DOI:
10.1039/C5RA07547E
(Paper)
RSC Adv., 2015,
5, 56583-56589
Layer-by-layer self-assembly of 2D graphene nanosheets, 3D copper oxide nanoflowers and 0D gold nanoparticles for ultrasensitive electrochemical detection of alpha fetoprotein†
Received
25th April 2015
, Accepted 23rd June 2015
First published on 23rd June 2015
Abstract
In this work, a novel and ultrasensitive label-free electrochemical immunosensor was developed for the quantitative detection of alpha fetoprotein (AFP). Thanks to the simple and versatile layer-by-layer self-assembly technology, the facile and firm immobilization of 2D graphene nanosheets (GS), 3D copper oxide nanoflowers (CuO NFs) and 0D gold nanoparticles (Au NPs) was achieved in the fabrication of the proposed immunosensor. A synergic effect amongst the electrocatalytic activity, conductivity and biocompatibility of GS, CuO NFs and Au NPs could provide high sensitivity for the label-free electrochemical immunosensor. The detection mechanism was based on monitoring of the electrocatalytic current response change towards the reduction of hydrogen peroxide (H2O2) when an immunoreaction occurred on the surface of the GS–CuO NFs–Au NPs modified electrode. Under optimal conditions, the designed immunosensor exhibited a wide linear range from 10−5 ng mL−1 to 102 ng mL−1 with a low detection limit of 5.3 fg mL−1 for AFP. It also displayed an electrochemical performance with good reproducibility, selectivity and stability, which could provide potential application in the clinical diagnosis of other tumor markers.
1. Introduction
The fast, accurate and sensitive detection of tumor markers is required for diagnosis and management of cancers.1,2 In recent years, taking advantage of their easy-to-operate, economical, sensitive, and portable properties,3–5 electrochemical immunosensors have obtained considerable attention for the detection of tumor markers. Owing to remarkable achievements in nanotechnology and nanoscience, electrochemical signal amplification strategies based on nanomaterials have great potential in the improvement of the sensitivity of electrochemical immunosensors.6–9 It is well known that multifunctional nanomaterials can produce a synergic effect among electrocatalytic activity, conductivity and biocompatibility to accelerate the signal transduction, leading to highly sensitive biosensing.10–12 Therefore, the effective immobilization of multifunctional nanomaterials plays a significant role in the fabrication of electrochemical immunosensors, especially for the label-free electrochemical immunosensors.13
In our previous study, different immobilization methods of multifunctional nanomaterials have been applied in the fabrication of label-free electrochemical immunosensors. Magnetic electrode-based label-free electrochemical impedance spectroscopy immunosensor has been established for the quantitative detection of cluster of differentiation 146 antigen.14 The effective immobilization of magnetic nanomaterials through the magnetic force of magnetic glassy carbon electrode played a significant role in improving the stability and sensitivity of the proposed immunosensor. A simple electrodeposition method has also been employed to obtain a bimetallic alloy for the fabrication of another label-free electrochemical immunosensor.15 The production and amplification of electrochemical signal was achieved simultaneously in the process of quantitative detection of carbohydrate antigen 724. Both magnetic electrode method and electrodeposition method have a satisfying electrochemical performance. However, these methods can only be employed to the immobilization of some nanomaterials with special properties such as magnetism and reducibility, rather than all nanomaterials.
Layer-by-layer self-assembly becomes an effective approach for the fabrication of multifunctional nanomaterials layers based on the alternating assembly of oppositely charged layers, due to its simplicity and versatility.16 That almost any nanomaterials can be layered in controllable thickness and in desired order renders this approach eminently suitable for the fabrication of immunosensors.17,18 Poly(diallyldimethylammonium chloride) (PDDA) has been widely used as interlinkers for fabricating multilayer via the layer-by-layer self-assembly technique.19 PDDA can effectively wrap around uncharged nanomaterials, or adsorb onto negatively charged nanomaterials via electrostatic interaction.20,21 Therefore, the layer-by-layer self-assembly method and PDDA were employed for the immobilization of multifunctional nanomaterials in the fabrication of a label-free electrochemical immunosensor in this work. The uncharged 2D graphene nanosheets (GS) and 3D copper oxide nanoflowers (CuO NFs) were modified on the surface of electrode by effectively wrapped around with PDDA. And the negatively charged 0D gold nanoparticles (Au NPs) were adsorbed onto electrode surface with PDDA via electrostatic interaction. The layer-by-layer self-assembly of GS–CuO NFs–Au NPs was employed to achieve the signal amplification strategy of the designed immunosensor.
