DOI:
10.1039/C5RA07593A
(Paper)
RSC Adv., 2015,
5, 63697-63704
Performance of hierarchical HZSM-5 zeolites prepared by NaOH treatments in the aromatization of glycerol
Received
26th April 2015
, Accepted 14th July 2015
First published on 14th July 2015
Abstract
A series of hierarchical HZSM-5 zeolites were prepared by post-synthesis modification of conventional bulk crystals of HZSM-5 zeolite with sodium hydroxide (NaOH) solution at different concentrations. These micro–mesoporous composite molecular sieves were characterized by powder X-ray diffraction, transmission electron microscopy, nitrogen adsorption, Fourier transform infrared (FT-IR) spectroscopy techniques and pyridine FT-IR to investigate the changes in crystallinity, acidity, morphology and textural property of HZSM-5 zeolite before and after alkaline treatment. The catalytic performances of these hierarchical HZSM-5 zeolites were evaluated by the aromatization of glycerol with methanol as the solvent, which was a promising route for converting renewable glycerol and methanol into high value aromatics. Substantial mesoporosity with sizes centered at around 4 nm could be generated for HZSM-5 zeolites after treatment with mild NaOH solution (≤0.4 M), coupled with improved retained microporosity, resulting in great improvements in catalytic lifetime and selectivity to benzene, toluene and xylene (BTX) aromatics during the reaction of glycerol to aromatics. Although the larger mesopore surface areas were achieved when treated with NaOH concentrations that were higher than 0.5 M, the HZSM-5 structure was partly damaged, leading to the reduction of catalytic lifetime and selectivity to BTX aromatics. The HZSM-5 treated with 0.3 M NaOH solution was found to be the optimum catalyst for the transformation of glycerol/methanol to aromatics, producing a nearly two-fold increase in BTX aromatics (carbon yields of 25.18%) and three-fold improvement in catalyst lifetime (12.5 h) when compared to the parent microporous HZSM-5 (13.9% carbon yields of BTX aromatics and catalyst lifetime of 4 h). These improved catalytic performances are mainly attributed to the optimized bimodal micro–mesoporous HZSM-5 zeolite during the alkali treatment, which retained sufficient micropores that have the capacity for aromatization and also more mesopores were created that would shorten the average diffusion path lengths, increase the accessibility to the acid sites and facilitate the transportation of large molecules, e.g., glycerol and carbon precursors, during the aromatization of glycerol.
1. Introduction
Benzene, toluene and xylene (BTX), which are known as basic organic chemical materials, are widely used in the chemical, pharmaceutical, agriculture, dyestuffs and other industries. Generally, BTX aromatics are mainly produced by tar pyrolysis and catalytic reforming of crude oil. Nevertheless, the proven storage of crude oil is expected to be sustainable for only a few decades at the present rate of consumption. Furthermore, the combustion products of crude oil have caused serious environmental pollution at the local and global level, resulting in irreversible effects on climate and life. As a consequence, it is necessary to find a sustainable and environmentally friendly process for the production of BTX aromatics.
In recent years, bio-energy, because of its characteristics such as abundance in raw materials, low price, eco-friendliness and carbon balance as well as renewability, is of growing interest in harnessing energy from renewable sources while decreasing the dependence on fossil fuels. In this situation, the biodiesel industry has achieved rapid development, giving rise to the excessive supply of glycerol as the main by-product in the biodiesel production process and making glycerol a cheap raw material in the chemical market. Therefore, extensive studies on the conversion of glycerol to more valuable chemicals have been carried out for the last few years.1–5 However, most of the studies have focussed on the reaction of glycerol hydrogenation and dehydration, and few studies have been made on the transformation of glycerol to aromatics. The catalytic transformation of glycerol into higher value BTX aromatics could not only solve the severe problems of the energy crisis but could also promote the development of the downstream of biodiesel industry.
Hoang et al.6 investigated the conversion of glycerol to alkyl-aromatics using a series of zeolites (HZSM-5, HY, Mordenite and HZSM-22). They found that the HZSM-5 zeolite showed a higher alkyl-aromatic yield compared to other zeolites. Thus, it appears that the ZSM-5 zeolite plays a significant role in the aromatization reaction of glycerol because of its shape, selectivity, and high acidity. Jang et al.7 conducted the aromatization of glycerol over HZSM-5 with water as the solvent, but the total aromatics yield was low and catalyst deactivation was fast. Luo and McDonald8 studied the aromatization of a glycerol/methanol mixture using HZSM-5 zeolite at 500 °C with 40% glycerol in methanol, at which the BTX yield and catalyst lifetime were about 10% and 3 h, respectively. The catalysts employed in the previously mentioned studies were all microporous HZSM-5 zeolites, which were generally deactivated because of pore blocking by the coke formed during the aromatization reaction.9 Glycerol has a molecular size of around 5 Å, which is similar to the apertures of the ZSM-5 channels (about 6 Å),10 which may plug the pores more readily, thus causing a fast deactivation of ZSM-5. Mesopores, which are much larger than micropores, can promote the catalytic efficiency during the aromatization of glycerol because the diffusivity of the glycerol molecules could be effectively improved with the larger mesopores. Furthermore, the mesopores could prevent the reaction intermediates, including precursors of carbon deposition, gathering in the pores during the reactions.11,12 However, excessive mesopores in the catalyst could lead to the decrease of aromatic yield because of the fact that mesopores do not have the same characteristics as micropores, such as the pore size closing to the dynamic diameter of benzene and good hydrothermal stability, which could promote aromatization reactions efficiently. Therefore, hierarchical zeolites, which offer a way to effectively combine the intrinsic characteristics of micropores with the diffusion properties of mesopores have been proposed as a promising catalyst in aromatization reactions.13–16
Desilication by alkali treatment has been proven to be an effective and economical method to introduce additional mesopores into different types of zeolites.17–20 Li et al.9 investigated the effects of desilication of ZSM-5 zeolite on its catalytic properties in catalytic fast pyrolysis of lignocellulosic biomass and discovered that the creation of mesopores in the desilicated ZSM-5 zeolites could improve the diffusion property of ZSM-5 zeolites, enhancing the conversion of lignin derived bulky oxygenates and producing more aromatic hydrocarbons. H. Mochizuki et al.21 studied the catalytic performance of alkali treated ZSM-5 in the hexane cracking reaction. The results showed that the deactivation rate of alkali treated ZSM-5 was slower than that of the parent ZSM-5 catalyst, which could be attributed to the larger number of pore entrances and shorter diffusion path lengths after the introduction of mesopores. Therefore, it was hypothesized that desilication of the ZSM-5 zeolite by alkaline treatment may provide a simple and effective way to improve the yield of BTX and prolong the catalyst life in the aromatization of glycerol.
