Zhao, Org. Lett., 2010, 12, 2876–2879 CrossRef CAS PubMed.
- G. Sprintschnik, H. W. Sprintschnik, P. P. Kirsch and D. G. Whitten, J. Am. Chem. Soc., 1977, 99, 4947–4954 CrossRef CAS.
- H. Zhang, B. Li, B. Lei, W. Li and S. Lu, Sens. Actuators, B, 2007, 123, 508–515 CrossRef CAS PubMed.
- J. Ding, B. Li, H. Zhang, B. Lei and W. Li, Mater. Lett., 2007, 61, 3374 CrossRef CAS PubMed.
- M. Wrighton and D. L. Morse, J. Am. Chem. Soc., 1974, 96, 998–1003 CrossRef CAS.
- F. J. Mingoarranz, M. C. Moreno-Bondi, D. Garcia-Fresnadillo, C. de Dios and G. Orellana, Mikrochim. Acta, 1995, 121, 107 CrossRef CAS.
- I. Klimant, P. Belser and O. S. Wolfbeis, Talanta, 1994, 41, 985–991 CrossRef CAS.
- X. Wu, L. Song, B. Li and Y. Liu, J. Lumin., 2010, 130, 374–379 CrossRef CAS PubMed.
- M. T. Murtagh, M. R. Shahriari and M. Krihak, Chem. Mater., 1998, 10, 3862–3869 CrossRef CAS.
- J. N. Demas, B. A. DeGraff and W. Xu, Anal. Chem., 1995, 67, 1377–1380 CrossRef CAS.
Footnote |
† Electronic supplementary information (ESI) available. See DOI: 10.1039/c5ra07809a |
|
This journal is © The Royal Society of Chemistry 2015 |
Click here to see how this site uses Cookies. View our privacy policy here.