DOI:
10.1039/C5RA05299H
(Paper)
RSC Adv., 2015,
5, 44408-44415
Building cell-containing multilayered phospholipid polymer hydrogels for controlling the diffusion of a bioactive reagent†
Received
25th March 2015
, Accepted 24th April 2015
First published on 27th April 2015
Abstract
Cytocompatible and multilayered phospholipid polymer hydrogels containing living cells and a specific bioactive reagent were used to determine the role of diffusion of a bioactive reagent in regulating cell-fate. Within the multilayered hydrogels prepared by a layer-by-layer assembling process, a bioactive reagent reservoir layer and a cell-laden layer were separated with a finely formed dual-crosslinked hydrogel multilayer that was composed of a 2-methacryloyloxyethyl phosphorylcholine polymer and photo-reactive poly(vinyl alcohol). The dual-crosslinked hydrogel was less permeable than the single-crosslinked hydrogel and thus served as a diffusion-controlling barrier. We demonstrated the use of this multilayered hydrogel by subjecting the human cervical cancer HeLa cell-line to paclitaxel. We found that cell viability was regulated by the thickness of the diffusion-controlling barrier and the bioactive reagent concentration. An increase in the barrier thickness and a decrease in the bioactive reagent concentration resulted in decreased apoptosis of the HeLa cells. Our results suggest that the gradient of a bioactive reagent formed was due to the diffusion-controlling barriers, which explains the observed diffusion-dependent cell behavior. This study provides insight into the regulation of bioactive reagent diffusion in micrometer scale hydrogels and the associated diffusion-dependent effects on cell behavior. This finding can contribute to the design of a platform for studying diffusion-dependent cell behavior for tissue engineering and regenerative medicine applications.
Introduction
Hydrogel matrices contain a large amount of water, therefore, they can provide viscoelastic mechanical properties similar to many soft tissues and permit diffusion of water as well as nutrients, proteins and signaling molecules.1–3 Altogether, they mimic some key features of the native extracellular matrix (ECM) and facilitated cell spreading, migration and differentiation.4,5 Diffusion of bioactive reagents plays an important role in gradient formation within three-dimensional (3D) matrices, which are involved in the regulation of cell behavior.6–8 Mimicry of these gradients in hydrogels could be particularly important in functional tissue engineering approaches, which aim to use combinations of cells, materials, and bioactive reagents to recreate natural tissue structure and function.1
In recent years, there have been increasing studies that try to implement soluble or covalently-attached gradients of growth factors, cytokines or adhesion sequences into 3D hydrogel matrices in order to control cell behavior such as migration, proliferation, apoptosis and differentiation.6,9–14 Several approaches such as photolithographic technology,10,11 soft lithographic processes,12 and microfluidic technology,13,14 have been used to spatially control the diffusional properties of hydrogels. While these new approaches represent great progress, each one has its specific drawbacks, such as complicated manipulations or lengthy constructions.
Layer-by-layer (LbL) assembly technology, which can mimic the highly organized and stratified architectures of biological tissues, can be useful for the integration of specific reagents into biomaterials. Several studies using this technology have been carried out.15–17 It is an economical, easily applicable, and versatile tool for the controlled fabrication of surface coatings or tissue scaffolds.
A variety of bioactive reagents, such as proteins, DNA, RNA, and nanoparticles, can be incorporated into single layers to functionalize multilayered assembly.11–13 However, until recently, stable incorporation of cells into single layers of the LbL assembly was a challenge, with the exception of a cell spraying LbL procedure.18 For the first time, we have reported a spinning-assisted LbL procedure that allows precise spatial control of multiple cell types in 3D without cellular damage.19 This method has been previously used to encapsulate tumor cells and stroma cells to study distance-dependent cell–cell interactions.20
When a water-soluble phospholipid polymer, poly[2-methacryloyloxyethyl phosphorylcholine (MPC)-co-n-butyl methacrylate (BMA)-co-p-vinylphenylboronic acid (VPBA)] (PMBV) and poly(vinyl alcohol) (PVA) were mixed together, based on the specific reaction between the VPBA unit in PMBV and the diol group in PVA, a hydrogel (PMBV/PVA) was spontaneously formed at room temperature. This hydrogel formation occurs under mild reaction conditions and can effectively encapsulate biological components, such as bacteria,21 living cells,22,23 and bioactive reagents.24,25
In this study, photo-reactive PVA (azido unit pendant water-soluble photopolymer, AWP)26 was used to prepare a dual-crosslinked hydrogel (PMBV/AWP) to increase the cross-linking degree of the hydrogel. Thus, this hydrogel plays the role of a diffusion-controlling barrier, by which the diffusion rate of the bioactive reagents contained in the hydrogel can be controlled as a function of the thickness of the barrier layer. We used the anticancer bioactive reagent, paclitaxel (PTX), as a model bioactive reagent. Although PTX is extremely hydrophobic, it can be dissolved in a PMBV aqueous solution with high efficiency due to the presence of hydrophobic domains in aqueous medium.24,25 Therefore, PTX can be loaded in situ into the PMBV/PVA hydrogel to prepare a reservoir layer of the bioactive reagent. What’s more, we developed a spinning-assisted LbL method for building a sandwich multilayered hydrogel platform, that is (PMBV/PVA as a PTX-loaded layer)–(PMBV/AWP as a diffusion-controlling barrier)–(PMBV/PVA as a cell-incorporated layer).19,20
The aim of this study was to investigate the diffusion of a bioactive reagent in sandwich structured multilayered hydrogels, and to determine the effect of the thickness of the diffusion-controlling barrier and the concentration of the bioactive reagent on cell behavior. The proposed methodology may contribute to the technological advancement of tissue engineering by offering the possibility to regulate the biomaterial composition and control different biological effects during tissue reconstruction.
