DOI:
10.1039/C5RA05087A
(Paper)
RSC Adv., 2015,
5, 44173-44182
A novel and sensitive turn-on fluorescent biosensor for the determination of thioctic acid based on Cu2+-modulated N-acetyl-L-cysteine capped CdTe quantum dots†
Received
23rd March 2015
, Accepted 11th May 2015
First published on 12th May 2015
Abstract
A new fluorescence sensor for the determination of thioctic acid (TA) in aqueous media based on the recovered fluorescence of N-acetyl-L-cysteine capped CdTe quantum dots [NALC-CdTe QDs]–Cu2+ system was proposed. The fluorescence intensity of NALC-CdTe QDs was quenched by Cu2+ due to the binding of Cu2+ to NALC on the surface of the QDs. However, in the presence of TA, the fluorescence intensity of NALC-CdTe QDs was found to be efficiently recovered. Experimental results showed that the pH of the buffer solution and the concentration of Cu2+ affected the fluorescence intensity upon adding TA. Under the optimal conditions, the recovered fluorescence intensity was linearly proportional to the increasing TA concentration in the range 4–120 μg mL−1. In addition, among the other biologically relevant chemical species that were tested, only TA could turn on the fluorescence intensity suggesting that the [NALC-CdTe QDs]–Cu2+ system was a highly selective sensor for TA. Moreover, to further investigate the performance of the perfect analysis, the developed biosensor was applied to the determination of TA in its commercial samples and detected TA in the blood of a rabbit with the time extended.
1 Introduction
Thioctic acid (TA: molecular structure given in Fig. 1), also known as α-lipoic acid, is a naturally occurring disulfide compound synthesized in the mitochondria. It is also a potent lipophilic antioxidant with the ability to scavenge free radicals.1 TA was first isolated by Reed and coworkers as an acetate-replacing factor.2,3 It is abundant in the human diet and mostly from animal sources, and also a small amount from fruits and vegetables.4 In Europe, TA has been prescribed for decades for the treatment of diabetic neuropathy. Therapeutic doses of TA for humans range from 200 to 1800 mg d−1, with no significant adverse effects reported at doses as high as 2400 mg d−1.5 Studies have demonstrated that supplementation with TA reduces blood pressure, reduces plasma triglycerides, and increases low-density lipoprotein (LDL) oxidation lag time.6–9 In diabetics, TA has been shown to be beneficial in preventing damage to the endothelium and controlling the progression of diabetic nephropathy.10,11 Additionally, it has been observed that TA improves glucose metabolism and insulin sensitivity in diabetic patients.12,13 Therefore, there is a strong incentive to develop a sensitive, reliable, and convenient approach for supplementing TA.
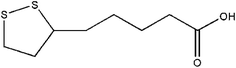 |
| Fig. 1 Molecular structure of TA. | |
Nowadays, various analytical methods have been developed for the inclusion of TA in pharmaceutical samples and dietary supplements. Those include high performance liquid chromatography (HPLC),14–17 and spectrophotometric methods.18–20 However, many of these assays are not readily adaptable to routine analysis because many of these methods are time-consuming, have low-sensitivity, require relatively expensive and complicated instruments.
It is well-known that semiconductor quantum dots (QDs) are attractive fluorescent labels due to their high quantum efficiency, photostability, and size-tunable optical properties, especially as compared to conventional organic dyes.21–23 In the past few years, QDs based fluorescence probe for the determination of small biological molecules,24 organic pollutants,25,26 and many metal ions.27–29 Nevertheless, most of the methods that used QDs as fluorescence probes work in a “turn-off” mode, and their selectivity is still a challenge. As we all know, the reactions between the surface of QDs and the metal ions and small molecules are not specific enough and many other species can also affect the surface of QDs in the turn-off mode. Therefore, recently, several metal ions functionalized QDs have been designed and provided a “turn-on” mode.30–35 As far as we know, the detection of TA using QDs as the fluorescence turn-on sensor has not been reported.
In this work, we used Cu2+ functionalized QDs as a fluorescence turn-on sensor for the determination of TA. Firstly, the fluorescence intensity of N-acetyl-L-cysteine capped CdTe QDs was quenched by Cu2+, and the fluorescence intensity of this system was then selectively recovered by TA as a result of high Cu2+–TA chelation. In addition, we also discussed the selectivity of the fluorescence turn-on sensor for other biologically relevant chemical species. Moreover, this new sensor had been applied to the determination of TA in its commercial samples and its blood concentration in the blood of rabbit with the time is extended.
2 Reagents and apparatus
All reagents used were of analytical grade and used without further purification. The main chemical reagents (and their sources): CdCl2·2.5H2O (Shanghai Chemicals Reagent Co., Shanghai, China), Te powder (Sinopharm Chemical Reagent Co., Shanghai, China), NaBH4 (Tianjin Huanwei Fine Chemical Co., Tianjin, China), N-acetyl-L-cysteine (NALC, Sinopharm Checal Reagent Co., Shanghai, China), thioctic acid (TA, Sinopharm Chemical Reagent Co., Shanghai, China). PBS buffer solutions with different pH were prepared by mixing 1/30 mol L−1 Na2HPO4 and 1/30 mol L−1 KH2PO4 in different proportions.
A Hitachi F-2500 spectrofluorophotometer (Hitachi Company, Tokyo, Japan) was used to record the fluorescence spectra and resonance Rayleigh scattering (RRS) spectra. A UV-2450 spectrophotometer (Tianmei Corporation, Shanghai, China) was applied to record the absorption spectra. Hitachi-600 transmission electron microscopy (TEM, Hitachi Company, Japan). A PHS-3C pH meter (Leici, Shanghai, China) was used to adjust the pH values of the aqueous solutions.
3 Methods
3.1 Synthesis of NALC-CdTe quantum dots
N-acetyl-L-cysteine (NALC) capped CdTe quantum dots (QDs) were prepared in aqueous solution using the method described previously36 with modification. Under N2 atmosphere and magnetic stirring, tellurium powder (0.0255 g) was reacted with excessive sodium borohydride in deionized water to produce the colorless solution of sodium hydrogen telluride (NaHTe).