In the present study, a series of hierarchical ZSM-5 zeolites were prepared by desilication of the conventional bulky crystals of ZSM-5 zeolite with NaOH solution at different concentrations. The catalytic performance of the prepared ZSM-5 zeolites was tested in the aromatization of glycerol. Because of the high viscosity of glycerol and the destructive effect of water on zeolites, methanol was chosen to be the solvent.6 Furthermore, a series of characterizations were performed to clarify the physicochemical properties of the desilicated ZSM-5 zeolites and associate the change of those properties with the performance of glycerol aromatization.
2. Experimental
2.1. Parent zeolites and treatments
A commercial HZSM-5 zeolite with a silicon/aluminium (Si/Al) ratio of 25 (from Nanjing Refinery Co., Ltd) was primarily used in this study. The hierarchical HZSM-5 samples were prepared by a simple alkali treatment method.22 In a typical run, 10 g of calcined zeolite was added into 200 mL of sodium hydroxide (NaOH) solution of varying concentrations (0.1 M, 0.2 M, 0.3 M, 0.4 M, 0.5 M and 0.7 M) under stirring at 348 K for 2 h. Subsequently, the resulting slurry was rapidly cooled down to room temperature, then filtered, washed with distilled water until a neutral pH was reached, and finally dried at 373 K overnight. The samples obtained were converted to the H-form using ion-exchange in 1.0 M ammonium nitrate solution at 353 K for 8 h, followed by drying at 373 K for 12 h and calcination at 823 K for 4 h. For the following analysis, the parent and alkali treated zeolites are referred to as ZSM-5-P and AT-nM, respectively, where the n denotes the concentration of NaOH solution used in the alkaline treatment, for example, AT-0.1M.
2.2. Catalyst characterizations
Powder X-ray diffraction (XRD) patterns of the parent and desilicated ZSM-5 zeolites were recorded on a Bruker D8 Focus powder X-ray diffractometer using CuKα radiation (λ = 1.5406 Å) with a step of 0.02° at a voltage of 40 kV and a current of 40 mA.
The surface areas and pore characteristics of the samples were measured using a Beishide 3H-2000 nitrogen (N2) absorption instrument. Before N2 adsorption at 77 K, the zeolites were degassed under vacuum at 573 K for 12 h. The specific areas of the zeolites were determined by applying the Brunauer–Emmett–Teller (BET) method. Microporous volumes were calculated using the t-plot method. Pore size was obtained from the analysis of the desorption branches of N2 isotherms using the Barrett–Joyner–Halenda (BJH) method.
The morphologies of the samples were examined using transmission electron microscopy (TEM) using a Jeol JEM-2000EX instrument with an accelerating voltage of 120 kV.
The Fourier transform infrared (FT-IR) spectroscopy spectra of the zeolites were recorded with a Nicolet 5700 spectrometer.
The acidities of the samples were identified using pyridine FT-IR (Py-IR) using a Nicolet 6700 spectrometer. All the samples were pressed into wafers (10 mm diameter). Then the wafers were placed into the measurement cell at 773 K under vacuum for 2 h. After the temperature of the cell was lowered to 423 K, the samples began to adsorb the pyridine vapour and this absorption is continued for 30 min and then the vapour is evacuated for 1 h before recording the spectra.
The amount of coke formed during the aromatization was determined using thermogravimetric analysis (TGA). About 8 mg of the samples was measured over the temperature range of 323–1073 K at a heating rate of 20 K min−1 under an oxygen atmosphere. The carbon deposition rate was calculated as follows:
2.3. Catalyst testing
The conversion of glycerol into BTX aromatics was carried out in a fixed-bed, stainless steel reactor (inner diameter = 8 mm). The catalyst (1.3 g, 40–60 mesh) was packed in the middle zone of the reactor. A thermocouple was inserted into the middle of the reactor to measure the temperature of the catalyst bed during the reaction. The mixture of 40% glycerol in methanol (Sinopharm Chemical Reagent Co., Ltd., purity > 99.5%) was fed by a syringe pump at a weight hourly space velocity (WHSV) of 0.96 h−1 into the reactor through a preheater maintained at a temperature of 563 K. The reaction was performed at 673 K and atmospheric pressure. The liquid products were collected in a cold trap and analysed using a Shanghai Techcomp Ltd., GC7890 gas chromatograph equipped with a flame ionization detector and a capillary column (SE-30). Chlorobenzene was used as the internal standard. The yield of aromatics was calculated from the number of carbon atoms. During the aromatization process, the conversion rates of both glycerol and methanol were nearly close to 100% (≥99%), thus, the selectivity of product is approximately equivalent to the carbon yield. The following computational formulas were used in this research:
where: X is the conversion rate of raw materials, Y is the carbon yield and S is the selectivity.