Experimental section
Materials
MPC was synthesized by a previously reported method27 and purchased from NOF Co. Ltd. (Tokyo, Japan). BMA was purchased from Kanto Chemicals (Tokyo, Japan); VPBA, PVA (mean polymerization degree = 1.0 × 103), and PTX were purchased from Wako Pure Chemical Industries, Ltd. (Osaka, Japan). Methacryloxyethyl thiocarbonyl rhodamine B (MTR) was purchased from Polysciences (PA, USA). Fluorescence-labeled PTX (Oregon-green 488-labeled) was purchased from Invitrogen (Carlsbad, CA, USA). AWP was purchased from Tokyo Gosei Co. Ltd., Japan. Other organic reagents and solvents were commercially available reagents of extra-pure grade and were used without further purification.
PMBV was synthesized by a conventional free radical polymerization of corresponding monomers, as previously described.19,20 The mole fractions of MPC, BMA, and VPBA units in the obtained PMBV were determined by 1H-NMR (JNM-NR30; JEOL, Tokyo, Japan) and are 0.60, 0.25, and 0.15, respectively. The average molecular weight of PMBV was 1.7 × 104, as determined by a gel permeation chromatographic system (Jasco, Tokyo, Japan) with poly(ethylene oxide) standards. Fluorescence-labeled PMBV, poly(MPC-co-BMA-co-VPBA-co-MTR) (PMBV-R) was synthesized by radical polymerization.19 The chemical structures of PMBV and AWP are shown in Fig. 1a. The dual-crosslinking reaction between PMBV and AWP is shown in Fig. 1b.
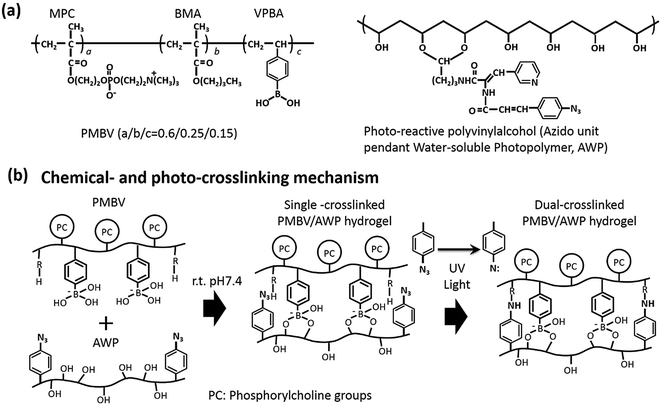 |
| Fig. 1 (a) The chemical structure of PMBV and AWP, and (b) the dual-crosslinking mechanism of the PMBV/AWP hydrogel. | |
Cell culture procedure
We used human cervical cancer HeLa cells that showed a stable expression of fluorescent ubiquitination-based cell cycle indicator (Fucci) fusion proteins. The Fucci fusion proteins were used as cell cycle sensors to perform quantitative analysis of cell activity kinetics.28 HeLa-Fucci cells were purchased from Riken Cell Bank (Ibaraki, Japan). They were cultured with DMEM (Sigma-Aldrich, St. Louis, MO) supplemented with 10% fetal bovine serum at 37 °C in a 5.0 vol% CO2 humidified atmosphere.