First, CuO has a good electrocatalytic performance towards the reduction of hydrogen peroxide (H2O2).22 The special 3D flower-like nanostructure of it can also increase the contact area reacted with H2O2. Second, the 2D lamellar folds nanostructure of GS with fast electron transportation, high specific surface area and good biocompatibility can accelerate the electron transfer of the electrocatalytic current response.23 Third, the 0D Au NPs can be effectively loaded on the surface of GS and CuO NFs for the capture of antibodies.24 Finally, there will be a synergic effect among electrocatalytic activity, conductivity and biocompatibility of GS, CuO NFs and Au NPs, leading to the high sensitivity of the label-free electrochemical immunosensor. Using alpha fetoprotein (AFP) as the target analyte, the electrochemical detection can be achieved when antibody–antigen immunocomplex hinders the direct electron transfer in the electrocatalytic process of GS–CuO NFs–Au NPs towards the reduction of H2O2.
2. Materials and methods
2.1. Apparatus and reagents
All electrochemical measurements were performed on a CHI760D electrochemical workstation (Chenhua Instrument Shanghai Co., Ltd, China). A conventional three-electrode system was used for all electrochemical measurements: a GCE (4 mm in diameter) as the working electrode, a saturated calomel electrode (SCE) as the reference electrode, and the platinum wire electrode as the counter electrode. Scanning electron microscopy (SEM) images were collected using a FEI QUANTA FEG250 coupled with INCA Energy X-MAX-50.
Human AFP and antibody to human AFP (anti-AFP) were purchased from Shanghai Linc-Bio Science Co., Ltd, China. Hydrazine hydrate solution and ammonium hydroxide solution were purchased from Tianjin Fuyu Fine Chemical Co., Ltd., China. Cu(NO3)2·3H2O, NaOH, HAuCl4·4H2O, trisodium citrate and PDDA were purchased from Shanghai Aladdin Chemistry Co., Ltd, China. Phosphate buffered saline (PBS 1/15 M Na2HPO4 and KH2PO4) was used as an electrolyte for all electrochemistry measurements, which was purged with nitrogen gas for 20 min to remove the dissolved oxygen. All other reagents were of analytical grade and ultrapure water was used throughout the study.
2.2. Synthesis of the GS
Graphene oxide (GO) was synthesized by an improved Hummers method.25 In brief, 36 mL of concentrated H2SO4 and 4 mL of H3PO4 was added into a mixture of 0.3 g of graphite flakes and 1.8 g of KMnO4, producing a slight exotherm to 35–40 °C. The reaction was then heated to 50 °C and stirred for 12 h. After that, the reaction was cooled to room temperature and poured onto 40 mL of ice with 0.3 mL of 30% H2O2, and the mixture was centrifuged and the supernatant was decanted away. For workup, the remaining solid material was washed in succession with water, 30% HCl, ethanol and ether. The obtained solid was dried in vacuum overnight.
In a typical procedure for chemical conversion of GO to GS,26 0.1 g of GO was added into 100 mL of aqueous solution containing 87.5 μL of 80 wt% hydrazine hydrate solution and 1.568 mL of 25 wt% ammonium hydroxide solution. The weight ratio of hydrazine hydrate to GO was about 7
:
10. After vigorously stirred for a few minutes, the reaction was maintained at 95 °C for 1 h. The powdery GS was obtained after centrifugation, washed three times with water, and dried in vacuum overnight.