CdCl2·2.5H2O (0.0488 g) and NALC (0.0574 g) were dissolved in 150 mL deionized water. Under magnetic stirring, the pH of the mixture was adjusted to 11.45 by using the dropwise addition of NaOH solution (1 mol L−1). The solution was deaerated by N2 bubbling for about 30 min. Under stirring, the H2Te gas generated by the reaction of the solution of NaHTe with diluted H2SO4 (0.5 mol L−1) was passed through the oxygen-free Cd2+ solution together with a slow nitrogen flow. The resulting solution mixture was then heated to 369 K and refluxed under nitrogen for 1 h, the salmon pink CdTe solution was obtained. The concentration of quantum dots was calculated using the original cadmium source and found to be 1.425 × 10−3 mol L−1. The quantum dots were stored at 4 °C. No precipitation was observed over a one month period.
3.2 Fluorescence quenching of NALC-CdTe QDs by Cu2+
In order to develop the ideal fluorescence biosensor for TA, the following procedure was carried out. A stock solution of 50 μg mL−1 Cu2+ was prepared. Researchers added 0.6 mL of the above prepared NALC-capped CdTe QDs, 1 mL PBS buffer solution (pH 7.6), and an appropriate amount of Cu2+ stock solution to 10 mL calibrated test tubes. This solution was then diluted with deionized water to the mark and mixed with gentle shaking. The solution mixture was then incubated at room temperature for 10 min. The fluorescence intensity was measured at λex = 278 nm.
3.3 Fluorescence recovered by TA
To study the fluorescence recovery of the Cu2+ modulated NALC-CdTe QDs system in the presence of TA, the following procedure was carried out. A stock solution of TA (200 μg mL−1) was prepared through the dissolution of 0.02 g solid TA in 3 mL 0.2 mol L−1 NaOH and deionized water. A combination of 0.6 mL NALC-CdTe QDs, 1 mL PBS buffer solution (pH 7.6), 0.5 mL Cu2+ (50 μg mL−1) and different concentration of TA stock solution was added to a 10 mL calibrated test tube, then diluted with deionized water to the mark and mixed thoroughly with gentle shaking. The solution mixture was then incubated at room temperature for 10 min. The fluorescence intensity was measured at λex = 278 nm.
4 Results and discussion
4.1 Characterization of the synthesized NALC-CdTe QDs
We have discussed how TEM affects the morphology of the as-prepared NALC-CdTe QDs. In Fig. 2(a), we can see that the particles are monodisperse in the shape and the sizes were about 2–3 nm. Moreover, the UV-vis absorption (curve 1) and fluorescence emission spectra (curve 2) of as-prepared NALC-CdTe QDs were also shown in Fig. 2(b). The UV-vis absorption spectrum showed a strong excitonic absorption in the ultraviolet region, and the characteristic absorption peak was located at 526 nm. Similarly, the fluorescence emission spectrum further confirmed that NALC-CdTe QDs were nearly monodisperse and homogenous for its favorable symmetry, the observed fluorescence band centered at 553 nm (excitation 278 nm).
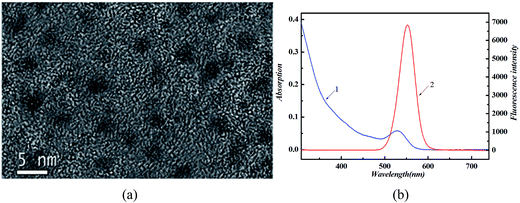 |
| Fig. 2 (a) TEM image; (b) UV-vis absorption (curve 1) and fluorescence (curve 2) spectra of NALC-CdTe QDs. | |
Particle size of NALC-CdTe QDs can be calculated by the following equation:37
|
D = (9.8127 × 10−7)λ3 − (1.7147 × 10−3)λ2 + 1.0064λ − 194.84
| (1) |
where
D stands for the diameter of NALC-CdTe QDs, and
λ is the wavelength of the first excitonic absorption of NALC-CdTe QDs. The result indicates that the particle size was about 2.92 nm (
λ = 526 nm), which is in accordance with the TEM image results.
4.2 Modulating the fluorescence intensity of NALC-CdTe QDs by Cu2+
As shown in Fig. 3, in the optimal experimental conditions, the fluorescence intensity of NALC-CdTe QDs was greatly quenched in the presence of Cu2+. Moreover, the quenching was accompanied by a shift of the wavelength emission maximum λmax (a red shift, from 553 to 565 nm) in the NALC-CdTe QDs spectrum. The red shift that can reasonably be attributed to an increased in polarity in the vicinity of the NALC-capped CdTe QDs as a result of the formation of a NALC-capped CdTe QDs–Cu2+ complex.38 Therefore, this quenching may be result from the coordination of Cu2+ to the ethanoyl group of the capping molecule on the surface of CdTe QDs.39 Therefore, the fluorescence quenching by Cu2+ may stem from the facilitation of the recombination of nonradiative e−/h+ recombination on the surface of NALC-CdTe QDs through an effective electron transfer process between surface ethanoyl groups and Cu2+,40 as shown in Scheme 1.