Solubilization of PTX with the polymer solutions
PTX was dissolved in a PMBV solution.24 Briefly, PTX (1.0 mg mL−1) was dissolved in 99.5 wt% ethanol, and PMBV was dissolved in PBS with polymer concentrations of 50 mg mL−1. Then a PTX-loaded PMBV solution was prepared by vortexing a mixture of the PTX and PMBV solutions at a ratio of PMBV/PTX = 90/10, and then removing the ethanol under reduced pressure. The PTX that was not dissolved was removed by filtration. The final PTX concentration in the PMBV solution was 75 μM (data not shown).
Preparation of dual-crosslinked PMBV/AWP multilayer hydrogels
The PMBV(-R) solution (5.0 wt%) was prepared in PBS. The AWP solution (2.0 wt%) was prepared using a diluted stock solution (6.0 wt%) comprised of a mixture of 99.5 wt% ethanol and PBS at a ratio of PBS/ethanol = 30/10. The PMBV/AWP multilayer hydrogel was prepared by a spinning assisted LbL procedure (Fig. 2) similar to our previous report.15,16 Briefly, the AWP solution was manually spread on a 35 mm plastic culture dish to form a thin membrane. AWP-coated dishes were then air-dried in a fume hood at room temperature. Then they were irradiated for 1.0 min with UV light (50 mW cm−2) from a UV Spot Cure (SP-7, Ushio Inc., Yokohama, Japan). Then the PMBV solution was deposited onto the AWP coated surface. After 1.0 min, to allow the boronic acid–diol interaction, the dish was rotated at 2000 rpm to remove the excess PMBV solution and form a layer of PMBV/AWP membrane. Next, the AWP solution and PMBV solutions were alternately deposited to form a (PMBV/AWP)–(PMBV/AWP)n hydrogel membrane, where the value of n (n = 1, 3, 5) indicates the number of PMBV/AWP layers. Finally, the multilayered hydrogel membranes were air-dried, irradiated with UV light (50 mW cm−2) for 3.0 min and submerged in PBS. With the help of PMBV-R to indicate the PMBV distribution in the multilayered hydrogels, the structure and thickness of the multilayered hydrogels were characterized using a Zeiss LSM 510 confocal laser-scanning microscope (CLSM; Carl Zeiss, Oberkochen, Germany).
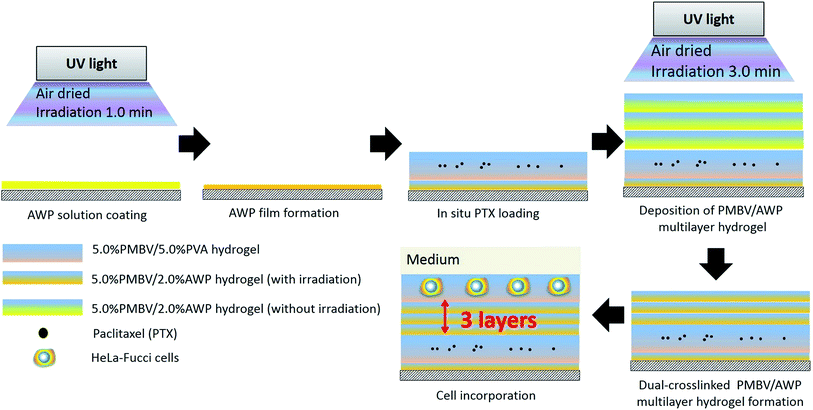 |
| Fig. 2 Fabrication of the multilayered hydrogel with encapsulation of PTX and cells. | |
For the sample preparation for FT-IR reflection absorption spectroscopy (RAS), the dual-crosslinked multilayered hydrogels were applied onto Au spattered glass substrates and irradiated with UV light for 0 or 3.0 min. After drying overnight in a desiccator, the membranes were measured by FT-IR RAS (FT-IR 6300/Jasco).
Incorporation of a bioactive reagent and cells into the multilayered hydrogels
The following procedure is shown in Fig. 2. HeLa-Fucci cells were dispersed in 5.0 wt% PMBV solution, and a 5.0 wt% PMBV solution containing PTX (750 nM, 375 nM, and 188 nM) was also prepared. Briefly, the 5.0 wt% PVA solution was dropped on an irradiated PMBV/AWP layer and spun at 2000 rpm followed by the deposition of 50 μL PMBV solution containing PTX. Then the PTX loaded layer was alternately deposited with AWP solution and PMBV solution to form a (PMBV/AWP)–(PMBV/PTX/PVA)–(PMBV/AWP)n multilayer hydrogel, where the value of n = 1, 3, and 5. Next, 4.0 μL PMBV/HeLa-Fucci cell suspension was spotted on the top of the growing multilayered hydrogel. The final structure was denoted as a (PMBV/AWP)–(PMBV/PTX/PVA)–(PMBV/AWP)n–(PMBV/HeLa-Fucci cell/PVA)–(PMBV/PVA) multilayer hydrogel. Finally, 2.0 mL DMEM was added to each dish and all the samples were incubated at 37 °C in a 5.0 vol% CO2 humidified atmosphere for 48 h.