2.3. Synthesis of the CuO NFs
CuO NFs was synthesized by a simple method.27 NaOH solution was prepared by dissolving NaOH in water and Cu(NO3)2 solution was prepared by dissolving Cu(NO3)2·3H2O in ethanol. 1.0 mL of 0.5 M Cu(NO3)2 solution was added into 20.0 mL of 5 M NaOH solution at 0.5 mL min−1. The mixture was aged for 72 h at room temperature. The black precipitate was separated by centrifugation, washed three times with water and dried in vacuum overnight.
2.4. Synthesis of the Au NPs
Au NPs were synthesized by the classical Frens method.28 In brief, 100 mL of 0.01 wt% HAuCl4 aqueous solution was heated to boiling, and then 1.5 mL of 1 wt% trisodium citrate was added. The boiling solution turned a brilliant ruby-red in around 15 min, indicating the formation of Au NPs, and then it was cooled to room temperature.
2.5. Fabrication of the immunosensor
Fig. 1 shows the schematic diagram of the designed label-free electrochemical immunosensor, which was fabricated by a layer-by-layer self-assembly method. A GCE was polished repeatedly with 1.0, 0.3 and 0.05 μm alumina powder, sonicated in ethanol for 30 s, washed with ultrapure water thoroughly and dried with nitrogen flow. Firstly, the clean GCE was immersed in a 1 mg mL−1 GS dispersion containing 1 wt% PDDA for 30 min to adsorb a GS layer. Secondly, the modified GCE was immersed in a 1 mg mL−1 CuO NFs dispersion containing 1 wt% PDDA for 30 min to adsorb a CuO NFs layer. Thirdly, the modified GCE was immersed the obtained Au NPs solution and kept for 30 min to adsorb an Au NPs layer. The above procedures were repeated three times to get a unique and stable modified GCE by layer-by-layer self-assembly of GS–CuO NFs–Au NPs. Then, 6 μL of 10 μg mL−1 anti-AFP solution was added onto the modified GCE and dried at 4 °C. After washed with PBS, 3 μL of 1 wt% bovine serum albumin (BSA) solution was added to eliminate nonspecific binding sites. After that, the modified GCE was washed and incubated with various concentrations of AFP for 1 h at room temperature. Finally, the modified electrode was washed thoroughly to remove the unbounded AFP and ready for measurement with the amperometric i–t method, which was recorded in PBS at pH 6.8 at a detection potential of −0.4 V. 5 mM H2O2 was added into the PBS after the back ground current was stabilized.
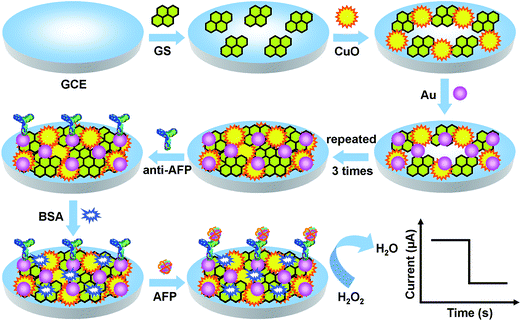 |
| Fig. 1 The schematic diagram of the label-free electrochemical immunosensor. | |
3. Results and discussion
3.1. SEM characterization
SEM images were used to confirm the successful synthesis of nanomaterials with different morphology. As shown in Fig. 2A, GS has a 2D lamellar folds nanostructure. The CuO NFs show a 3D flower-like nanostructure with a diameter of 4 μm (Fig. 2B). Au NPs (0D) exhibit a nearly spherical nanostructure with an average size of ∼25 nm (Fig. 2C). SEM images of GS–CuO NFs–Au NPs modified electrode were used to further confirm the successful modification of different nanomaterials on the surface of GCE. It can be observed that Au NPs are closely adsorbed on the surface of both GS (Fig. 2D) and CuO NFs (Fig. 2E and F). Therefore, the immunocomplex can be connected on the surface of GS–CuO NFs–Au NPs to decrease the synergic effect and prevent the electrocatalytic process towards the reduction of H2O2.