3. Results and discussion
3.1. Effects of alkali treatment on the physicochemical properties of HZSM-5 zeolite
3.1.1. XRD results. The XRD patterns of the parent and alkali treated HZSM-5 zeolites are shown in Fig. 1. It was observed that all of the catalysts showed the characteristic diffraction peaks of an MFI type zeolite. Although the intensities of the peaks were very slightly decreased with an increase of NaOH concentration, the characteristic diffraction peaks of the MFI zeolite were still preserved even for AT-0.4M, indicating that the structure of HZSM-5 was not damaged even when treated with 0.4 M NaOH solution. However, a higher concentration of NaOH caused a dramatic decrease in the intensity of the peaks, indicating a noticeable loss of crystallinity because of the partial dissolution of zeolite grains, (e.g., AT-0.5M), and even the severe collapse of the zeolitic structure, (e.g., AT-0.7M). These results were similar to the previous observations found in other reports.9,17
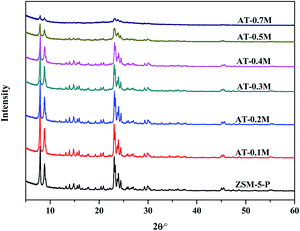 |
| Fig. 1 XRD patterns of parent (ZSM-5-P) and alkali treated ZSM-5 (AT-nM) zeolites. | |
3.1.2. Surface area and pore volume. Fig. 2 shows the N2 adsorption–desorption isotherms and the corresponding pore size distribution results of the parent and alkali treated HZSM-5 zeolites. As illustrated in Fig. 2A, the parent HZSM-5 zeolite presented a typical Type I isotherm, which is characteristic of a microporous nature. The alkali treated HZSM-5 zeolites showed a combination of Type I and Type IV isotherms, with the appearance of micropore filling at low pressures and hysteresis loops at higher pressures, indicating the formation of hierarchical porous system combining microporosity and mesoporosity.23 In addition, by increasing the concentration of NaOH solution used in the alkaline treatment, the hysteresis loop became more distinct, indicating the growing number of mesopores. The pore size distributions of all HZSM-5 samples are presented in Fig. 2B. The mesopore sizes for all the hierarchical samples are mainly centered at around 4 nm, and the more the concentration of the alkaline solution was increased, the more visible 4 nm mesopores became. Such phenomena were also observed by Ogura et al.24 and Song et al.25 As shown in Table 1, after alkali treatment, the mesopore volumes and external surface areas of the zeolites increased significantly, whereas the micropore volumes of the HZSM-5 zeolites decreased, which can be attributed to the transformation of some micropores to mesopores. It was supported by the results shown in Fig. 2 and Table 1 that more mesoporosity was generated gradually with the increase of the dosage of NaOH solution, and these results were consistent with the visual inspections from the TEM observation (Fig. 3). However, when the parent HZSM-5 was treated with a lower concentration of NaOH solution, the formation of mesoporosity on the surface of HZSM-5 hardly led to substantial decreases in the microporous surface areas and volumes. This showed that the microporosities were mostly retained in these samples, which was expected to have a positive effect on the catalytic activity for the conversion of glycerol. Nevertheless, the treatment with higher concentrated (0.5 M and 0.7 M) NaOH would destroy the micropore system more severely.