We used four groups to evaluate the cell response as a function of the diffusion-controlling barrier thickness, which are shown in Table 1: a thick barrier group (5 layers PMBV/AWP), an intermediate barrier group (3 layers PMBV/AWP), a thin barrier group (1 layer PMBV/AWP), and a control group (5 layers PMBV/PVA).
Table 1 Experimental groups to study HeLa-Fucci cells associated with PTX stimulation under different conditions
Experimental group |
Number of hydrogel layersa |
Concentration of PTX |
Number of hydrogel layers between the PTX-loaded layer and the HeLa-Fucci cell laden layer.
Number of PMBV/AWP hydrogel layers (diffusion-controlling barrier).
Number of PMBV/PVA hydrogel layers.
|
Thick barrier |
5b |
375 nM |
Intermediate barrier |
3b |
375 nM |
Thin barrier |
1b |
375 nM |
Control |
5c |
375 nM |
High concentration |
1b |
750 nM |
Intermediate concentration |
1b |
375 nM |
Low concentration |
1b |
188 nM |
Another three groups were used to evaluate the cell response as a function of the bioactive reagent concentration, with a constant barrier thickness (1 layer PMBV/AWP), including a high concentration group (750 nM PTX), an intermediate concentration group (375 nM PTX), and a low concentration group (188 nM PTX).
Evaluation of the cell proliferation-cycle distribution and cell viability
The numbers, positions, and fluorescence of HeLa-Fucci cells were measured using a BIOREVO BZ-9000 fluorescence microscope (Keyence, Osaka, Japan) at 12 h and 48 h. For quantitative analysis of fluorescent cells, at least 300 cells in five independent fields were analyzed.
Cells designated as apoptotic were those that displayed the characteristic morphological features of apoptosis, including cell volume shrinkage, chromatin condensation, and the presence of membrane-bound apoptotic bodies. According to this apoptotic cell morphology, the percentage of apoptotic cells was calculated.
Evaluation of bioactive reagent diffusivity in dual-crosslinked PMBV/AWP multilayer hydrogels
For the evaluation of bioactive reagent diffusion in the PMBV/AWP hydrogels, the diffusion coefficient of PTX-F was determined by fluorescence correlation spectroscopy (FCS) measurements (CLSM, Carl Zeiss) as previously described.29 The PTX-F/PBS solution (200 nM, 2.0 mL) was added onto multilayered PMBV/AWP (0 min irradiation), multilayered PMBV/AWP (3.0 min irradiation), and multilayered PMBV/PVA, which were built on 35 mm (glass 27ϕ) glass base dishes (Iwaki) and stored at 37 °C for 24 h to equilibrate the samples. Then the diffusion coefficients of PTX-F were determined at eight positions within the multilayered hydrogels to get an average value. The experiments were repeated three times.
In order to observe the diffusion process of PTX-F within the multilayered hydrogel, a (PMBV-R/AWP)–(PMBV/2.0 μM PTX-F/PVA)–(PMBV-R/AWP)3–(PMBV-R/PVA) (PMBV/PVA) multilayer hydrogel was prepared. Cross-section fluorescence images of the multilayered hydrogel were taken at 0.5, 12, and 48 h under the same settings using a confocal laser-scanning microscope (Carl Zeiss).
Statistical analysis of the data
A student’s t-test was carried out to determine whether the observed differences were statistically significant (p < 0.05).
Results and discussion
Fabrication of multifunctional hydrogel layers
The LbL assembling procedure was applied for the preparation of multilayered hydrogels composed of PMBV and PVA. We successfully controlled the thickness of the multilayered hydrogels by changing both the polymer concentration and the number of layers.19
In this study, we embedded PTX in a PMBV/PVA layer, and then separated this layer from a cell-containing PMBV/PVA layer by PMBV/AWP layers. These multilayered hydrogels are novel because: (1) their micrometer size allows for the incorporation of both bioactive reagents and cells in their architecture, which would not be easily achieved by other LbL technologies, and (2) separating the bioactive reagent-loaded layer and the cell-containing layer with different thicknesses of PMBV/AWP layers in a multilayered hydrogel allows for fine-tuned accessibility of bioactive reagents into the cells.