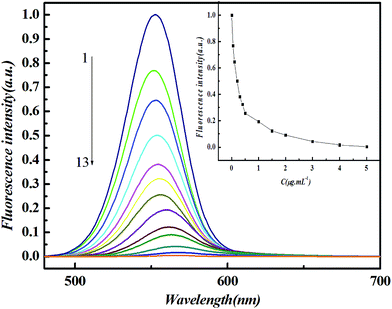 |
| Fig. 3 Fluorescence spectra of NALC-CdTe QDs in the presence of Cu2+ in 1 mL PBS buffer solution at pH = 7.6; the inset shows the linear of the quenched fluorescence intensity of QDs against the concentration of Cu2+. | |
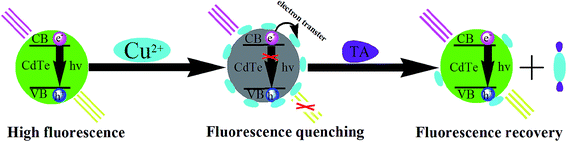 |
| Scheme 1 Schematic illustration for the signal transduction mechanism for the developed turn-on fluorescent biosensor for TA based on Cu2+ modulated NALC-capped CdTe QDs. | |
As a quenching reaction, there are usually two quenching mechanisms for fluorescence emission from QDs, which are either dynamic quenching or static quenching. To our knowledge, the well-known Stern–Volmer equation is the best way to demonstrate the quenching behavior of a quencher on the fluorescence of QDs.41
In the expression, F0 and F are the fluorescence intensity of NALC-CdTe QDs in the absence and presence of a quencher (Cu2+), respectively; [Q] is the concentration of the quencher. Ksv is the Stern–Volmer quenching constant, which defines the quenching efficiency of the quencher. As shown in Fig. 4, the linear plots for the NALC-CdTe QDs–Cu2+ solution system at three different temperatures (277 K, 295 K and 306 K) were investigated. The Ksv value of the NALC-CdTe QDs–Cu2+ solution system at three different temperatures were calculated according to eqn (2) and are listed in Table 1. It can be seen that the quenching constants decreased with the rise of temperature, which indicates that the probable quenching mechanism is the formation of NALC-CdTe QDs–Cu2+ complex (static quenching) rather than by dynamic quenching.42
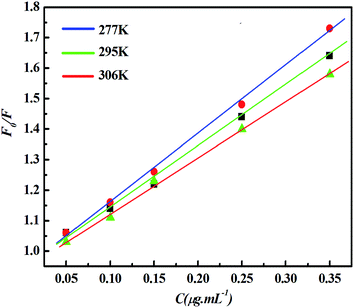 |
| Fig. 4 Stern–Volmer curves for the NALC-CdTe QDs–Cu2+ solution system at three different temperatures (NALC-CdTe QDs, 8.55 × 10−5 mol L−1; PBS buffer solution, 1.0 mL, pH = 7.6). | |
Table 1 Stern–Volmer quenching constants for the interaction of NALC-CdTe QDs with Cu2+ at three different temperatures
Temperature (K) |
Sterm–Volmer linear equation |
Ksv (L mol−1) |
Ra |
S.D.b |
R is the correlation coefficient. S.D. is the standard deviation for the Ksv values. |
306 |
F0/F = 0.9379 + 1.181 × 105[Q] |
1.181 × 105 |
0.9990 |
0.01160 |
295 |
F0/F = 0.9462 + 1.258 × 105[Q] |
1.258 × 105 |
0.9984 |
0.01561 |
277 |
F0/F = 0.9367 + 1.427 × 105[Q] |
1.427 × 105 |
0.9990 |
0.01449 |
As we know, in dynamic quenching, charge transfer occurs and the fluorescence is quenched when the electron acceptor collides with the excited fluorophore. The collision between the quencher and the fluorophore affects only the excited state of the fluorophore, on this account, no changes in the absorption spectrum are expected. In contrast, in static quenching, the absorption spectra of the fluorophore will be perturbed during the formation of ground–state complex.43 Therefore, by careful examination of the absorption spectrum, one can attempt to distinguish static and dynamic quenching. The absorption spectra of QDs in solution, of Cu2+ in solution, and of QDs–Cu2+ in solution are shown in Fig. 5. In comparison with NALC-CdTe QDs, the absorption spectrum of QDs in the presence of Cu2+ was higher, a bit flatter and with an observed red shift of 3 nm was observed, which implies changes in size or surface properties of the QDs. Namely, the quenching type is static quenching.
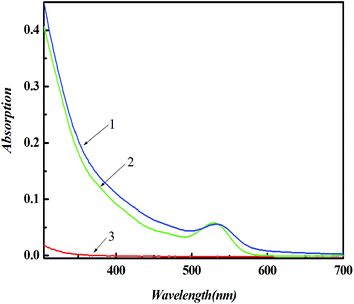 |
| Fig. 5 UV-vis absorption spectra of (1) mixture solution system (NALC-CdTe QDs and Cu2+), (2) NALC-CdTe QDs, (3) Cu2+ (with distilled water as the reference). | |
In order to further prove our conjecture, we have studied the RRS spectra of the NALC-capped CdTe QDs–Cu2+ system. As can be seen from Fig. 6, the RRS intensities of NALC-capped CdTe QDs and Cu2+ (curves a and b) are very weak. Whereas, when the QDs and Cu2+ were mixed together, the RRS intensity of the system significantly enhanced and the maximum peak was located at 370 nm. Then, we have investigated the effect of ionic strength on the RRS intensity of NALC-capped CdTe QDs–Cu2+ system by adding NaCl solution. The results shown that an increase in the concentration of NaCl increase, the RRS intensity of the system would decrease. With this, it could be speculated that electrostatic attraction played an important role in the reaction of NALC-capped CdTe QDs and Cu2+.
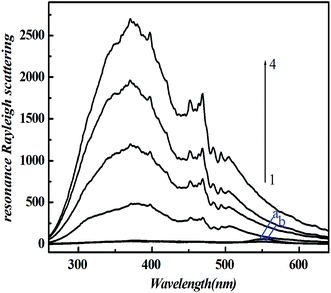 |
| Fig. 6 RRS spectra of NALC-capped CdTe QDs in the presence of Cu2+ in pH 7.6 PBS buffer solution, the concentration of Cu2+ (curve 1–4): 0.5, 1.0, 1.5, and 2.0 μg mL−1; NALC-capped CdTe QDs: 8.55 × 10−5 mol L−1 (curve a: 8.55 × 10−5 mol L−1 NALC-capped CdTe QDs; curve b: 1.5 μg mL−1 Cu2+). | |
4.3 Optimization of analytical conditions
In comparison with the fluorescence “turn-off” sensors, the fluorescence “turn-on” modes seem to be more preferable due to the reduction of the chance of false positives. Namely, the fluorescence “turn-on” sensing systems have better selectivity than “turn-off” sensors.44,45 As we have described before, the fluorescence recovery of NALC-CdTe QDs and Cu2+ system could be used as an optical probe for detecting TA. In order to obtain the best sensing sensitivity, we have investigated various possible parameters that affected the detection sensitivity.