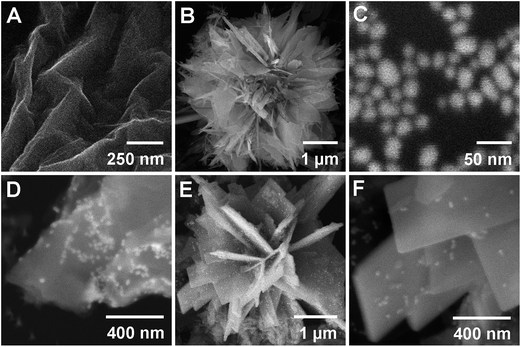 |
| Fig. 2 SEM images of GS (A), CuO NFs (B), Au NPs (C) and GS–CuO NFs–Au NPs modified electrode (D, E and F). | |
3.2. Electrochemical characterization
In order to investigate the signal amplification strategy of the process of layer-by-layer self-assembly, amperometric i–t curve method was employed to characterize the electrocatalytic performances of different nanomaterials towards the reduction of H2O2. It can be seen in the Fig. 3A that either GS (curve a) or Au NPs (curve b) has no obvious electrocatalytic current response. Even when GS–Au NPs (curve d) are modified on the GCE, which consists of both GS and Au NPs, the electrocatalytic current response of it is no significant difference. It can be concluded that both GS and Au NPs have no electrocatalytic performances towards the reduction of H2O2. However, the CuO NFs exhibit an electrocatalytic current response of about 50 μA (curve c). It indicates that CuO NFs have good electrocatalytic performances towards the reduction of H2O2. Interestingly, it can be observed that the electrocatalytic current response of CuO NFs–Au NPs (curve e) increases obviously than that of pure CuO NFs. It indicates that Au NPs, which has good biocompatibility and can be used for the capture of antibodies in the fabrication of immunosensor, can produce a synergic effect with CuO NFs to increase the electrocatalytic performances of CuO NFs. In addition, it can also be observed that the electrocatalytic current response of GS–CuO NFs (curve f) increases obviously than that of pure CuO NFs. It indicates that GS, which has fast electron transfer and good biocompatibility, can also produce a synergic effect with CuO NFs to increase the electrocatalytic performances of CuO NFs. After the process of layer-by-layer self-assembly of GS–CuO NFs–Au NPs (curve g), the electrocatalytic current response increases to about 100 μA, displaying that the electrochemical signal is amplified twice. It indicates that the good synergic effect between GS, CuO NFs and Au NPs can produce a positive effect on the electrocatalytic performance of CuO NFs. In addition, it well indicates that the GS, CuO NFs and Au NPs were successfully assembled layer-by-layer on the surface of GCE. In conclusion, the signal amplification strategy is based on the good synergic effect between GS, CuO NFs and Au NPs.