As shown in Fig. 3, confocal microscopy imaging shows a multilayered hydrogel consisting of fluorescent-labeled PMBV, fluorescent-labeled PTX, and HeLa-Fucci cells. In the vertical cross-section, a sandwich structure of the bioactive reagent loaded layer, diffusion-controlling barrier, and cell-incorporated layer was visualized. As shown in Fig. 4a and b, the thickness of dual-crosslinked PMBV/AWP could be finely tuned by varying the number of gel layers. The HeLa-Fucci cell-laden PMBV/PVA layer was on top of the dual-crosslinked PMBV/AWP layers. The thickness of one layer of 5.0%-PMBV/5.0%-PVA was about 15–20 μm, which was enough to hold cells. Our previous research proved that the concentration of PVA was very important to form a cell-containing layer, because it could provide enough thickness and mechanical properties to hold the cells due to its relatively high viscosity.19
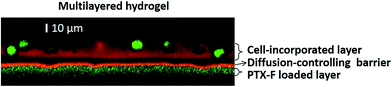 |
| Fig. 3 Confocal microscopy observation of the structure of a multilayered hydrogel with the encapsulation of PTX and cells: (PMBV-R/AWP)–(PMBV/PTX-F/PVA)–(PMBV-R/AWP)3–(PMBV-R/HeLa-Fucci cell/PVA)–(PMBV/PVA) (scale bars: 10 μm). | |
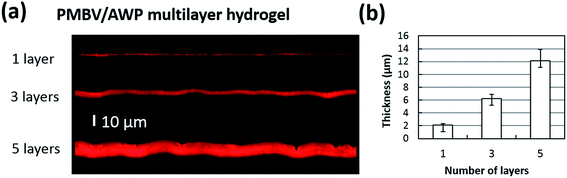 |
| Fig. 4 Thickness regulation of the PMBV/AWP multilayer hydrogel. (a) Confocal Z section of the dual-crosslinked PMBV-R/AWP multilayer hydrogel and (b) the thickness of the PMBV/AWP multilayer hydrogels exhibit a linear growth behavior with the increase in the number of layers (scale bars: 10 μm). | |
To confirm the photoreaction in the dual-crosslinked hydrogel membranes, dried membranes were measured by FT-IR RAS (see Fig. S1 in the ESI†). A specific peak of the azide group was detected at 2123 cm−1 before UV irradiation. This peak was eliminated after UV irradiation, indicating a photochemical-crosslinking of the azide group.
Bioactive reagent diffusivity within multilayered hydrogels
To understand the permeability of the dual-crosslinked hydrogels, we measured the diffusion coefficient of PTX-F across different multilayered hydrogels. As shown in Fig. 5a, the diffusion coefficient of PTX-F across the PMBV/AWP hydrogel (photoirradiation for 3.0 min) was much lower than the single-crosslinked PMBV/AWP hydrogel (no irradiation) or PMBV/PVA. A probable reason for this phenomenon is that dual-crosslinked PMBV/AWP has the highest crosslinking degree, which prevents the diffusion of PTX-F. Therefore, PMBV/AWP could work as a diffusion-controlling barrier and generate unique spatial gradients of PTX-F. As shown in Fig. 5b, PTX-F was encapsulated into a reservoir, and was allowed to gradually diffuse through the diffusion-controlling barrier. Over the course of 48 h, green fluorescent PTX-F gradually emanated, providing a spatial gradient field over a micrometer-size scale (Fig. 5b and c). The evolution of the PTX-F intensity gradients demonstrated that the gradient gradually decreased with time and reached equilibrium after 48 h (Fig. 5c).