4.3.1 Effect of pH. At first, the pH of the solution strongly affected the fluorescence quenching of Cu2+ toward the NALC-CdTe QDs. Then, the pH of the solution could also have an effect on the fluorescence restoration ability of TA. In this work, we used PBS as the buffer solution. As shown in Fig. 7(a), the pH of the solution influenced the fluorescence quenching ability of Cu2+ to CdTe QDs significantly, the maximum value of F0 − F was obtained when the pH value was 7.6 (F0 and F were the fluorescence intensities of the aqueous CdTe QDs without and with a given concentration of Cu2+). Therefore, the optimal pH of QDs–Cu2+ system was 7.6. More importantly, we must seek out the optimum pH in the recovery system. In Fig. 7(b), we can see that the maximum fluorescence recovery (ΔF = F1 − F, F and F1 were the fluorescence intensities of the aqueous CdTe QDs–Cu2+ system without and with a given concentration of TA) of NALC-CdTe QDs–Cu2+ system after the addition of TA was obtained at pH 7.6, which fitted well with the optimum pH (7.6) of QDs–Cu2+ system. Hence, we chose PBS (7.6) as the buffer solution in this work.
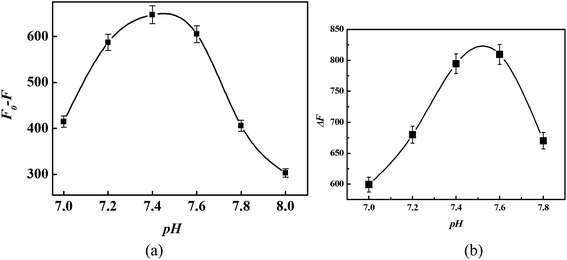 |
| Fig. 7 (a) Effect of acidity on the decrease of fluorescence intensity of NALC-CdTe QDs after the addition of Cu2+ in the PBS buffer solution (NALC-CdTe QDs: 8.55 × 10−5 mol L−1; Cu2+, 0.05 μg mL−1); (b) effect of acidity on the recovery of fluorescence intensity of NALC-CdTe QDs and Cu2+ system after the addition of TA in the PBS buffer solution (NALC-CdTe QDs, 8.55 × 10−5 mol.L−1; Cu2+, 2.5 μg mL−1; TA, 60 μg mL−1). | |
4.3.2 Effect of the concentration of NALC-CdTe QDs. We have investigated the influence of the concentration of NALC-CdTe QDs on the fluorescence intensity of NALC-CdTe–Cu2+ system. In this work, we kept the concentration of Cu2+ and pH value invariable while changing the concentration of NALC-CdTe QDs. The results indicated that the optimum amount of NALC-CdTe QDs was 8.55 × 10−5 mol L−1 (Fig. S3(a)†).
4.3.3 Effect of reaction time. The influence of reaction time on the fluorescence intensity of NALC-CdTe QDs, Cu2+ and TA system has been studied. The results revealed that the reaction finished after 10 min and the fluorescence intensity of the ternary system was stable within 2 h (Fig. S3(b)†). Therefore, in the following experiment, we measured the spectra of this system after 10 min.
4.3.4 Effect of the concentration of Cu2+. The concentration of Cu2+ in the ternary system was also found to be an essentially important factor affecting the fluorescence recovery by TA. To our knowledge, if the amount of Cu2+ in the solution were excessive, some TA would react with free Cu2+. Therefore, the remainder TA reacting with Cu2+ on the surface of QDs would decrease. On the other hand, if the solution contained an insufficient amount of Cu2+, the working range and sensitivity of the sensor would be limited. As shown in Fig. 3, with the increase of the concentration of Cu2+, the fluorescence intensity of QDs–Cu2+ system decreased. The inset of Fig. 3 shown that when the Cu2+ was at a lower concentration, the fluorescence intensity decreased greatly, but with the increase of the concentration of Cu2+, the extent of the decrease in fluorescence intensity diminished. Therefore, considering the above discussion, we chose 2.5 μg mL−1 as the optimum concentration in the following experiment.
4.4 Fluorescence detection of TA with Cu2+ modulated NALC-CdTe QDs
Based on the primary analysis above, the fluorescence intensity of NALC-CdTe QDs quenched greatly after adding Cu2+. Therefore, a new fluorescence “turn-on” sensor that used Cu2+-modulated NALC-CdTe QDs was obtained in our experiment to detect TA. As shown in Fig. 8(a), the fluorescence intensity of the sensor enhanced with an increase of TA concentration in a certain range. As demonstrated in Fig. 8(b), a linear calibration plot of the fluorescence intensity (F1) against the concentration of TA was observed in the range of 4–120 μg mL−1 with a correlation coefficient of 0.9975 and a linear regression equation of F1 = 445.29 + 6.27C (where C is the concentration of TA in μg mL−1). The detection limit of 14 ng mL−1 for TA was determined by using 3σ/K, where σ was the standard deviation of eleven replicate measurements of the fluorescence intensity of the blank samples and K was the slope of the calibration plot. These results showed the sensitivity of using this fluorescence sensing system for TA determination in aqueous solution. Fig. 9 exhibits the light observed from three different systems: (a) NALC-CdTe QDs, (b) [NALC-CdTe QDs]–Cu2+ and (c) [NALC-CdTe QDs]–Cu2+ after adding TA. The original QDs emitted the fluorescent light after UV illumination, which was turned off upon adding Cu2+. After adding TA into the [NALC-CdTe QDs]–Cu2+ solution, the emission light could be turned on again with a more shallow color compared with the original QDs. This observation corresponds well with the partial recovery of the spectrum from that of the original QDs obtained by the spectrofluorometer.