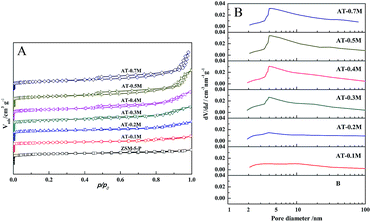 |
| Fig. 2 The N2 adsorption and desorption isotherm (A) and the corresponding pore size distribution results (B) of the parent and alkali treated HZSM-5 zeolites. | |
Table 1 Physical properties of parent and alkali treated HZSM-5 zeolites
Sample |
SBETa (m2 g−1) |
Smicrob (m2 g−1) |
Sextb (m2 g−1) |
Vtotal (mL g−1) |
Vmicrob (mL g−1) |
Vmesoc (mL g−1) |
From N2 adsorption measurements (BET method). From N2 adsorption measurements (t-plot). From N2 adsorption measurements (BJH method). |
ZSM-5-P |
412.5 |
350.6 |
61.9 |
0.242 |
0.146 |
0.115 |
AT-0.1M |
409.9 |
340.2 |
69.7 |
0.294 |
0.141 |
0.162 |
AT-0.2M |
401.6 |
324.2 |
77.4 |
0.323 |
0.132 |
0.192 |
AT-0.3M |
429.9 |
297.9 |
132.0 |
0.414 |
0.123 |
0.331 |
AT-0.4M |
393.4 |
245.8 |
147.6 |
0.476 |
0.102 |
0.419 |
AT-0.5M |
423.9 |
261.0 |
162.9 |
0.640 |
0.107 |
0.578 |
AT-0.7M |
364.6 |
207.0 |
157.7 |
0.664 |
0.086 |
0.632 |
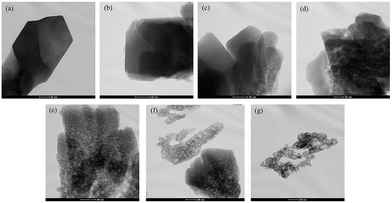 |
| Fig. 3 Transmission electron micrographs of parent and alkali treated HZSM-5 zeolites: (a) ZSM-5-P, (b) AT-0.1M, (c) AT-0.2M, (d) AT-0.3M, (e) AT-0.4M, (f) AT-0.5M, (g) AT-0.7M. | |
3.1.3. TEM studies. The TEM images (Fig. 3) provide some direct information about the influence of the NaOH treatment. As the TEM images show, after being treated with 0.1 M NaOH, the surface of the parent zeolite remains smooth. When the concentration of NaOH solution was above 0.1 M, the external surface of the alkali treated HZSM-5 zeolite (Fig. 3c) became partially corroded, and this was accompanied with many visible etched holes and pores, suggesting that the alkaline treatment had caused the development of mesoporosity. The observed characteristics are similar to previous observations by other researchers.18,21,26 The increase of the NaOH solution concentration appears to enhance the corrosion effect and results in more mesopores (Fig. 3c–e). However, the morphologies of the zeolite were severely damaged after treatment with 0.5 M and 0.7 M NaOH solution. Furthermore, several portions of the framework had collapsed and big void defects with dimensions exceeding 50 nm were formed, implying that the pore system of the zeolite was seriously destroyed.
3.1.4. FT-IR studies. The FT-IR spectra of the zeolite samples are illustrated in Fig. 4. The bands at 1221 cm−1 and 795 cm−1 (external T–O–T asymmetric stretching and symmetric stretching, respectively), 1095 cm−1 (internal T–O–T asymmetric stretching), 545 cm−1 (the vibration absorption peak of a five membered oxygen ring in the skeleton) and 453 cm−1 (T–O–T bending vibration) (T stands for Si or Al) are presented for all alkali treated samples and these spectra imply the persistence of the framework of the HZSM-5 zeolite.27 In addition, a broad band at 3448 cm−1 and on at 1637 cm−1 were assigned to the stretching and bending vibration of an hydroxyl group of physically absorbed water on the surface of the HZSM-5 zeolite.
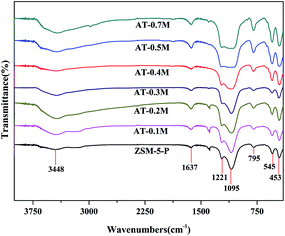 |
| Fig. 4 The FT-IR spectra of parent and alkali treated HZSM-5 zeolites. | |
However, compared to the bands obtained for the parent HZSM-5, the band at 1095 cm−1 of the alkali treated samples is less resolved because of the increase of the band at 1221 cm−1 with the increasing concentration of NaOH solution, implying that there are changes of internal T–O–T vibration and external T–O–T vibration in the zeolite frameworks. This shift could be ascribed to the selective extraction of framework Si atoms in the alkaline medium.
3.1.5. Acidity studies. The acidities of all the samples were identified by Py-IR spectra and the results are shown in Table 2. The amount of Brönsted acid (B) on the zeolites decreased gradually with an increase in the NaOH concentration, whereas the amount of Lewis acid (L) increased. Thus, the alkaline treatment of the HZSM-5 zeolite resulted in the transformation of a Brönsted acid to a Lewis acid, which may be attributed not only to the removal of internal Si–OH bonds resulting from desilication, but also to the reprecipitation of extracted Al on the external surface of the HZSM-5 zeolite.20,28 Generally, Brönsted acid sites and Lewis acid sites showed a synergistic effect during the aromatization process19 and as a result, these changes of HZSM-5 acidity originating from alkaline treatment would affect the performance of converting glycerol to aromatics, which will be discussed later in Section 3.2.