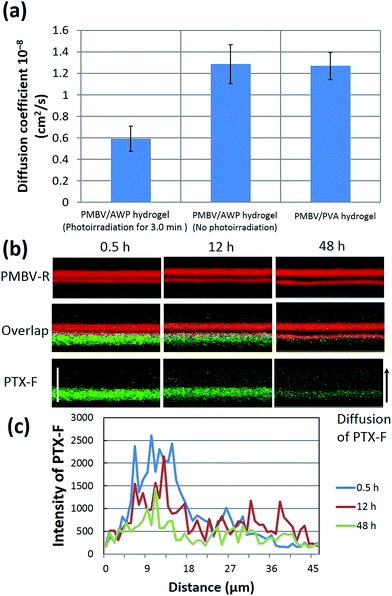 |
| Fig. 5 Bioactive reagent diffusivity within multilayered hydrogels. (a) The diffusion coefficients of PTX-F determined within multilayered hydrogels. (b) Time lapse images of a functionalized multilayer hydrogel dosed with 2 μM fluorescence labeled PTX (PTX-F) in the bottom layer. (c) Fluorescent intensities were obtained starting from the bottom of the gel and to a distance of 45 μm from the bottom for three different time points. The fluorescent intensity of PTX-F was plotted as a function of distance from the bottom (scale bars: 45 μm). | |
One point that should be emphasized here is the constant permeability of the PMBV/PVA (or AWP) hydrogel under cell culture conditions. As mentioned above, the PMBV/PVA hydrogel is a chemical crosslinked hydrogel. The boronic acid/diol complex can be dissociated by sugar solutions, such as sorbitol, which has a stronger affinity to boronic acids than that of the diol group, and thus degrades the PMBV/PVA hydrogel.23,30,31 There are no matrix metalloproteinase (MMP) sensitive segments in the PMBV/PVA hydrogel, so the PMBV/PVA hydrogel cannot be destroyed by MMPs secreted by cells. Therefore, it is impossible for the cells to degrade the hydrogels and subsequently enhance the permeability of the hydrogel. Actually, it was found that the cells embedded in the PMBV/PVA hydrogel were rounded and they could not spread or migrate in the hydrogel.20,22,23,29–32
Effects of PTX on the cell proliferation-cycle distribution
Next, we demonstrated the applicability of our approach to control PTX diffusion within the hydrogel layers by changes in the thickness of the diffusion-controlling barrier. First, we determined the effects of PTX on the cell proliferation-cycle distribution in HeLa-Fucci cells at 12 h after stimulation. Fucci allows determination of the dynamics of live-cell proliferation during a cell cycle; G1 and S/G2/M phase cells expressing Fucci emit red and green fluorescence, respectively.28 At 12 h, significant G2/M arrest was observed (Fig. 6a and d). Besides, confirming the previous report,33 we also observed that abnormal red fluorescence appeared in addition to the original green fluorescence at the M phase. Cells collapsed several hours after the appearance of the abnormal red fluorescence, suggesting that the emergence of the abnormal red fluorescence is an early indicator of mitotic catastrophe. As shown in Fig. 6d, the percentage of (G2/M phase cells + abnormal cells) in the four groups in descending order were as follows: control group ≈ thin barrier group > intermediate barrier group > thick barrier group. No significant differences were observed between the control group and the thin barrier group. This shows that one can tune the biological activity by altering the number of PMBV/AWP layers composing the barrier. In order to further verify this barrier thickness dependent phenomenon, the cell viability of the above four groups was also characterized. Fig. 6b and c show the cell viability after 48 h of bioactive reagent stimulation. Indeed, there were significant differences among them. While one PMBV/AWP layer does not show a significant barrier effect compared with the control group, five layers efficiently hinder the effects of PTX. A barrier composed of three PMBV/AWP layers partially hinders the diffusion of PTX, leading to a cellular viability between that of one and five PMBV/AWP layers. In addition, we found a dose effect: higher embedded-PTX concentrations lead to higher apoptosis of HeLa-Fucci cells (see Fig. S2 in the ESI†).
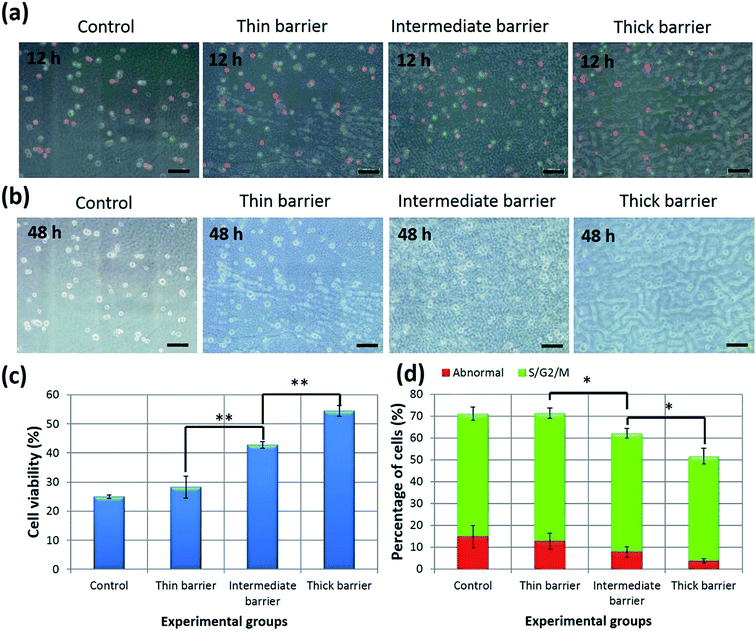 |
| Fig. 6 Regulation of cell cycle distribution and cellular viability by the thickness of the diffusion-controlling barrier. (a) Fluorescent images of HeLa-Fucci cells encapsulated within the top layer of a multilayer hydrogel is shown for the cell cycle distribution at 12 h; (b) bright-field images show the viability of HeLa-Fucci cells at 48 h; (c) cell viability is shown as the average ± SD (n = 3, α = 0.05, **p < 0.01); (d) cell cycle distribution, shown as the average ± SD (n = 3, α = 0.05, *p < 0.05) (scale bars: 100 μm). | |
These results can be explained based on the following mechanism: PTX can diffuse through a diffusion-controlling barrier and into the cell-containing gel and can come in contact with the cells. However, the amount of PTX in the cell-containing hydrogel is dependent on the thickness of the diffusion-controlling barrier and the initial concentration of the loaded bioactive reagent. With an increasing number of barrier layers, the diffusion of PTX became limited and induced a weaker stimulation effect to the HeLa-Fucci cells.