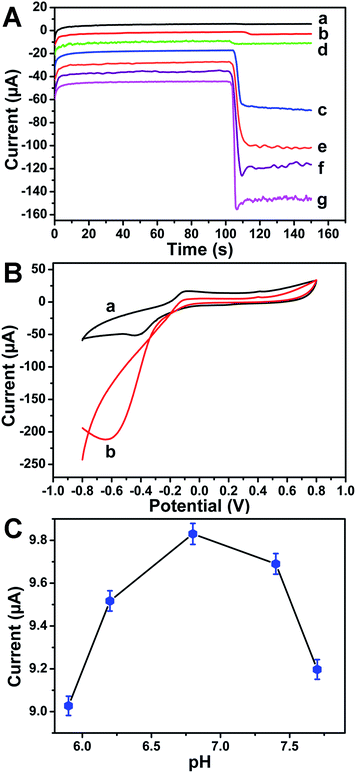 |
| Fig. 3 (A) Electrocatalytic current responses towards 5 mM H2O2 of GS (curve a), Au NPs (curve b), CuO NFs (curve c), GS–Au NPs (curve d), CuO NFs–Au NPs (curve e), GS–CuO NFs (curve f) and GS–CuO NFs–Au NPs (curve g) in PBS at pH 6.8; (B) cyclic voltammograms of the GS–CuO NFs–Au NPs modified electrode before (curve a) and after (curve b) the addition of 5 mM H2O2; (C) effect of pH on the electrocatalytic current responses of the proposed immunosensor for the detection of 1 pg mL−1 AFP. Error bar = RSD (n = 5). | |
In order to investigate the electrocatalytic mechanism of GS–CuO NFs–Au NPs towards the reduction of H2O2, cyclic voltammetry was employed to further characterize the electrocatalytic performance. Fig. 3B displays cyclic voltammograms (CVs) of the GS–CuO NFs–Au NPs modified electrode before and after the addition of 5 mM H2O2 in PBS at pH = 6.8 before and after the addition of 5 mM H2O2. Before the addition of H2O2 (curve a), CV of the GS–CuO NFs–Au NPs modified electrode exhibits a redox activity, which is produced by CuO NFs. After the addition of H2O2 (curve b), a dramatic increase of the reduction current was observed, demonstrating the good electrocatalytic performance of GS–CuO NFs–Au NPs towards the reduction of H2O2 and the high sensitivity of the immunosensor. According to the literature,29 electrocatalytic mechanism could be expressed as following:
|
2CuO + 2H+ + 2e− → Cu2O + H2O;
| (1) |
|
Cu2O + H2O2 → 2CuO + H2O.
| (2) |
In order to achieve an optimal electrochemical signal, the optimization of experimental conditions is necessary. As shown in the electrocatalytic mechanism, H+ can participate in the electrocatalytic process. Therefore, the value of pH can influence the electrocatalytic process towards the reduction of H2O2. In addition, the specific recognition between antibodies and antigens will be broke under acidic or alkaline conditions. Therefore, the value of pH can also influence the activity of biological materials. The Fig. 3C shows the different electrocatalytic current responses of the proposed immunosensor for the detection of 1 pg mL−1 AFP in different pH values of PBS at a detection potential of −0.4 V. As shown in this figure, the optimal amperometric response was achieved at pH = 6.8. Higher or lower pH has negative influence on both the electrocatalytic process towards the reduction of H2O2 and the activity of biological materials. Therefore, PBS at pH = 6.8 was selected for the test throughout this study.
3.3. Characterization of the immunosensor
In this study, A.C. impedance method was employed to characterize fabrication process of the proposed immunosensor. As shown in the Fig. 4A, Nyquist plots of the A.C. impedance method was recorded from 1 to 105 Hz at 0.24 V in a solution containing 0.1 M KCl and 2.5 mM Fe(CN)63−/Fe(CN)64−. Nyquist plots are consist of two portions. The linear portion at low frequencies is associated with electrochemical behavior limited by diffusion. The semicircle portion at high frequencies is associated with the electrochemical process subject to electron transfer, where the diameter corresponds to the resistance. Simply, resistance change could be judged by observing the diameter change of semicircle portion. Thus, A.C. impedance is a suitable method for monitoring the changes in the surface features during the fabrication process.30 It can be observed that the bare GCE exhibits a very small resistance (curve a), which is characteristic of a diffusion-limiting step in the electrochemical process. After the process of lay-by-layer self-assembly, the electrode shows a smaller resistance (curve b), implying that the GCE modified with GS–CuO NFs–Au NPs has an better electron transfer ability than the bare GCE. The gradually increasing resistance of electrodes further modified with anti-AFP (curve c), BSA (curve d) and AFP (curve e) indicates the successful immobilization of the non-conductive bioactive substances. It indicates that GS–CuO NFs–Au NPs can not only offer a biocompatible surface for protein capturing but also provides a sensitive electric interface for further biosensing.