Table 2 The acidities of parent and alkali treated HZSM-5 zeolites
Samples |
Aciditya (mmol g−1) |
B acid |
L acid |
B/L |
Measured by Py-IR. |
ZSM-5-P |
0.173 |
0.271 |
0.638 |
AT-0.1M |
0.157 |
0.281 |
0.559 |
AT-0.2M |
0.132 |
0.287 |
0.460 |
AT-0.3M |
0.109 |
0.290 |
0.376 |
AT-0.4M |
0.076 |
0.313 |
0.243 |
AT-0.5M |
0.063 |
0.332 |
0.190 |
AT-0.7M |
0.049 |
0.341 |
0.144 |
3.2. Catalytic activity and stability of desilicated zeolites in glycerol aromatization
The catalytic performances of the parent and desilicated HZSM-5 zeolites were measured in glycerol aromatization reaction with methanol as the solvent and the results obtained are given in Fig. 5 and 6. The conversion rates of glycerol and methanol were always close to 100% (≥99%) along the reaction time. There were two types of products obtained during the aromatization process: (i) liquid products and (ii) gaseous products. When the aromatization reaction had been carried out for 2 h over the parent HZSM-5 zeolite under the conditions of 673 K and atmospheric pressure, the yield of total gas and liquid phase were 45 wt% and 55 wt%, respectively, and these results are in agreement with those found in the literature results.8 Fig. 5 is a representative chromatogram of liquid products collected between 1 and 2 h at 673 K from the aromatization of 40% glycerol in methanol over the parent HZSM-5 zeolite. It can be seen from Fig. 5 that before deactivation of the HZSM-5 zeolite, the main liquid products are aromatics, meanwhile, only trace amounts of oxygenates could be detected in the liquid products. And in these liquid products, besides BTX aromatics which were the target products, various heavier compounds, including trimethylbenzene, 1,2-hydrindene, diisopropylbenzene, α-tetralone, methylnaphthalene, 2-isopropylnaphthalene, and biphenyl, could be found during the process of converting glycerol to aromatics (Fig. 5). Nevertheless, the composition of liquid products changed with the reaction time favoring heavier compounds, thus leading to the deactivation of the catalyst. But after deactivation of the HZSM-5 catalyst, there were almost no aromatic products generated, while oxygenate products (such as acrolein or acetol) became the main ingredients in the liquid products. The results indicated that the deactivated catalysts were only active for the dehydration of glycerol and the active sites of the catalyst which are beneficial for aromatization were lost because of the deactivation resulting in the inability to convert these dehydration products to aromatics. The products of desilicated HZSM-5 zeolites were generally the same as those obtained with the parent zeolite, but the contents of some compounds differed greatly. Generally, under a mild alkali treatment, (e.g., using 0.1 M, 0.2 M and 0.3 M NaOH solution), the content of the gas-phase products gradually decreased, whereas the content of the liquid-phase products (mainly aromatics) increased (see Fig. 6A), indicating that the desilicated HZSM-5 zeolites obtained using an appropriate alkaline treatment were favorable for the formation of liquid products, which can be attributed to the change in the acidity and pore structure of the HZSM-5 after alkali treatment.
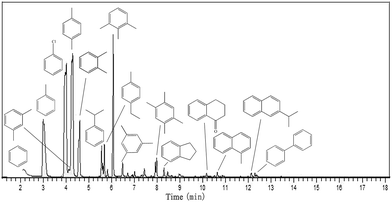 |
| Fig. 5 Total ion chromatogram of liquid products collected between 1 and 2 h in the aromatization of 40% glycerol in methanol at 673 K over the parent HZSM-5 zeolites. The WHSV is 0.96 h−1. Chlorobenzene is used as the internal standard. | |
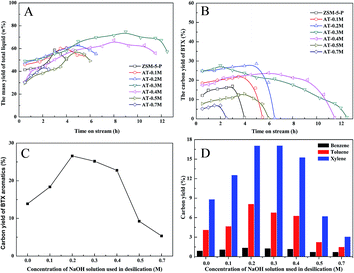 |
| Fig. 6 (A) The mass yield of total liquid products versus the reaction time. (B) The carbon yield of total BTX aromatics versus the reaction time. (C and D) The total carbon yield of BTX aromatics collected between 1 and 2 h versus the alkali concentration in the aromatization of 40% glycerol in methanol at 673 K over the parent zeolite and the desilicated HZSM-5 zeolite. The WHSV is 0.96 h−1. | |
As illustrated in Fig. 6B and C, the catalytic performance of HZSM-5 in glycerol aromatization was intensely affected by the severity of the alkaline treatment. The BTX aromatics yield with the parent HZSM-5 sample decreased rapidly with reaction time and it exhibited a lower BTX yield in the reaction of glycerol aromatization compared to the alkali treated HZSM-5 zeolites when treated with a NaOH solution concentration of not higher than 0.4 M. The BTX yield increased from 13.89% with the parent ZSM-5-P to 18.36% with AT-0.1M, 26.59% with AT-0.2M and increased even more to 25.18% with AT-0.3M, and to 22.76% with AT-0.4M. Meanwhile, the catalyst lifetime increased from 4 h for the parent ZSM-5-P to 5.5 h for AT-0.1M, to 6.5 h for AT-0.2M, to 12.5 h for AT-0.3M, and to 11.5 h for AT-0.4M. The same effect was observed in results reported in other papers.28–31 As shown in Fig. 6D, compared to the parent HZSM-5 catalyst, for BTX aromatics, the selectivity of xylene was the highest, toluene was second highest, and the selectivity of benzene was the lowest. The alkali treatment did not particularly change the benzene selectivity of the parent catalyst, whereas the selectivity of toluene and xylene both had significant changes with NaOH dosage. Also, the appropriate alkaline treatment improved the yield of the liquid-phase and reduced the yield of the gas-phase, indicating that more gaseous products were transferred into aromatics in the reaction over the desilicated HZSM-5 zeolites (see Fig. 6A). In combination with the results of sample characterization, the increase in BTX selectivity and catalytic stability for hierarchical HZSM-5 catalysts could be related to the formation of mesopores on the HZSM-5 surface, whereas there was no severe decrease of micropores after mild alkaline treatment. Furthermore, the improved B/L ratio was also the reason for the increased yield of aromatics. Aromatization of glycerol is a complex reaction, which involves dehydration, aldol condensation, cracking, oligomerization, cyclization, dehydrogenation, aromatization, H-transfer, alkylation and other reactions. The reaction process is closely related to the amount and distribution of both Brönsted acid sites and Lewis acid sites. The Lewis acid sites are beneficial to dehydration and oligomerization, while the Brönsted acid sites are advantageous to the cyclization, cracking and H-transfer reactions. Therefore, only a suitable B/L ratio can maximize the efficiency of the HZSM-5 zeolite. However, excessive Brönsted acid sites can cause the formation of heavy aromatics, leading to carbon deposition and deactivation of the zeolites. After an appropriate alkaline treatment, an appropriate B/L ratio is obtained for HZSM-5 which improves the generation of BTX aromatics, while reducing the carbon deposition. Furthermore, the hierarchical meso- and microporous system in HZSM-5 zeolites obtained by the mild alkali treatment provides shorter diffusion paths, increases the accessibility of the active sites and facilitates the diffusion of the reaction intermediates including the carbon precursors, and thus the yield of aromatics is increased and the unwanted side reactions, (e.g., polymerization of monoaromatics) are suppressed, giving hierarchical zeolites a higher activity and a longer lifetime.