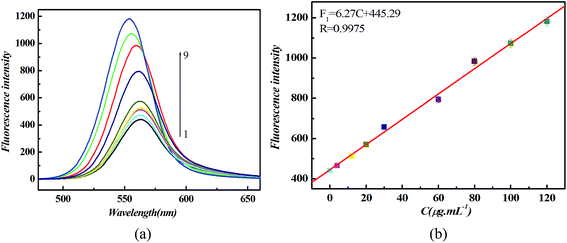 |
| Fig. 8 (a) Fluorescence spectra of NALC-CdTe QDs with the addition of 2.5 μg mL−1 of Cu2+ recover due to introducing TA in the concentration range of 0 to 120 μg mL−1; (b) the liner calibration plot of the fluorescence intensity against the concentration of TA. Concentration of NALC-CdTe QDs: 8.55 × 10−5 mol L−1. Concentration of TA: 0.0, 4.0, 12.0, 20.0, 30.0, 60.0, 80.0, 100.0, 120.0 μg mL−1, respectively. PBS buffer solution, 1.0 mL, pH = 7.6. | |
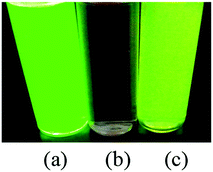 |
| Fig. 9 Photos under UV illumination, NALC-CdTe QDs (a), [NALC-CdTe QDs]–Cu2+ before (b) and after (c) adding TA (NALC-CdTe QDs, 8.55 × 10−5 mol L−1; Cu2+, 2.5 μg mL−1; TA, 60 μg mL−1). | |
In this work, in order to explore the interaction of TA with the proposed “turn-on” fluorescent sensor, we have investigated the reaction of TA with NALC-CdTe QDs. As we can seen from Fig. 10(a), the fluorescence emission of NALC-CdTe QDs was not influenced by the addition of TA (varied amounts of TA added in the same concentration of [NALC-CdTe QDs]–Cu2+ solution: 0.0, 4.0, 12.0, 20.0, 30.0, 60.0, 80.0, 100.0, 120.0 μg mL−1, respectively). These results showed that TA had no obvious influence on the fluorescence of NALC-CdTe QDs, and the fluorescence intensity recovery caused by the addition of TA result from the interaction of TA with Cu2+. To further prove this hypothesis, the absorption spectrums of NALC-CdTe QDs and [NALC-CdTe QDs]–Cu2+ before and after adding of TA were measured in 1 mL PBS buffer solution at pH = 7.6. As illustrated in Fig. 10(b), the curve (1), (2) and (3) were the absorption spectrum of [NALC-CdTe QDs]–Cu2+ after adding of TA, Cu2+ modulated NALC-CdTe QDs and NALC-CdTe QDs, respectively. It was observed that the addition of Cu2+ to the NALC-CdTe QDs solution induced the characteristic absorption peak of QDs at 526 nm and had a red-shift behavior, which we have discussed before as having been caused by the formation of NALC-CdTe QDs–Cu2+ complex. What is noteworthy is that after the addition of TA in [NALC-CdTe QDs]–Cu2+ solution system, a hypochromatic shift appeared. Compared with the range of the red-shift, the blue-shift was shorter. These results corresponds well with the partial recovery of the fluorescence “turn-on” sensor. Therefore, the result of fluorescence recovery of [NALC-CdTe QDs]–Cu2+ solution was caused by the interaction of Cu2+ with TA. To our knowledge, Cu2+ could react with TA through chelation.46–48 In this work, the added TA chelated with Cu2+ on the surface of NALC-CdTe QDs and lead to the fluorescence recovery of QDs, whose fluorescence was quenched by Cu2+. The designed turn-on fluorescent biosensor worked in principles as exhibited in Scheme 1.
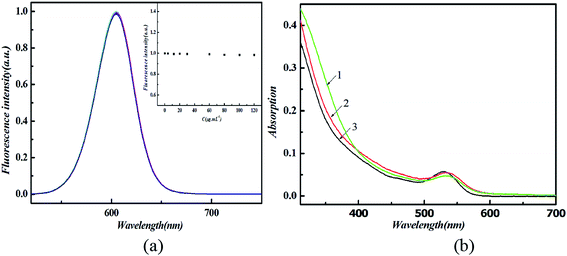 |
| Fig. 10 (a)The effect of TA on the fluorescence intensity of NALC-CdTe QDs in 1 mL PBS buffer solution at pH = 7.6 (NALC-CdTe QDs: 8.55 × 10−5 mol L−1; TA: 0.0, 4.0, 12.0, 20.0, 30.0, 60.0, 80.0, 100.0, 120.0 μg mL−1, respectively); (b) UV-vis absorption spectra of (1) [NALC-CdTe QDs]–Cu2+ after adding of TA, (2) Cu2+ modulated NALC-CdTe QDs, (3) NALC-CdTe QDs (NALC-CdTe QDs, 8.55 × 10−5 mol L−1; Cu2+, 2.5 μg mL−1; TA, 80 μg mL−1; PBS buffer solution, 1 mL, pH = 7.6). | |
4.5 Selectivity of the proposed turn-on fluorescent sensor for TA
Generally, selectivity is a crucial characteristic for most sensors. In this work, in order to evaluate the selectivity of the proposed turn-on fluorescent biosensor for TA, which has carboxylate radical, a series of interferential experiments was carried out. We compared the responses of Cu2+-modulated NALC-capped CdTe QDs to a variety of biologically relevant chemical species such as some anions, amino acids, purine, HSA and BSA. As seen from Fig. 11, only TA was able to turn on the fluorescence intensity of the [NALC-CdTe QDs]–Cu2+ probe. On the other hand, for the other tested anions, a slight recovery of the fluorescence intensity of the sensor was found. Compared with turn-off sensor, the selectivity of this sensor is better, due to the fact that the operation in turn-off followed the turn-on signal strategy, providing double sources of the selectivity. At first, the selectivity benefitted from the selective quenching by Cu2+. Then, most importantly, the selectivity was obtained from the strong Cu2+–TA complex which could discriminate between TA and other anions. These results suggested that the proposed sensor platform provided good selectivity toward TA by the fluorescence turn-on mechanism.