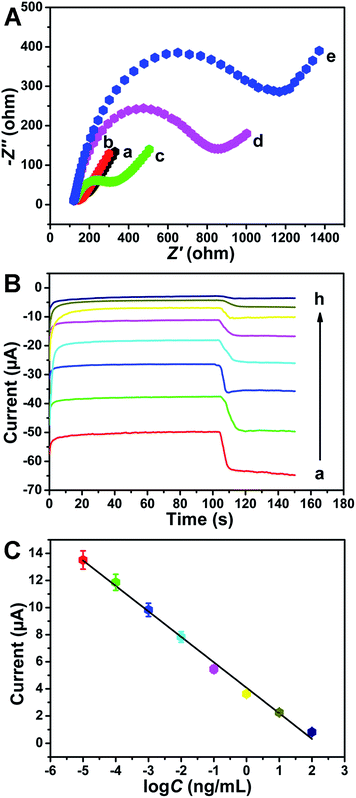 |
| Fig. 4 (A) Nyquist plots of the A.C. impedance method: bare GCE (a), GS–CuO NFs–Au NPs/GCE (b), anti-AFP/GS–CuO NFs–Au NPs/GCE (c), BSA/anti-AFP/anti-AFP/GS–CuO NFs–Au NPs/GCE (d), AFP/BSA/anti-AFP/anti-AFP/GS–CuO NFs–Au NPs/GCE/GCE (e); (B) electrocatalytic current responses of the immunosensor for the detection of different concentrations of AFP: 10−5 ng mL−1 (a), 10−4 ng mL−1 (b), 10−3 ng mL−1 (c), 10−2 ng mL−1 (d), 0.1 ng mL−1 (e), 1 ng mL−1 (f), 10 ng mL−1 (g) and 100 ng mL−1 (h); (C) calibration curve of the immunosensor for the detection of different concentrations of AFP. Error bar = RSD (n = 5). | |
Under optimal conditions, the label-free electrochemical immunosensor based on the layer-by-layer self-assembly method was employed to detect different concentrations of AFP. Fig. 4B shows the electrocatalytic current responses of the proposed immunosensor for the detection of AFP covering the concentration range from 10−5 ng mL−1 (curve a) to 102 ng mL−1 (curve h). Fig. 4C shows a linear relationship between electrocatalytic current responses and the logarithmic values of AFP concentration. When the concentration of AFP increased at high concentration range, the decrease rate of electrocatalytic current response slowed down, due to steric hindrance or saturation of couple antigen molecules.31,32 Therefore, the electrocatalytic current responses have a linear relationship with the logarithmic values of AFP concentration. And the linear regression equation of the calibration curve was I = 4.08 − 1.88 log
C with correlation coefficient of 0.99. The low detection limit of 5.3 fg mL−1 was obtained, which was ascribed to signal amplification strategy based on the layer-by-layer self-assembly of GS–CuO NFs–Au NPs in the fabrication of the designed immunosensor.
3.4. Reproducibility, selectivity and stability
To evaluate the reproducibility of the label-free electrochemical immunosensor, a series of five electrodes were prepared for the detection of 0.1 pg mL−1 of AFP (Fig. S1A†). The relative standard deviation (RSD) of the measurements for the five electrodes was less than 5%, suggesting the precision and reproducibility of the proposed immunosensor was quite good.
To investigate the specificity of the label-free electrochemical immunosensor, interference study was performed by using ascorbic acid (AA), carcinoembryonic antigen (CEA), human immunoglobulin (IgG) and BSA. The 1 pg mL−1 of AFP solution containing 100 pg mL−1 of interfering substance was measured by the designed immunosensor. As shown in Fig. S1B,† the electrocatalytic current response variation due to the interfering substance was less than 5% of that without the interference, indicating the selectivity of the proposed immunosensor was acceptable.
To test the stability of the label-free electrochemical immunosensor, it was stored at 4 °C when not in use. After one month, no apparent change for the detection of the same AFP concentration was found. The good stability could be ascribed to the good biocompatibility of the layer-by-layer self-assembly of GS–CuO NFs–Au NPs. The reproducibility, selectivity and stability of this proposed immunosensor were all acceptable, thus it was suitable for quantitative detection of AFP in real human samples.
