DOI:
10.1039/C5RA04128G
(Paper)
RSC Adv., 2015,
5, 44165-44172
One-pot green synthesis of Ag/AgCl nanocube/reduced graphene oxide and its application to the simultaneous determination of hydroquinone and catechol
Received
9th March 2015
, Accepted 8th May 2015
First published on 8th May 2015
Abstract
Uniformly dispersed Ag/AgCl nanocubes (AgNC) were successfully obtained on reduced graphene oxide (rGO) through the simultaneous reduction of Ag+ and graphene oxide (GO) by chitosan in the presence of a little HCl. The AgCl acted as a seed. The obtained nanocomposite was characterized by X-ray diffraction (XRD), energy dispersive X-ray spectroscopy (EDS) and scanning electron microscopy (SEM). The as-synthesized AgNC/rGO was immobilized on the surface of a poly(5-amino-1,3,4-thiadiazole-2-thiol) (p-ATT) modified carbon fiber disk ultramicroelectrode (CFME) for the simultaneous determination of hydroquinone (HQ) and catechol (CT). This sensor showed a wide linear range of 0.08–1000 μmol L−1 for HQ and 1.5–900 μmol L−1 for CT in the presence of 30 μmol L−1 of the counterpart.
1. Introduction
Silver nanoparticles (AgNP) with unique properties1 have attracted great attention from chemists in various fields, such as optics,2 sensing3 and catalysis.4 However, well-dispersed AgNP are difficult to obtain without the use of stabilizers or surfactants because AgNP tend to agglomerate. To resolve the problem, different materials, including silicon nanowires,5 multi-walled carbon nanotubes,6 carbonaceous nanospheres7 and rGO8 have been employed to enhance the stability of AgNP. Among them, rGO has received considerable attention due to its strictly two-dimensional closely packed honeycomb lattice structure, high surface area (∼2600 m2 g−1), high chemical stability and unique electronic, mechanical properties.9–12 Many methods have been developed to prepare AgNP/rGO nanocomposites. However, the synthesis of AgNP/rGO nanocomposite is usually based on the reduction of preformed AgNP/GO hybrids or the decoration of rGO sheets with pre-synthesized AgNPs, which involve multiple steps and require complex manipulation.13,14 Asiri et al. demonstrated a one-pot hydrothermal reduction method for the preparation of AgNP/rGO hybrids.15 Sun et al. reported a rapid approach to prepare AgNP/rGO sheets using hydrazine hydrate as reducing agent.16 However, these strategies usually require high temperature (more than 100 °C) with high energy-consumption, or suffer from the use of poisonous and hazardous reductant, which may bring about environmental and health risks. Environmentally friendly, cost-effective, and one-step strategy toward rapid preparation of AgNP/rGO nanocomposite attracted attention. The shape-selective catalytic activity could be interpreted in terms of the different reaction performances induced by the specific crystal facets selectively exposed by an anisotropically shaped nanostructure, and also the amount of the catalytically active sites, such as corners, edges, steps, etc. Pan et al. anchored hexagonal Ag nanoplates to rGO to form novel Ag nanoplates/rGO nanocomposite.17 Jiang et al. prepared silver nanorod/reduced graphene oxide by the chemical-microwave reduced procedure.18 Chen et al. synthesized silver nanowire-decorated reduced graphene oxide nanosheets with the aid of trisodium citrate.19 Among various shapes, nanocubes have exhibited extremely high electrocatalytic activity due to its rich (1 0 0) facets.20 For example, Yang et al. reported an amperometric bienzyme glucose biosensor by co-immobilization HRP and GOx on the Ag nanocubes.21 Yang et al. developed an amperometric non-enzymatic glucose sensor by electrodepositing copper nanocubes onto vertically well-aligned multi-walled carbon nanotube arrays.22 To the best of our knowledge, no literatures are reported for the preparation of AgNC/rGO. Lu et al. synthesized four different morphologies of silver nanoproducts by altering the concentration of Na2S.23 Liu et al. facilely formulated AgCl nanocubes in terms of hybridizing GO.24 Based on these, we want to synthesize AgNC/rGO. GO works as a capping agent during the nanofabrication step. Chitosan is derivative of the polysaccharide chitin widely distributed in nature and a large number of free amino and hydroxyl groups in chitosan chains offering unique physicochemical properties. In this paper, taking advantage of the reducing ability of chitosan towards AgNO3
25 and GO,26 a green one-pot method for growing Ag/AgCl nanocubes on rGO is developed by simultaneous reduction of AgNO3 and GO with chitosan in the presence of little HCl.