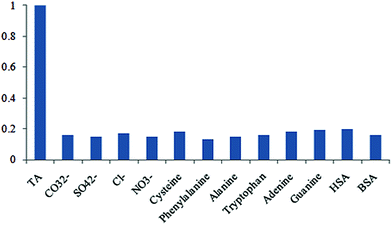 |
| Fig. 11 Fluorescence turn-on selectivity to various anions. | |
4.6 Application of the proposed turn-on fluorescent sensor
4.6.1 Detection of TA in commercial tablets. To confirm the feasibility of the proposed “off-on” fluorescent biosensor for real sample determination, the present biosensor was applied to determine TA in commercial pharmaceutical dosage forms (thioctic acid tablets). Grinding 10 medicinal tablets (0.1 g per tablet) of TA into powder and dissolved in ethanol, then filtrated and transferred it in a 100 mL calibrated flask, diluted to the mark. Transfer 10 mL of this solution to a 500 mL volumetric flask, diluted with water to volume, and mixed thoroughly. Finally, 0.4, 0.6, 1.0 mL of the solution was taken to determine the content of TA according to the general procedure, respectively. The results showed that the average content of TA from replicate measurements (RSD, 2.58%, n = 3) was 0.101 g per tablet, which was agreed well with the declared content (0.10 g per tablet), and confirmed the accuracy of this proposed method. Recovery experiments were performed by using the standard addition method. Complete results of this analysis were given in Table 2. It showed an acceptable recovery in the range 98.9–102.3%, indicating that the determination of TA using the proposed “off-on” fluorescent probe was sensitive and reliable.
Table 2 Results of real sample analysis indicating percentage recovery
Sample |
Original found (μg mL−1) |
Added (μg mL−1) |
Total found (μg mL−1) |
R.S.D. (%) (n = 5) |
Recovery (%) |
1 |
20.00 |
8.00 |
28.64 |
2.03 |
102.3 |
2 |
20.00 |
12.00 |
31.65 |
2.35 |
98.9 |
3 |
20.00 |
20.00 |
39.68 |
1.96 |
99.2 |
4.6.2 Control experiment for detection of blood drug (TA) concentration in living rabbits vivo and in vitro plasma. To further investigate the feasibility of this proposed “off-on” fluorescent sensor in vivo rabbits and in their vitro plasma, we first injected thioctic Acid Injection into the vein of the rabbit, and then drew blood from the rabbit at different times. A 3 mL aliquot of fresh blood and appropriate amount of heparin were mixed thoroughly and centrifuged at 5000 rpm for 10 min the supernatant fluid was added 2 mL trichloroacetic acid, then mixed thoroughly and centrifuged at 5000 rpm for 5 min. Finally, 0.2 mL the supernatant fluid was pipette into a 10 mL volumetric flask to determine the content of TA according to the general procedure). We then detected the TA concentration in vivo vein blood; the results are shown in Table 3. After 1 h, the concentration of TA in the rabbit blood was high. With the passage of time, however, the concentration of the drug in the blood gradually decreased; after 8 h, we could detect any TA in the blood of rabbit. In order to confirm the detected signal resulted from TA, we drew blood from the rabbit before injecting thioctic Acid Injection with “off-on” probe to detect. The result showed that the blood had no signal. Furthermore, we also determined TA in the plasma of rabbits in vitro. An appropriate amount of thioctic Acid Injection was added to 1 mL of the blood plasma. The method in this work was then used to detect the concentration of TA. The results are shown in Table 4.
Table 3 Results for the determination of TA in the blood of rabbit in different time after injected TA
Sample |
Injected g |
Found (1 h) μg mL−1 (n = 3) |
Found (3 h) μg mL−1 (n = 3) |
Found (5 h) μg mL−1 (n = 3) |
Found (8 h) μg mL−1 (n = 3) |
1 |
0.05 |
106 |
50 |
19 |
0 |
2 |
0.05 |
103 |
52 |
17 |
0 |
3 |
0.05 |
105 |
49 |
20 |
0 |
Table 4 Results for the determination of TA in fresh rabbit plasma samples, n = 5
Sample |
Found |
Added (μg mL−1) |
Found (μg mL−1) |
Recovery (n = 5, %) |
R.S.D (n = 5, %) |
ND: not detected. |
1 |
NDa |
25 |
24.8 |
99.2 |
1.87 |
2 |
NDa |
25 |
24.5 |
98.0 |
2.98 |
3 |
NDa |
25 |
25.4 |
101.6 |
2.50 |
5 Conclusion
Summarily, in this work, a turn-on fluorescent sensor was successfully developed for the determination of TA. Concerning the sensor, at first, the initial fluorescence of NALC-CdTe QDs was markly quenched, which was ascribed to the electrostatic association of Cu2+ on the surface of CdTe QDs resulting in the occurrence of a photoinduced electron transfer process. Then, upon addition of TA, the fluorescence enhancement behavior of Cu2+-modulated QDs was observed, which was attributed to TA induced Cu2+ to be dissociated from the surface of QDs, and then Cu2+ chelated with TA to form stable complex. The present sensor has demonstrated a good selectivity and high sensitivity for TA in commercial samples. Finally, the new sensor had been applied to the determination of TA its blood concentration in the blood of rabbit with the time is extended.
Acknowledgements
This work was supported by the two National Natural Science Foundation of China (no. 21175015; no. 21475014) and all authors here express their deep thanks.
References
- D. Ziegler, Thioctic acid for patients with symptomatic diabetic polyneuropathy: a critical review, Treat. Endocrinol., 2004, 3, 173–189 CrossRef CAS PubMed.
- L. J. Reed, B. G. DeBusk, I. C. Gunsalus and C. S. Hornberger Jr, Crystalline α-lipoic acid: a catalytic agent associated with pyrnvate dehydrogenase, Science, 1951, 114, 93–94 CAS.
- L. J. Reed, The chemistry and function of lipoic acids, Adv. Enzymol. Relat. Subj. Biochem., 1957, 18, 319–347 CAS.