3.5. Real sample analysis
In order to validate the proposed immunosensor, a comparison with the commercialized available enzyme-linked immunosorbent assay (ELISA) method for the detection of AFP in human serum sample is showed in Table 1. The relative error between the two methods was in the range from −3.8–4.8%. These data revealed a good agreement between the two analytical methods, indicating the feasibility of the label-free electrochemical immunosensor for clinical application.
Table 1 Human serum sample analysis using the proposed method and the ELISA method
Sample |
This methoda (ng mL−1) |
ELISAa (ng mL−1) |
Relative error (%) |
Each value is the average of five measurements. |
1 |
2.3 |
2.2 |
4.5 |
2 |
5.1 |
5.3 |
−3.8 |
3 |
8.8 |
8.4 |
4.8 |
4. Conclusions
This work has developed a label-free electrochemical immunosensor for the quantitative detection of AFP. Layer-by-layer self-assembly of GS, CuO NFs and Au NPs was employed to achieve the high sensitivity of the immunosensor. The designed immunosensor displayed a wide detection range, a low detection limit, acceptable reproducibility, selectivity and stability. The designed immunosensor will be a promising tool for clinical cancer diagnosis.
Acknowledgements
This study was supported by the Natural Science Foundation of China (nos 21175057, 21375047 and 21377046), the Science and Technology Development Plan of Shandong Province (no. 2014GSF120004), the Science and Technology Plan Project of Jinan (no. 201307010), Achievements Transformation of Shandong Province (no. 2014ZZCX05101) and QW thanks the Special Foundation for Taishan Scholar Professorship of Shandong Province and UJN (no. ts20130937).
References
- S. Cheng, S. Hideshima, S. Kuroiwa, T. Nakanishi and T. Osaka, Sens. Actuators, B, 2015, 212, 329–334 CrossRef CAS PubMed.
- B. Rapp, F. Gruhl and K. Länge, Anal. Bioanal. Chem., 2010, 398, 2403–2412 CrossRef CAS PubMed.
- J. Narayanan, M. K. Sharma, S. Ponmariappan, Sarita, M. Shaik and S. Upadhyay, Biosens. Bioelectron., 2015, 69, 249–256 CrossRef CAS PubMed.
- J. Liu, G. Lin, C. Xiao, Y. Xue, A. Yang, H. Ren, W. Lu, H. Zhao, X. Li and Z. Yuan, Biosens. Bioelectron., 2015, 71, 82–87 CrossRef CAS PubMed.
- Z.-H. Yang, Y. Zhuo, R. Yuan and Y.-Q. Chai, Biosens. Bioelectron., 2015, 69, 321–327 CrossRef CAS PubMed.
- Y. Wang, X. Li, W. Cao, Y. Li, H. Li, B. Du and Q. Wei, Biosens. Bioelectron., 2014, 61, 618–624 CrossRef CAS PubMed.
- J. Han, Y. Zhuo, Y. Chai, Y. Xiang, R. Yuan, Y. Yuan and N. Liao, Biosens. Bioelectron., 2013, 41, 116–122 CrossRef CAS PubMed.
- X. Wang, L. Chen, X. Su and S. Ai, Biosens. Bioelectron., 2013, 47, 171–177 CrossRef CAS PubMed.
- L. Li, J. Xu, X. Zheng, C. Ma, X. Song, S. Ge, J. Yu and M. Yan, Biosens. Bioelectron., 2014, 61, 76–82 CrossRef CAS PubMed.
- C. Zhu, G. Yang, H. Li, D. Du and Y. Lin, Anal. Chem., 2015, 87, 230–249 CrossRef CAS PubMed.
- D. Wu, H. Fan, Y. Li, Y. Zhang, H. Liang and Q. Wei, Biosens. Bioelectron., 2013, 46, 91–96 CrossRef CAS PubMed.