Hydroquinone (HQ) and catechol (CT) are two important isomers of dihydroxybenzene, which are frequently used as industrial reagents in the production of rubber, dyes, plastics, pharmaceuticals and cosmetics. They widely exist in environment as a kind of important pollutant because they are toxic to humans and difficult to be degraded. What is more, due to their similar structures and properties, HQ and CT usually coexist in products and interfere with each other during their identification.27 Several methods have been exploited for the determination of HQ and CT, such as chemiluminescence,28 liquid chromatography,29 spectrophotometry,30 synchronous fluorescence technique31 and electrochemical methods.32 Among them, electrochemical methods have attracted more and more attentions due to the advantages of fast response, cheap instrument, low cost, simple operation, time-saving, high sensitivity and excellent selectivity. Various electrodes were used for the determination of HQ and CT such as glassy carbon electrode,33 platinum electrode34 and carbon electrode.35 However, they couldn't distinguish HQ and CT directly because HQ and CT have similar structures and properties. Zhang et al. developed boron-doped rGO modified glassy carbon electrode for the determination of HQ and CT.36 Wang et al. presented multiwall carbon nanotubes-poly(diallyldimethylammonium chloride)-rGO modified glassy carbon electrode for the simultaneous detection of HQ and CT.37 Kashanian described a method for construction of an electrochemical biosensor to detect CT based on covalent immobilization of laccase onto polyaniline electrodeposited onto a glassy carbon electrode.38 Li et al. fabricated an electrochemical sensor based on disulfides bridged β-cyclodextrin dimer-functionalized multi-walled carbon nanotube for simultaneous determination of 4-aminophenol, 4-chlorophenol and 4-nitrophenol.39 Srivastava et al. reported the synthesis of AgNP/rGO in the presence of sodium hydroxide, and AgNP/rGO based electrochemical sensor was fabricated for the simultaneous determination of ascorbic acid, dopamine, uric acid, and tryptophan.40 The application of AgNC/rGO and CFME for electrochemical sensors of HQ and CT has rarely been explored. In the present study, we find that CFME, which has excellent intrinsic characteristics such as temporal resolution, high current densities and reduced ohmic drop, can achieve their simultaneous determination on bare electrode. Conjugated polymers derive from heterocyclic compounds have emerged as promising materials for immobilizing nanomaterial due to their conducting nature and high stability. In this work, we electrodeposited ATT onto CFME for the first time. The –NH2 and –SH groups exposed to the p-ATT can facilitate the immobilizing of AgNC/rGO. Considering above, AgNC/rGO/p-ATT modified CFME was developed for the simultaneous and sensitive determination of HQ and CT, where the high electron transfer ability of p-ATT was combined with the unique electronic structure of AgNC/rGO. This sensor showed a good electrocatalytic performance to HQ and CT with wide linear range, low detection limit, good stability and reproducibility.
2. Experimental
2.1. Reagents and apparatus
Chitosan with a deacetylation degree of 90% was purchased from Sinopharm Chemical Regent Co. Ltd, used as received. AgNO3 was purchased from Tianjin Damao Chemical Factory. All other chemicals were of analytical grade from Shanghai Chemical Factory (Shanghai, China), and all solutions were prepared with distilled water.
The morphologies of the samples were observed using a scanning electron microscope (SEM, Hitachi S-4800) with energy dispersive X-ray spectrometry (EDS) mode. The crystal structures of the samples were examined by X-ray diffraction (XRD; D/max2550VB X-ray diffractometer, Rigaku).