- Y. J. Suzuki, M. Tsuchiya and L. Packer, Thioctic acid and dihydrolipoic acid are novel antioxidants which interact with reactive oxygen species, Free Radical Res. Commun., 1991, 15, 255–263 CrossRef CAS.
- R. A. Passwater, Lipoic Acid: The Metabolic Antioxidant, Keats Publishing Inc, New Canaan, CT, 1995 Search PubMed.
- S. Sola, M. Q. Mir and F. A. Cheema, et al., Irbesartan and lipoic acid improve endothelial function and reduce markers of inflammation in the metabolic syndrome: results of the Irbesartan and lipoic acid in endothelial dysfunction (ISLAND) study, Circulation, 2005, 111, 343–348 CrossRef CAS PubMed.
- A. Mahmud and J. Feely, Effect of angiotensin II receptor blockade on arterial stiffness: beyond blood pressure reduction, Am. J. Hypertens., 2002, 15, 1092–1095 CrossRef CAS.
- J. Ambrose, D. G. Pribnow, G. D. Giraud, K. D. Perkins, L. Muldoon and B. H. Greenberg, Angiotensin type 1 receptor antagonism with irbesartan inhibits ventricular hypertrophy and improves diastolic function in the remodeling post-myocardial infarction ventricle, J. Cardiovasc. Pharmacol., 1999, 33, 433–439 CrossRef CAS PubMed.
- S. Mankad, T. A. d'Amato and N. Reichek, et al., Combined angiotensin II receptor antagonism and angiotensin-converting enzyme inhibition further attenuates postinfarction left ventricular remodeling, Circulation, 2001, 103, 2845–2850 CrossRef CAS.
- M. C. Corretti, T. J. Anderson and E. J. Benjamin, et al., Guidelines for the ultrasound assessment of endothelial-dependent flow-mediated vasodilation of the brachial artery: a report of the international brachial artery reactivity task force, J. Am. Coll. Cardiol., 2002, 39, 257–265 CrossRef.
- R. Ross, Atherosclerosis is an inflammatory disease, Am. Heart J., 1999, 138, S419–S420 CrossRef CAS.
- P. Libby, P. M. Ridker and A. Maseri, Inflammation and atherosclerosis, Circulation, 2002, 105, 1135–1143 CrossRef CAS.
- R. Candido, K. A. Jandeleit-Dahm and Z. Cao, et al., Prevention of accelerated atherosclerosis by angiotensin-converting enzyme inhibition in diabetic apolipoprotein E-deficient mice, Circulation, 2002, 106, 246–253 CrossRef CAS.
- H. Salem, Chromatographia, LC simultaneous determination of thioctic acid, benfotiamine and cyanocobalamin in thiotacid compound capsules, Chromatographia, 2010, 72, 327–330 CAS.
- H. Y. Aboul-Eneina and H. Hoenena, Validated method for determination of α-lipoic acid in dietary supplement tablets by reversed phase liquid chromatography, J. Liq. Chromatogr. Relat. Technol., 2005, 27, 3029–3038 CrossRef.
- H. Kataoka, Chromatographic analysis of lipoic acid and related compounds, J. Chromatogr. B: Biomed. Sci. Appl., 1998, 717, 247–262 CrossRef CAS.
- A. I. Durrani, H. Schwartz, W. Schmid and G. Sontag, α-Lipoic acid in dietary supplements: development and comparison of HPLC–CEAD and HPLC–ESI-MS methods, J. Pharm. Biomed. Anal., 2007, 45, 694–699 CrossRef CAS PubMed.
- M. I. Walash, A. M. El-Brashy, M. S. Metwally and A. A. Abdelal, Spectrophotometric and kinetic determination of some sulphur containing drugs in bulk and drug formulations, Bull. Korean Chem. Soc., 2004, 25, 517–524 CrossRef CAS.
- N. El-Enany, F. Belal and M. Rizk, Spectrophotometric determination of thioctic acid in its dosage forms through complex formation with Pd(II), J. Chin. Chem. Soc., 2007, 54, 941–948 CrossRef CAS PubMed.
- Z. Koricanalc, M. Cakar, S. Tanaskovic and T. Jovanovic, Spectrophotometric determination of thioctic (α-lipoic) acid in water and pharmaceutical preparations, J. Serb. Chem. Soc., 2007, 72, 29–35 CrossRef.
- J. M. Costa-Fernańdez, R. Pereiro and A. Sanz-Medel, The use of luminescent quantum dots for optical sensing, Trends Anal. Chem., 2006, 25, 207–218 CrossRef PubMed.
- A. P. Alivisatos, W. W. Gu and C. Larabell, Annu. Rev. Biomed. Eng., 2005, 7, 55–76 CrossRef CAS PubMed.
- A. M. Smith and S. M. Nie, Semiconductor Nanocrystals: Structure, Properties, and Band Gap Engineering, Acc. Chem. Res., 2010, 43, 190–200 CrossRef CAS PubMed.
- Q. Ma, W. Yu, H. Huang and X. G. Su, Determination of L-tyrosine Based on Luminescence Quenching of Mn-Doped ZnSe Quantum Dots in Enzyme Catalysis System, J. Fluoresc., 2011, 21, 125–131 CrossRef CAS PubMed.
- C. A. Constantine, K. M. Gattás-Asfura, S. V. Mello, G. Crespo, V. Rastogi, T. Cheng, J. J. De Frank and R. M. Leblanc, Layer-by-Layer Films of Chitosan, Organophosphorus Hydrolase and Thioglycolic Acid-Capped CdSe Quantum Dots for the Detection of Paraoxon, J. Phys. Chem. B, 2003, 107, 13762–13764 CrossRef CAS.
- F. Qu and H. Li, Selective molecular recognition of polycyclic aromatic hydrocarbons using CdTe quantum dots with cyclodextrin as supramolecular nano-sensitizers in water, Sens. Actuators, B, 2009, 135, 499–505 CrossRef CAS PubMed.