This strategy will now be extended to include the incorporation of customized multi-agents and cell-containing systems to mimic diffusion-controlled cell behavior in vivo. We envision that this system could serve as a powerful tool in tissue engineering and cell biology to address basic questions related to spatiotemporal signaling of biomolecules, such as morphogens. For example, the recapitulation of morphogen gradients within 3D structures and an examination of their influence on the fate of developing pluripotent stem cells, is possible.34,35
Conclusion
In this study, we have engineered sandwich structured multilayered hydrogels where a cell-containing gel layer was separated from the bioactive reagent reservoir layer by dual-crosslinked hydrogels. The multilayered hydrogels could be micropatterned three-dimensionally by a single mechanism or dual mechanisms to spatially control the hydrogel permeability on the micrometer scale and permit examination of the diffusion of the bioactive reagent on encapsulated cell behavior. By manipulating the thickness of the dual-crosslinked hydrogel, which worked as a diffusion-controlling barrier, the barrier effects were tunable. Thus, we have shown that PTX embedded in the reservoir layer decreased apoptosis in HeLa cells as the barrier thickness increased and the PTX concentration decreased. Overall, the permeability of the hydrogel system was introduced as a design parameter for regulating cellular behavior, and this multilayered hydrogel system provides a new platform for studying diffusion-dependent cell behavior for tissue engineering and regenerative medicine applications.
Acknowledgements
This work was supported by a Grant-in-Aid for Scientific Research on Innovative Areas “Nanomedicine Molecular Science” (no. 2306) from the Ministry of Education, Culture, Sports, Science and Technology of Japan. One of the authors (B.G.) would like to acknowledge the Chinese Scholarship Council (CSC) for financial support.
References
- L. G. Griffith and M. A. Swartz, Nat. Rev. Mol. Cell Biol., 2006, 7, 211–224 CrossRef CAS PubMed.
- H. K. Lau and K. L. Kiick, Biomacromolecules, 2015, 16, 28–42 CrossRef CAS PubMed.
- M. K. Gupta, J. R. Martin, T. A. Werfel, T. Shen, J. M. Page and C. L. Duvall, J. Am. Chem. Soc., 2014, 136, 14896–14902 CrossRef CAS PubMed.
- H. J. Lee, A. Sen, S. Bae, J. S. Lee and K. Webb, Acta Biomater., 2015, 14, 43–52 CrossRef CAS PubMed.
- S. Das, F. Pati, Y. J. Choi, G. Rijal, J. H. Shim, S. W. Kim, A. R. Ray, D. W. Cho and S. Ghosh, Acta Biomater., 2015, 11, 233–246 CrossRef CAS PubMed.
- T. Lühmann and H. Hall, Materials, 2009, 2, 1058–1083 CrossRef PubMed.
- C. Fan and D. A. Wang, Macromol. Biosci., 2015, 15, 535–545 CrossRef CAS PubMed.
- J. B. Gurdon and P. Y. Bourillot, Nature, 2001, 413, 797–803 CrossRef CAS PubMed.
- B. J. Peret and W. L. Murphy, Adv. Funct. Mater., 2008, 18, 3410–3417 CrossRef CAS PubMed.
- O. Jeon and E. Alsberg, Adv. Funct. Mater., 2013, 23, 4765–4775 CAS.
- K. A. Mosiewicz, L. Kolb, A. J. van der Vlies, M. M. Martino, P. S. Lienemann, J. A. Hubbell, M. Ehrbar and M. P. Lutolf, Nat. Mater., 2013, 12, 1072–1078 CrossRef CAS PubMed.