Electrochemical measurements were performed on a CHI-920c workstation (CH Instruments). A Faraday cage was used to reduce electrical noise. A three-electrode configuration consisted of a carbon fiber disk ultramicroelectrode, a platinum wire, and saturated calomel electrode (SCE) as the working, the counter and the reference electrodes, respectively. All potentials in this work are referred to SCE as the reference.
2.2. Preparation of AgNC/rGO nanocomposite
GO was obtained by oxidizing graphite using an improved method published by Marcano et al.41 A stock solution of 1% chitosan was prepared by dissolving chitosan in 0.5 mol L−1 acetic acid solution. 4 mL AgNO3 solution (26 mmol L−1) was added to the stirring GO suspension (10 mL, 0.5 mg mL−1, pH = 6 adjust with HCl). Then, they were added to the chitosan solution (20 mL). Subsequently, the mixture was kept at 95 °C for 3 days. Upon the reaction, the suspension changed its color from light brown to dark, suggesting the formation of AgNC/rGO nanocomposite. The solid product was isolated by centrifugation.
2.3. Preparation of AgNC/rGO/p-ATT/CFME
CFME was fabricated according to the reported method with some modifications.42 A single carbon fiber (5 μm diameter) was inserted into the tip of the glass tube (1–2 mm diameter) and filled with epoxy resin. After drying, graphite and copper wire were inserted for electrical contact. The microelectrode surface was polished mechanically with graded alumina powder of different sizes (1, 0.3, 0.05 μm) on a polishing cloth. The response of polished electrodes was tested by voltammetry in 0.1 mol L−1 KCl containing 10 mmol L−1 K3[Fe(CN)6] at a scan rate of 10 mV s−1. Due to its well-established behavior, the voltammograms exhibited sigmoid profiles characteristic of the utilization of microelectrodes.43
Electropolymerization of ATT onto the CFME was carried out by using cyclic voltammetry. 15 successive potential sweeps were applied between −0.40 and +1.70 V at a scan rate of 50 mV s−1 in 0.10 mol L−1 H2SO4 containing 1.0 mmol L−1 ATT. The CV of electrochemical polymerized ATT was similar but lower ATT oxidation and reduction signal comparing with the earlier report.44 Then, the CFME was dipped for 30 min in 0.5 mg mL−1 AgNC/rGO solution and dried in air before use.
3. Results and discussion
3.1. Characterization of AgNC/rGO
Using chitosan as a reducing reagent to prepare AgNC/rGO is simple and can't introduce any environmental toxicity. The XRD pattern was used to identify the formation of AgNC/rGO (Fig. 1). Graphite powder shows a sharp peak at 26.4° with a typical interlayer spacing of 0.34 nm (curve a). The XRD pattern shows a sharp peak at 2θ of 9.6° (curve b), which can be ascribed to the characteristic diffraction peak of GO. It is corresponding to a layer-to-layer distance (d-spacing) of about 0.86 nm. This is larger than that of pristine graphite (0.34 nm), as a result of the introduction of oxygenated functional groups on carbon sheets. After being reduced by chitosan, the diffraction peaks at 2θ of 37.7 (1 1 1), 44.3 (2 0 0), 64.4 (2 2 0) and 77.3 (3 1 1) correspond to the cubic phase of Ag (JCPDS no. 65-2871). The intensity ratio of the (2 0 0) to (1 1 1) peak is 0.644, which is higher than the value (i.e., 0.4) detected in conventional powder samples (JCPDS card file no. 04-0783), indicating that the silver nanostructures are growing preferentially along the (1 0 0) direction and the sides of the silver cubes are bound by the enlarged (1 0 0) facets. The XRD result manifests the fcc silver crystal feature of the synthesized nanocubes.20 The peaks at 2θ of 27.6 (1 1 1), 32.4 (2 0 0), 46.1 (2 2 0), 54.8 (3 1 1) and 57.4 (2 2 2) can be assigned to the cubic phase of AgCl (JCPDS no. 31-1238) (curve c). The XRD result indicates the coexistence of Ag and AgCl in our nanocomposite. The low intensity for the diffraction peaks of AgCl may be due to the low content. No diffraction peak attributed to rGO is found. This is because the anchored AgNC disrupt the layered and ordered structure of rGO, and consequently lead to the disappearance of the reflection peak.