- Y. Chen and Z. Rosenzweig, Luminescent CdS Quantum Dots as Selective Ion Probes, Anal. Chem., 2002, 74, 5132–5138 CrossRef CAS.
- Z. B. Shanga, Y. Wanga and W. J. Jin, Triethanolamine-capped CdSe quantum dots as fluorescent sensors for reciprocal recognition of mercury(II) and iodide in aqueous solution, Talanta, 2009, 78, 364–369 CrossRef PubMed.
- J. G. Liang, X. P. Ai, Z. K. He and D. W. Pang, Functionalized CdSe quantum dots as selective silver ion chemodosimete, Analyst, 2004, 129, 619–622 RSC.
- M. J. Ruedas-Rama and E. A. H. Hall, Azamacrocycle Activated Quantum Dot for Zinc Ion Detection, Anal. Chem., 2008, 80, 8260–8268 CrossRef CAS PubMed.
- J. G. Liang, S. Huang, D. Y. Zeng, Z. K. He, X. H. Ji, X. P. Ai and H. X. Yang, CdSe quantum dots as luminescent probes for spironolactone determination, Talanta, 2006, 69, 126–130 CrossRef CAS PubMed.
- P. Wu and X.-P. Yan, Ni2+-modulated homocysteine-capped CdTe quantum dots as a turn-on photoluminescent sensor for detecting histidine in biological fluids, Biosens. Bioelectron., 2010, 26, 485–490 CrossRef CAS PubMed.
- B. Han, J. Yuan and E. Wang, Sensitive and Selective Sensor for Biothiols in the Cell Based on the Recovered Fluorescence of the CdTe Quantum Dots−Hg(II) System, Anal. Chem., 2009, 81, 5569–5573 CrossRef CAS PubMed.
- H. Xu, R. Miao, Z. Fang and X. Zhong, Quantum dot-based “turn-on” fluorescent probe for detection of zinc and cadmium ions in aqueous media, Anal. Chim. Acta, 2011, 687, 82–88 CrossRef CAS PubMed.
- Y. Z. Shen, S. P. Liu, J. Y. Yang, L. L. Wang, X. P. Tan and Y. Q. He, A novel and sensitive turn-on fluorescent biosensor for the DNA detection using Sm3+-modulated glutathione-capped CdTe quantum dots, Sens. Actuators, B, 2014, 199, 389–397 CrossRef CAS PubMed.
- Q. Xiao, S. Huang, W. Su, W. H. Chan and Y. Liu, Facile synthesis and characterization of highly fluorescent and biocompatible N-acetyl-L-cysteine capped CdTe/CdS/ZnS core/shell/shell quantum dots in aqueous phase, Nanotechnology, 2012, 23, 495717–495726 CrossRef PubMed.
- Y. L. Yu, L. R. Xu, J. Chen, H. Y. Gao, S. Wang, J. Fang and S. K. Xu, Hydrothermal synthesis of GSH–TGA co-capped CdTe quantum dots and their application in labeling colorectal cancer cells, Colloids Surf., B, 2012, 95, 247–253 CrossRef CAS PubMed.
- Y. J. Hu, Y. Wang, Y. Ou-Yang, J. Zhou and Y. Liu, Characterize the interaction between naringenin and bovine serum albumin using spectroscopic approach, J. Lumin., 2010, 130, 1394–1399 CrossRef CAS PubMed.
- A. G. Kumbhar and K. Kishore, Redox reactions of Cu(II)-amine complexes in aqueous solutions, Radiat. Phys. Chem., 2003, 66, 275–280 CrossRef CAS.
- J. Chen, Y. C. Gao, Z. B. Xu, G. H. Wu, Y. C. Chen and C. Q. Zhu, A novel fluorescent array for mercury(II) ion in aqueous solution with functionalized cadmium selenide nanoclusters, Anal. Chim. Acta, 2006, 577, 77–84 CrossRef CAS PubMed.
- D. Zhao, W. H. Chan, Z. K. He and T. Qiu, Quantum dot ruthenium complex dyads: recognition of double-strand DNA through dual-color fluorescence detection, Anal. Chem., 2009, 81, 3537–3543 CrossRef CAS PubMed.
- Y. J. Hu, H. L. Yue, X. L. Li, S. S. Zhang, E. Tang and L. P. Zhang, Molecular spectroscopic studies on the interaction of morin with bovine serum albumin, J. Photochem. Photobiol., B, 2012, 112, 16–22 CrossRef CAS PubMed.
- C. D. Geddes, Optical halide sensing using fluorescence quenching: theory, simulations and applications-a review, Meas. Sci. Technol., 2001, 12, 53 CrossRef.
- R. Badugu, J. R. Lakowicz and C. D. Geddes, Enhanced Fluorescence Cyanide Detection at Physiologically Lethal Levels:
Reduced ICT-Based Signal Transduction, J. Am. Chem. Soc., 2005, 127, 3635–3641 CrossRef CAS PubMed. - G. L. Wang, H. J. Jiao, X. Y. Zhu, Y. M. Dong and Z. J. Li, Enhanced fluorescence sensing of melamine based on thioglycolic acid-capped CdS quantum dots, Talanta, 2012, 93, 398–403 CrossRef CAS PubMed.
- M. P. Miranda, R. del Rio, M. A. del Valle, M. Faundez and F. Armijo, Use of fluorine-doped tin oxide electrodes for lipoic acid determination in dietary supplements, J. Electroanal. Chem., 2012, 668, 1–6 CrossRef CAS PubMed.
- P. Ou, H. J. Tritschler and S. P. Wolef, Thioctic (lipoic) acid: a therapeutic metal-chelating antioxidant, Biochem. Pharmacol., 1995, 50, 123–126 CrossRef CAS.
- L. Packer, E. H. Witt and H. J. Tritschler, Alpha-lipoic acid as a biological antioxidant, Free Radical Biol. Med., 1995, 19, 227–250 CrossRef CAS.
Footnote |
† Electronic supplementary information (ESI) available. See DOI: 10.1039/c5ra05087a |
|
This journal is © The Royal Society of Chemistry 2015 |