- Q. Wei, Z. Xiang, J. He, G. Wang, H. Li, Z. Qian and M. Yang, Biosens. Bioelectron., 2010, 26, 627–631 CrossRef CAS PubMed.
- L. Ding, A. M. Bond, J. Zhai and J. Zhang, Anal. Chim. Acta, 2013, 797, 1–12 CrossRef CAS PubMed.
- Y. Wang, Z. Guo, H. Ma, Y. Li, W. Cao, B. Du and Q. Wei, RSC Adv., 2014, 4, 59106–59113 RSC.
- Y. Wang, Y. Li, H. Ma, X. Ren, W. Cao, T. Yan and Q. Wei, RSC Adv., 2015, 5, 31262–31269 RSC.
- Y. Zhang, M. A. Arugula, M. Wales, J. Wild and A. L. Simonian, Biosens. Bioelectron., 2015, 67, 287–295 CrossRef CAS PubMed.
- T. Cassagneau, F. Guérin and J. H. Fendler, Langmuir, 2000, 16, 7318–7324 CrossRef CAS.
- D. M. DeLongchamp and P. T. Hammond, Langmuir, 2004, 20, 5403–5411 CrossRef CAS.
- S. Wang, D. Yu and L. Dai, J. Am. Chem. Soc., 2011, 133, 5182–5185 CrossRef CAS PubMed.
- M. Zhang, Y. Yan, K. Gong, L. Mao, Z. Guo and Y. Chen, Langmuir, 2004, 20, 8781–8785 CrossRef CAS PubMed.
- D.-Q. Yang, J.-F. Rochette and E. Sacher, J. Phys. Chem. B, 2005, 109, 4481–4484 CrossRef CAS PubMed.
- F. Xu, M. Deng, G. Li, S. Chen and L. Wang, Electrochim. Acta, 2013, 88, 59–65 CrossRef CAS PubMed.
- H. Chen, M. B. Müller, K. J. Gilmore, G. G. Wallace and D. Li, Adv. Mater., 2008, 20, 3557–3561 CrossRef CAS PubMed.
- Y. Li, Z. Zhong, Y. Chai, Z. Song, Y. Zhuo, H. Su, S. Liu, D. Wang and R. Yuan, Chem. Commun., 2012, 48, 537–539 RSC.
- D. C. Marcano, D. V. Kosynkin, J. M. Berlin, A. Sinitskii, Z. Sun, A. Slesarev, L. B. Alemany, W. Lu and J. M. Tour, ACS Nano, 2010, 4, 4806–4814 CrossRef CAS PubMed.
- D. Li, M. B. Muller, S. Gilje, R. B. Kaner and G. G. Wallace, Nat. Nanotechnol., 2008, 3, 101–105 CrossRef CAS PubMed.
- Z. Yang, J. Xu, W. Zhang, A. Liu and S. Tang, J. Solid State Chem., 2007, 180, 1390–1396 CrossRef CAS PubMed.
- G. Frens, Nature, 1973, 241, 20–22 CAS.
- P. Gao, Y. Gong, N. P. Mellott and D. Liu, Electrochim. Acta, 2015, 173, 31–39 CrossRef CAS PubMed.
- W. Lu, X. Cao, L. Tao, J. Ge, J. Dong and W. Qian, Biosens. Bioelectron., 2014, 57, 219–225 CrossRef CAS PubMed.
- K. Wang, J. Liao, X. Yang, M. Zhao, M. Chen, W. Yao, W. Tan and X. Lan, Biosens. Bioelectron., 2015, 63, 172–177 CrossRef CAS PubMed.
- J. Huang, G. Yang, W. Meng, L. Wu, A. Zhu and X. a. Jiao, Biosens. Bioelectron., 2010, 25, 1204–1211 CrossRef CAS PubMed.
Footnote |
† Electronic supplementary information (ESI) available. See DOI: 10.1039/c5ra07547e |
|
This journal is © The Royal Society of Chemistry 2015 |