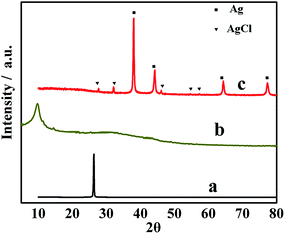 |
| Fig. 1 XRD patterns of the (a) graphite, (b) GO, and (c) AgNC/rGO composite. | |
In addition, the existence of both rGO and AgNC can be indicated from peaks of energy dispersive X-ray spectroscopy (EDS). The EDS spectrum suggests that nanocomposite consists of Ag, Cl, C, O and Si elements. The result shows that the nanocomposite is composed of Ag and AgCl with a molar ratio of 4.65
:
1 (Fig. 2), which is consistent with the result of XRD. The Si signal derives from the silicon chip. Reduction of GO with chitosan does not generate perfect graphene, and few oxygen-containing groups (OCGs) still remain in the rGO owing to the limited restoring ability of reductions.45 The residual OCGs have the opportunity to capture the amino and hydroxyl groups of chitosan via zwitterionic interaction and hydrogen bonding, by which chitosan noncovalently bond to the rGO and endows with dispersibility in water.46 Therefore, we had successfully synthesized AgNC/rGO.
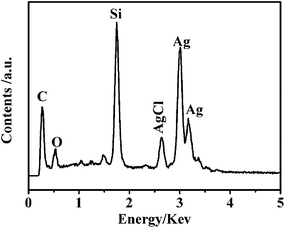 |
| Fig. 2 EDS spectrum of AgNC/rGO. | |
The morphologies of GO and AgNC/rGO are characterized by SEM. The SEM image of GO (Fig. 3A) depicts wrinkled structures, explicating the intrinsic GO morphology. The SEM image of the AgNC/rGO (Fig. 3B) reveals that large quantities of AgNC with the size of about 100 nm are attached on the silk-like surface of the rGO. The wrinkles appearing on the surface of the particles could illustrate the existence of rGO.
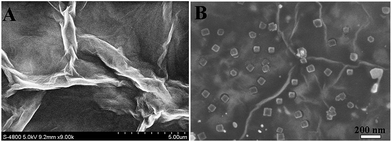 |
| Fig. 3 SEM images of the (A) GO, and (B) AgNC/rGO composite. | |
Fig. 4 shows the cyclic voltammograms of different electrodes in the 10.0 mmol L−1 K3[Fe(CN)6] containing 0.1 mol L−1 KCl. According to Tomes criterion for a reversible system, this potential difference (|E3/4 − E1/4|) should be 54.4/n mV at 298 K where n is the number of electrons transferred during the electrochemical process. Bare CFME showed that this potential difference is 46 mV. The value is close to the expected value, which indicated that reversible system was obtained on the bare CFME. Compared to the bare CFME, the peak current of p-ATT/CFME increased, which could be attributed to the excellent electrical conductivity and fast electron transfer rate of p-ATT. The peak current of K3[Fe(CN)6] at AgNC/rGO/CFME was higher than the peak current at the bare CFME, which was due to the good electrical conductivity and catalyst effect of AgNC/rGO. The AgNC/rGO/p-ATT/CFME showed the biggest peak current compared to bare CFME and p-ATT/CFME. The result could be attributed to the synergistic effects of p-ATT and AgNC/rGO, which improved the whole interfacial conductivity.