In comparison, further stronger alkali treatments, (e.g., using 0.5 M and 0.7 M NaOH solution) caused a worse catalytic performance than the parent HZSM-5 catalyst, despite the much larger mesoporosity that was obtained for alkali treated HZSM-5 catalysts (Table 1). Therefore, the catalyst lifetime decreased back to 6 h for AT-0.5M and 2.5 h for AT-0.7M. It can be seen from Table 1 and Fig. 3 that the decrease of BTX yield and catalytic stability resulted from the modified HZSM-5 catalysts via a harsh NaOH treatment did not retain the microporosity and Brönsted acid sites which were beneficial for aromatization reaction very well, leading to a lower yield of liquid products, a lower BTX yield and a shorter lifetime for the catalysts.
From the previous results, the 0.3 M NaOH solution was found to be the optimal NaOH concentration for the HZSM-5 zeolite when it was used as a catalyst for the aromatization of glycerol and methanol mixtures.
3.3. Carbon deposit characterization and regeneration
TGA measurements of the spent catalysts after the aromatization reaction are shown in Fig. 7. On the whole, the weight losses were concentrated in two temperature ranges: 50–40 °C and 400–750 °C; the former was mostly attributed to the removal of water or other low boiling impurities and the latter was mainly caused by the oxidation of carbon deposited on the zeolites. According to the data obtained from the curves, the amount and rate of carbon deposition were calculated (see Table 3). As shown in Table 3, although the amount of carbon deposited on the alkali treated HZSM-5 zeolites was larger than that on the parent HZSM-5 zeolite, the lifetime of the catalysts increased and the carbon deposition rate obviously decreased after alkaline treatment over mild NaOH concentrations (0.1 M–0.4 M). This can be explained by taking into account the increase in Sext with well preserved microporosity during the alkali treatment, as indicated by the BET results (Table 1) and the TEM images (Fig. 3). With the development of mesopores, the carbon formed during the aromatization reaction tended to be deposited on the easily accessible external surfaces,13,33 effectively retarding the blocking up of the micropores, where the aromatization reaction occurs.34–36 However, with a further increase in the severity of the alkaline treatment using 0.5 M and 0.7 M NaOH, the microporosity was seriously destroyed (Fig. 3), thus, the assembled carbonaceous intermediates were readily transformed to carbon, giving rise to the reduction of the catalyst lifetime and increasing the carbon deposition rate over the treated zeolites. These results implied that the formation of mesopores with the persistence of microporosity in the zeolite structure after carefully controlling the severity of the alkaline treatment can effectively restrain the carbon deposition and prolong the lifetime of the catalyst.
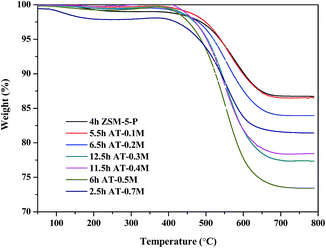 |
| Fig. 7 TGA curves for parent and alkali treated HZSM-5 zeolites. | |
Table 3 The lifetime and carbon deposition rates of parent and alkali treated HZSM-5 zeolitesa
Sample |
ZSM-5-P |
AT-0.1M |
AT-0.2M |
AT-0.3M |
AT-0.4M |
AT-0.5M |
AT-0.7M |
t: the lifetime of catalyst; wc: the amount of carbon deposition; rc: the rate of carbon deposition. |
t/h |
4 |
5.5 |
6.5 |
12.5 |
11.5 |
6 |
2.5 |
wc/% |
12.14 |
13.15 |
15.38 |
22.16 |
21.60 |
26.01 |
16.43 |
rc/% h−1 |
3.035 |
2.391 |
2.366 |
1.773 |
1.878 |
4.335 |
6.571 |
To evaluate the reusability of the catalysts, the spent catalysts were regenerated at 823 K in air for 6 h to remove the carbon deposits. Then the regenerated catalysts were reused in the aromatization of glycerol at the same conditions as used previously. The conversion rates of glycerol and methanol were still close to 100% and the composition of the products was basically the same as those shown in Fig. 5. The carbon yields of BTX aromatics certain decreased throughout two cycles of regeneration for both the parent and alkali treated zeolites (see Fig. 8). The previous results indicated that regeneration of the catalysts led to the decrease of the selectivity to BTX aromatics, which could be attributed to the destruction of the HZSM-5 structure during regeneration.32
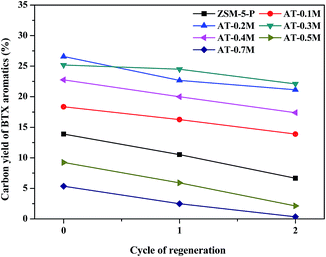 |
| Fig. 8 The carbon yields of BTX aromatics obtained by the aromatization of 40% glycerol in methanol at 673 K with regenerated HZSM-5 zeolites. | |
4. Conclusions
Hierarchical HZSM-5 zeolites combining micro- and meso-porosity were successfully prepared using a post-synthesis modification of conventional microporous HZSM-5 zeolites in NaOH solutions of different concentrations. The pore structure (microporosities and mesoporosities) can be tuned by using different amounts of NaOH. Very narrow mesopore size distributions with mesopore sizes centered at around 4 nm were mostly formed for all the alkali treated zeolites, and were observed in the N2 adsorption/desorption isotherms. The TEM images showed that the alkaline treatment induced defects in the zeolite framework and caused the generation of mesopores in the HZSM-5 zeolite. A greater degree of mesoporosity was generated gradually with the increase of the dosage of NaOH solution. XRD results showed that there was no obvious change in crystallinity of HZSM-5 zeolite when the NaOH concentration was no more than 0.4 M, but the higher concentrations of NaOH caused a noticeable loss of crystallinity and even the severe collapse of the zeolitic structure. In addition, the alkaline treatment led to the transformation of a Brönsted acid to a Lewis acid. The higher the concentration was, the more the Brönsted acid was transformed.