- G. Eng, B. W. Lee, H. Parsa, C. D. Chin, J. Schneider, G. Linkov, S. K. Sia and G. Vunjak-Novakovic, Proc. Natl. Acad. Sci. U. S. A., 2013, 110, 4551–4556 CrossRef CAS PubMed.
- M. P. Cuchiara, A. C. Allen, T. M. Chen, J. S. Miller and J. L. West, Biomaterials, 2010, 31, 5491–5497 CrossRef CAS PubMed.
- J. S. Miller, K. R. Stevens, M. T. Yang, B. M. Baker, D. H. Nguyen, D. M. Cohen, E. Toro, A. A. Chen, P. A. Galie, X. Yu, R. Chaturvedi, S. N. Bhatia and C. S. Chen, Nat. Mater., 2012, 11, 768–774 CrossRef CAS PubMed.
- P. T. Hammond, Mater. Today, 2012, 15, 196–206 CrossRef CAS.
- V. Gribova, R. Auzely-Velty and C. Picart, Chem. Mater., 2011, 24, 854–865 CrossRef PubMed.
- A. Shukla and B. Almeida, Wiley Interdiscip. Rev.: Nanomed. Nanobiotechnol., 2014, 6, 411–421 CrossRef CAS PubMed.
- L. Grossin, D. Cortial, B. Saulnier, O. Felix, A. Chassepot, G. Decher, P. Netter, P. Schaaf, P. Gillet, D. Mainard, J. C. Voegel and N. Benkirane-Jessel, Adv. Mater., 2009, 21, 650–655 CrossRef CAS PubMed.
- B. Gao, T. Konno and K. Ishihara, Colloids Surf., B, 2013, 108, 345–351 CrossRef CAS PubMed.
- B. Gao, T. Konno and K. Ishihara, Biomaterials, 2014, 35, 2181–2187 CrossRef CAS PubMed.
- X. Lin, K. Nishio, T. Konno and K. Ishihara, Biomaterials, 2012, 33, 8221–8227 CrossRef CAS PubMed.
- Y. Xu, K. Sato, K. Mawatari, T. Konno, K. Jang, K. Ishihara and T. Kitamori, Adv. Mater., 2010, 22, 3017–3021 CrossRef CAS PubMed.
- H. Oda, T. Konno and K. Ishihara, Biomaterials, 2013, 34, 5891–5896 CrossRef CAS PubMed.
- J. Choi, T. Konno, M. Takai and K. Ishihara, Biomaterials, 2009, 30, 5201–5208 CrossRef CAS PubMed.
- J. Choi, T. Konno, M. Takai and K. Ishihara, Biomaterials, 2012, 33, 954–961 CrossRef CAS PubMed.
- Y. Ito, M. Nogawa, M. Takeda and T. Shibuya, Biomaterials, 2005, 26, 211–216 CrossRef CAS PubMed.
- K. Ishihara, T. Ueda and N. Nakabayashi, Polym. J., 1990, 22, 355–360 CrossRef CAS.
- A. Sakaue-Sawano, H. Kurokawa, T. Morimura, A. Hanyu, H. Hama, H. Osawa, S. Kashiwagi, K. Fukami, T. Miyata, H. Miyoshi, T. Imamura, M. Ogawa, H. Masai and A. Miyawaki, Cell, 2008, 132, 487–498 CrossRef CAS PubMed.
- T. Aikawa, T. Konno and K. Ishihara, Soft Matter, 2013, 9, 4628–4634 RSC.
- B. Gao, T. Konno and K. Ishihara, Eur. Polym. J., 2015 DOI:10.1016/j.eurpolymj.2015.03.030.
- H. Oda, T. Konno and K. Ishihara, Biomaterials, 2015, 56, 86–91 CrossRef CAS PubMed.
- T. Konno and K. Ishihara, Biomaterials, 2007, 28, 1770–1777 CrossRef CAS PubMed.
- A. Honda-Uezono, A. Kaida, Y. Michi, K. Harada, Y. Hayashi, Y. Hayashi and M. Miura, Biochem. Biophys. Res. Commun., 2012, 428, 224–229 CrossRef CAS PubMed.
- O. Lieleg and K. Ribbeck, Trends Cell Biol., 2011, 21, 543–551 CrossRef CAS PubMed.
- N. Gjorevski, A. Ranga and M. P. Lutolf, Development, 2014, 141, 1794–1804 CrossRef CAS PubMed.
Footnote |
† Electronic supplementary information (ESI) available. See DOI: 10.1039/c5ra05299h |
|
This journal is © The Royal Society of Chemistry 2015 |
Click here to see how this site uses Cookies. View our privacy policy here.