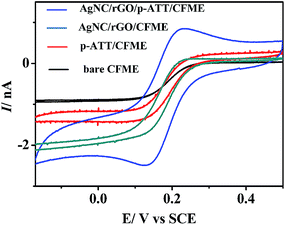 |
| Fig. 4 Cyclic voltammograms of 10.0 mmol L−1 K3[Fe(CN)6] containing 0.1 mol L−1 KCl at bare CFME, p-ATT/CFME, AgNC/rGO/CFME, and AgNC/rGO/p-ATT/CFME. | |
3.2. Electrochemical behaviors of HQ and CT at AgNC/rGO/p-ATT/CFME
Among the electroanalytical techniques for use with microelectrodes, square-wave voltammetry (SWV) has proved to be extremely sensitive for the detection of organic molecules.47 The electrocatalytic activity of the AgNC/rGO/p-ATT film was evaluated by the comparison of SWVs of 300 μmol L−1 HQ and CT in pH 5.0 acetate buffer recorded at four different working electrodes (Fig. 5). Two peaks are presented at potentials of 0.154 and 0.284 V, which correspond to the oxidation peaks of HQ and CT on bare CFME (curve a). The electron cloud density of HQ is lower than that of CT, so CT is harder to be oxidized than HQ. The resultant separation in two peak potentials (about 130 mV) is sufficient enough to achieve the simultaneous determination of HQ and CT in their mixtures. At the AgNC/rGO/CFME (curve b), the peaks current of HQ and CT are higher than that of the bare CFME, which should be due to the high surface area and excellent electrical conductivity of AgNC/rGO. At the p-ATT/CFME (curve c), intensive increases in peak currents of both HQ and CT are obtained. The catalytic effect is attributed to the following reasons: on the one hand, polymer film has the hydrophobic interaction with the aromatic part of analysts; on the other hand, the pKa values of HQ and CT are 9.85 and 9.4, respectively. At pH 5.0, –NH2 and –SH groups exposed to the p-ATT layer and the ionized hydroxyl in dihydroxybenzenes increase the adsorption capacity of the three dihydroxybenzene isomers.48 As for AgNC/rGO/p-ATT/CFME, the peak currents of HQ and CT are 5.6 and 3.7 times of the bare CFME (curve d). The excellent performance may attribute to the following reasons:
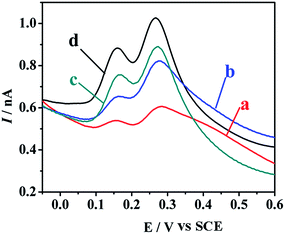 |
| Fig. 5 Square-wave voltammetrys for 300 μmol L−1 HQ and CT in acetate buffer (pH 5.0) on the surface of various electrodes: curve (a) CFME, (b) AgNC/rGO/CFME, (c) p-ATT/CFME, and (d) AgNC/rGO/p-ATT/CFME. | |
(1) p-ATT film is highly conducting and its redox kinetics are fast.44
(2) The high density of localize π electrons in rGO surface would be beneficial for the accumulation of HQ and CT through the strong π–π interactions.
(3) AgNC/rGO can provide larger electrochemical active surface areas and effectively accelerate the electron transfer between electrode and analytes.
In summary, synergistic effect of above reasons makes AgNC/rGO/p-ATT/CFME possible as an electrochemical sensor for simultaneous and sensitive determination of HQ and CT.