The catalytic performances of these hierarchical HZSM-5 zeolites were evaluated using the aromatization of glycerol with methanol as the solvent, with microporous HZSM-5 zeolite used as a reference. The results indicated that the HZSM-5 zeolite treated in mild alkali solution could improve the yields of BTX aromatics and catalyst lifetimes in the catalytic transformation of glycerol and methanol to aromatics, which could be attributed not only to the introduction of mesopores into the HZSM-5 crystals while minimizing the loss of micropores where the aromatization reaction occurs, but also to the appropriate B/L ratio, which made it easier for the conversion of reactive intermediates to aromatics. The newly formed mesopores reduced the diffusional limitations of large molecules, (e.g., glycerol and carbon precursors) and shortened the diffusion path, to some extent, improving the yields of BTX aromatics and carbon tolerance. However, severe alkali treatment of the HZSM-5 zeolite in a higher concentration of NaOH solution, (e.g., 0.5 M and 0.7 M) disturbed the crystal structure of the parent zeolite and reduced the selectivity to BTX aromatics and catalyst lifetime during the aromatization of glycerol. The modified hierarchical HZSM-5 catalyst with 0.3 M NaOH as alkaline source gave optimum results for the aromatization of 40% glycerol in methanol, which gave a nearly two-fold increase in BTX aromatics (carbon yields of 25.18%) and three-fold improvement in the catalyst lifetime (12.5 h) compared to the parent microporous HZSM-5 (13.9% BTX aromatics and 4 h for the catalyst lifetime).
Acknowledgements
The authors would like to thank the financial support from National Natural Science Foundation of China (No. 21406034, 21276050 and 21076044).
Notes and references
- V. Balaga, S. Amirineni, K. Vanama Pavan and C. V. R. Komandur, J. Nanosci. Nanotechnol., 2015, 15, 5391–5402 CrossRef PubMed
. - L. Niu, R. P. Wei, F. Jiang, M. H. Zhou, C. Y. Liu and G. M. Xiao, React. Kinet., Mech. Catal., 2014, 113, 543–556 CrossRef CAS
. - F. Kirby, A. E. Nieuwelink, B. W. M. Kuipers, A. Kaiser, P. C. A. Bruijnincx and B. M. Weckhuysen, Chem.–Eur. J., 2015, 21, 5101–5109 CrossRef CAS PubMed
. - F. S. H. Simanjuntak, J. S. Choi, G. Lee, H. J. Lee, S. D. Lee, M. Cheong, H. S. Kim and H. Lee, Appl. Catal., B, 2015, 165, 642–650 CrossRef CAS PubMed
. - M. Watanabe, T. Iida, Y. Aizawa, T. M. Aida and H. Inomata, Bioresour. Technol., 2007, 98, 1285–1290 CrossRef CAS PubMed
. - T. Q. Hoang, X. Zhu, T. Danuthai, L. L. Lobban, D. E. Resasco and R. G. Mallinson, Energy Fuels, 2010, 24, 3804–3809 CrossRef CAS
. - H. S. Jang, K. Bae, M. Shin, S. M. Kim, C. U. Kim and Y. W. Suh, Fuel, 2014, 134, 439–447 CrossRef CAS PubMed
. - G. Luo and A. G. McDonald, Energy Fuels, 2014, 28, 600–606 CrossRef CAS
. - J. Li, X. Y. Li, G. Q. Zhou, W. Wang, C. W. Wang, S. Komarneni and Y. J. Wang, Appl. Catal., A, 2014, 470, 115–122 CrossRef CAS PubMed
. - H. Schulz, Catal. Today, 2010, 154, 183–194 CrossRef CAS PubMed
. - Y. K. Park, B. R. Jun, S. H. Park, J. K. Jeon, S. H. Lee, S. S. Kim and K. E. Jeong, J. Nanosci. Nanotechnol., 2014, 14, 5120–5123 CrossRef CAS PubMed
. - S. S. Kim, B. R. Jun, S. H. Park, J. K. Jeon, D. J. Suh, T. W. Kim and Y. K. Park, J. Nanosci. Nanotechnol., 2014, 14, 2925–2930 CrossRef CAS PubMed
. - Y. M. Ni, A. M. Sun, X. L. Wu, G. L. Hai, J. L. Hu, L. Tao and G. X. Li, J. Nat. Gas Chem., 2011, 20, 237–242 CrossRef CAS
. - Q. J. Yu, Y. Li, X. J. Meng, Q. K. Cui and C. Y. Li, Mater. Lett., 2014, 124, 204–207 CrossRef CAS PubMed
. - Q. J. Yu, C. Y. Cui, Q. Zhang, J. Chen, Y. Li, J. P. Sun, C. Y. Li, Q. K. Cui, C. H. Yang and H. H. Shan, J. Energy Chem., 2013, 22, 761–768 CrossRef CAS
. - Y. L. Wang, W. M. Zhao, Z. Li, H. Wang, J. H. Wu, M. Li, Z. P. Hu, Y. S. Wang, J. Huang and Y. P. Zhao, J. Porous Mater., 2015, 22, 339–345 CrossRef CAS
. - K. Sasowska, K. Góra-Marek, M. Drozdek, P. Kuśtrowski, J. Datka, J. M. Triguero and F. Rey, Microporous Mesoporous Mater., 2013, 168, 195–205 CrossRef PubMed
. - K. Sasowska, A. Wach, Z. Olejniczak, P. Kuśtrowski and J. Datka, Microporous Mesoporous Mater., 2013, 167, 82–88 CrossRef PubMed
. - X. L. Zhu, L. L. Lobban, R. G. Mallinson and D. E. Resasco, J. Catal., 2010, 271, 88–98 CrossRef CAS PubMed
. - C. Femandez, L. Stan, J. P. Gilson, K. Thomas, A. Vicente, A. Bonilla and J. Perez-Ramirez, Chem.–Eur. J., 2010, 16, 6224–6233 CrossRef PubMed
. - H. Mochizuki, T. Yokoi, H. Imai, S. Namba and J. N. Kondo, Appl. Catal., A, 2012, 449, 188–197 CrossRef CAS PubMed
. - J. Kang, K. Cheng, L. Zhang, Q. Zhang, J. Ding, W. Hua, Y. Lou, Q. Zhai and Y. Wang, Angew. Chem., Int. Ed., 2011, 50, 5306–5309 CrossRef PubMed
. - J. C. Groen, L. A. A. Peffer, J. A. Moulijn and J. Perez-Ramirez, Colloids Surf., A, 2004, 241, 53–58 CrossRef CAS PubMed
. - M. Ogura, S. Y. Shinomiya, J. Tateno, Y. Nara, M. Nomura, E. Kikuchi and M. Matsukata, Appl. Catal., A, 2001, 219, 33–43 CrossRef CAS
. - Y. Q. Song, Y. L. Feng, F. Liu, C. L. Kang, X. L. Zhou, L. Y. Xu and G. X. Yu, J. Mol. Catal. A: Chem., 2009, 310, 130–137 CrossRef CAS PubMed
. - S. Abelló, A. Bonilla and J. Pérez-Ramírez, Appl. Catal., A, 2009, 364, 191–198 CrossRef PubMed
. - R. M. Mohamed, H. M. Aly, M. F. EI-Shahat and I. A. Ibrahim, Microporous Mesoporous Mater., 2005, 79, 7–12 CrossRef CAS PubMed
. - M. Bjorgen, F. Joensen, M. S. Holm, U. Olsbye, K. P. Lillerud and S. Svelle, Appl. Catal., A, 2008, 345, 43–50 CrossRef PubMed
. - C. S. Mei, P. Y. Wen, Z. C. Liu, H. X. Liu, Y. D. Wang, W. M. Yang, Z. K. Xie, W. M. Hua and Z. Gao, J. Catal., 2008, 258, 243–249 CrossRef CAS PubMed
. - J. Perez-Ramirez, S. Abello, A. Bonilla and J. C. Groen, Adv. Funct. Mater., 2009, 19, 164–172 CrossRef CAS PubMed
. - D. Ohayon, R. L. Mao, D. Ciaravino, H. Hazel, A. Cochennec and N. Rolland, Appl. Catal., A, 2001, 217, 241–251 CrossRef CAS
. - S. Kim, E. Sasmaz and J. Lauterbach, Appl. Catal., B, 2015, 168–169, 212–219 CrossRef CAS PubMed
. - J. Kim, M. Choi and R. Ryoo, J. Catal., 2010, 269, 219–228 CrossRef CAS PubMed
. - Y. Q. Yu, X. Y. Li, L. Su, Y. Zhang, Y. J. Wang and H. Z. Zhang, Appl. Catal., A, 2012, 447, 115–123 CrossRef PubMed
. - J. Jae, G. A. Tompsett, A. J. Foster, K. D. Hammond, S. M. Auerbach, R. F. Lobo and G. W. Huber, J. Catal., 2011, 279, 257–268 CrossRef CAS PubMed
. - G. Fogassy, N. Thegarid, Y. Schuurman and C. Mirodatos, Energy Environ. Sci., 2011, 4, 5068–5076 CAS
.
|
This journal is © The Royal Society of Chemistry 2015 |
Click here to see how this site uses Cookies. View our privacy policy here.