3.3. Influence of solution pH and scan rate
The effect of pH on the peak potential and current of HQ and CT at AgNC/rGO/p-ATT/CFME was also investigated in 0.2 mol L−1 acetate buffer solutions by SWV in the pH range of 3.0–7.0. As seen from Fig. 6A, the peak potentials for HQ and CT display a same trend and shift almost linearly toward negative potential when pH increased from 3.0 to 7.0, suggesting that protons have directly taken part in the electrode reaction process of both HQ and CT. Accordingly, HQ is more sensitive to respond oxidation even at low concentration as compared to CT. The maximal peak current for HQ is obtained at pH 6.0, and the maximal peak current for CT is obtained at pH 5.0. In order to determine HQ and CT sensitively, all experiments were carried out at pH 5.0. The dependence of Ep on pH can be represented by the equations (Fig. 6B): |
HQ: Ep = 0.48 − 0.06pH
| (1) |
|
CT: Ep = 0.549 − 0.055pH
| (2) |
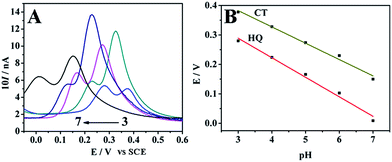 |
| Fig. 6 (A) Square-wave voltammetrys of AgNC/rGO/p-ATT/CFME for 300 μmol L−1 HQ and CT in 0.2 mol L−1 acetate buffer with different pH values; (B) the dependence of peak potentials of HQ and CT on the pH of the buffer at AgNC/rGO/p-ATT/CFME. | |
The two almost parallel lines indicate that the peak potential difference between HQ and CT is constant. According to the following formula:
|
dEp/dpH = −2.303mRT/nF 49
| (3) |
‘
m’ and ‘
n’ are the number of proton and electron, respectively; ‘
m/
n’ is calculated to be 1.01 and 0.93 for the HQ and CT oxidation process, respectively. It means the number of proton and electron involved in the oxidative process of HQ and CT is equal. In addition, the slopes of the regression equations are close to the theory value of 58.5 mV pH
−1 for two electrons and two protons process.
50 The probable electrode reaction is shown in
Scheme 1.
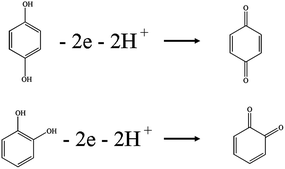 |
| Scheme 1 Mechanism of oxidative reactions of HQ and CT at AgNC/rGO/p-ATT modified CFME. | |
The reaction kinetic was investigated by studying the effects of scan rate dependence toward the oxidation peak current of 300 μmol L−1 HQ and CT in acetate buffer (pH 5.0) (Fig. 7). With the increase of scan rate from 30 to 380 mV s−1, the anodic peak currents increased linearly with the square root of scan rates, following the regression equation:
|
HQ: Ip = 0.10366v1/2 − 0.5296 (R = 0.993)
| (4) |
|
CT: Ip = 0.05863v1/2 − 0.1293 (R = 0.990)
| (5) |
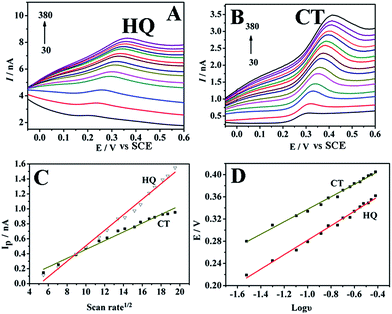 |
| Fig. 7 Influence of scan rate on the peak currents of 300 μmol L−1 HQ (A) and CT (B) at AgNC/rGO/p-ATT/CFME in 0.2 mol L−1 acetate buffer solution (pH 5.0): 30, 50, 80, 100, 130, 150, 180, 200, 230, 250, 280, 300, 330, 350, and 380 mV s−1; (C) plot of peak currents of HQ and CT versus square roots of scan rate; (D) plot of peak potentials of HQ and CT versus logarithm of scan rate. | |
This indicated that the electrode reaction of HQ and CT on AgNC/rGO/p-ATT/CFME was a typical diffusion-controlled process. The Ep shifted positively along with the increase of scan rate, showing a linear relationship with log
v (Fig. 7D), which was further constructed with the equation:
|
HQ: Ep = 0.130 log v + 0.412 (R = 0.993)
| (6) |
|
CT: Ep = 0.112 log v + 0.45 (R = 0.990)
| (7) |
According to the following formula:
|
Ep = A + 2.303RT log v/(1 − α)nF
| (8) |
‘
A’ is a constant; ‘
α’ is the transfer coefficient characterizes; ‘
n’ is the number of electrons involved in the rate-controlling step; ‘
R’, ‘
T’ and ‘
F’ are the gas constant, temperature and Faraday constant, respectively. On the basis of the slope being equal to 2.303
RT/(1 −
α)
nF, the transferred electron was calculated to be 1.58 for HQ and 1.76 for CT (
α = 0.7), suggesting that two electrons were involved in the oxidation reaction. The result was consistent with
Scheme 1.
3.4. Determination of HQ and CT by square-wave voltammetry
Under the optimal conditions, the determination of HQ and CT at AgNC/rGO/p-ATT/CFME was carried out by SWV, where the concentration of one species changed while the other one remained constant. As shown in Fig. 8A, keeping the concentration of CT constant (30 μmol L−1), the oxidation peak current increases linearly with increasing the concentration of HQ in the range of 0.08–1000 μmol L−1. The regression equation is described as: |
log Ip = 0.725 log C − 0.88752 (R = 0.997)
| (9) |
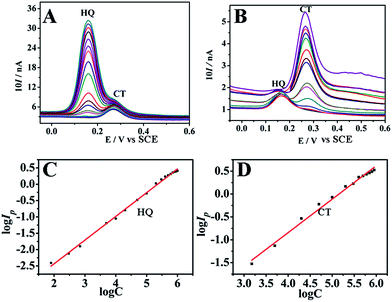 |
| Fig. 8 Square-wave voltammetrys at AgNC/rGO/p-ATT/CFME in 30 μmol L−1 CT and different concentrations of HQ: 0.08, 0.3, 0.7, 5, 10, 20, 50, 100, 200, 300, 400, 500, 600, 700, 800, 900, and 1000 μmol L−1 (A); (B) 30 μmol L−1 HQ and different concentrations of CT: 1.5, 5, 20, 50, 100, 200, 300, 400, 500, 600, 700, 800, and 900 μmol L−1; calibration curves from logarithm of the current response at AgNC/rGO/p-ATT/CFME vs. logarithm of concentrations of HQ (C) and CT (D) in acetate buffer solutions (pH 5.0). | |
Similarly, as shown in Fig. 8B, the peak current linearly increases with increasing concentration of CT from 1.5 to 900 μmol L−1, while keeping the concentration of HQ constant at 30 μmol L−1. The regression equation is described as:
|
log Ip = 0.731 log C − 3.7683
| (10) |
The determination limits were calculated from the calibration curve using the 3sb/m, where sb is the standard deviation of the intercept and m is the slope of the calibration graph.51 The detection limits of HQ and CT are shown in Table 1. The peak current of CT kept almost constant as HQ concentration was increased, further confirming that this modified electrode can be employed for the simultaneous determination of HQ and CT without interference with each other. The present results are compared to those in literatures (Table 2). It shows that the linear range of HQ and CT at the present work are much wider, and the detection limit is lower than that in previously published works. We used CFME to determine HQ and CT, which can be used in vivo. The RSD of regression equation is similar with other sensors, which demonstrates the good linear relationship between logarithm of concentrations and logarithm of the current.
Table 1 Results obtained from the linear regression
|
HQ |
CT |
sb |
0.002 |
0.031 |
m |
0.725 |
0.731 |
Detection limit (μmol L−1) |
0.0094 |
0.13 |
Table 2 Performance comparison of the fabricated electrode for HQ and CT detection with other electrodes
Electrode |
Linear range (μmol L−1) |
LOD (μmol L−1) |
RSD |
Ref. |
HQ |
CT |
HQ |
CT |
HQ |
CT |
IL-rGO: ionic liquids-functionalized graphene. PANI: polyaniline. PEDOT: poly(3,4-ethylenedioxy-thiophene). PARS: polymeric alizarin red S. PCV: poly(crystal violet). Pyridinic-rGO: pyridinic nitrogen doped graphene. |
IL-rGOa/GCE |
1–300 |
8.0–391 |
0.85 |
2.6 |
— |
— |
32 |
rGO-MWNTs/GCE |
2–400 |
5.55–40 |
1.0 |
1.8 |
0.994 |
0.992 |
33 |
Carbon electrode |
2–50 |
5–50 |
2 |
5 |
0.996 |
0.997 |
35 |
PANIb/MnO2/GCE |
0.2–100 |
0.2–100 |
0.13 |
0.16 |
0.997 |
0.997 |
52 |
PEDOTc/GO/GCE |
25–200 |
2–400 |
1.6 |
1.6 |
0.998 |
0.999 |
53 |
PARSd/CS/BCN-GO/GCE |
1–100 |
1–100 |
0.19 |
0.11 |
0.997 |
0.997 |
54 |
PDAe-rGO/GCE |
1–250 |
1–230 |
0.62 |
0.74 |
0.996 |
0.999 |
55 |
Pyridinic-rGOf/GCE |
5–30; 30–200 |
5–200 |
0.38 |
1 |
0.996 |
0.998 |
56 |
CdS/rGO/GCE |
0.2–2300 |
0.5–1350 |
0.054 |
0.09 |
0.995 |
0.997 |
57 |
AgNC/rGO/p-ATT/CFME |
0.08–1000 |
1.5–900 |
0.0094 |
0.13 |
0.997 |
0.991 |
This work |
3.5. Reproducibility, stability of AgNC/rGO/p-ATT/CFME and interference studies
Besides the sensitivity and selectivity, reproducibility and stability are important parameters to evaluate the applicability of electrochemical sensor. To characterize the reproducibility of the AgNC/rGO/p-ATT/CFME, 6 consecutive measurements were done for 300 μmol L−1 HQ and CT at the same electrode. The relative standard deviation (RSD) was 0.9% and 2.1% for HQ and CT, revealing that the AgNC/rGO/p-ATT/CFME sensing device had good reproducibility. The long term stability of the AgNC/rGO/p-ATT/CFME sensing device was also examined by measuring SWV peak currents of 300 μmol L−1 HQ and CT after storing the electrode for 10 days. After 10 days, the AgNC/rGO/p-ATT/CFME sensing device retained 91% of the original values, demonstrating that the sensing device had better long term stability. These results revealed the appreciable reproducibility and good stability of the proposed sensor. The possible interferences of some inorganic ions and organic compounds were investigated with a mixed dihydroxybenzens solution (HQ and CT, each 300 μmol L−1) by SWV. It was found that 100-fold Na+, K+, NH4+, Mg2+, Cl−, SO42−, glycine, ethanol, glucose and ascorbic acid, 10-fold Ca2+, Fe2+, Cu2+, H2PO4− and 2-fold nitrophenol, resorcinol and phenol did not interfere with the determination (signals change below 5.0%), indicating good antiinterference ability of AgNC/rGO/p-ATT modified CFME.
3.6. Water samples analysis
To demonstrate the practical application of AgNC/rGO/p-ATT/CFME for the simultaneous determination of HQ and CT, local lake water and tap water samples were tested. The determination of the HQ and CT concentration was performed by the standard addition method. The lake water samples were obtained from South Lake (Changchun, China), and they were determined after certain volume of pH 5.0 acetate buffer was introduced. The obtained results are listed in Table 3, which indicate the direct determination of HQ and CT in water is possible after dilution of the sample with the supporting electrolyte.
Table 3 Recovery of HQ and CT in different water samples at AgNC/rGO/p-ATT/CFME
Sample |
Added (μmol L−1) |
Founda (μmol L−1) |
Recovery (%) |
HQ |
CT |
HQ |
CT |
HQ |
CT |
Average of three determinations. |
Tap water |
50.0 |
70.0 |
48.0 |
70.8 |
96.0 |
101.1 |
Tap water |
100.0 |
120.0 |
98.4 |
122.2 |
98.4 |
101.8 |
Lake water |
60.0 |
70.0 |
61.4 |
72.2 |
102.3 |
103.1 |
Lake water |
100.0 |
130.0 |
102.5 |
133.1 |
102.5 |
102.4 |
4. Conclusions
In order to prepare Ag/AgCl nanocubes/reduced graphene oxide, we used AgCl as crystal seed. Uniformly dispersed Ag/AgCl nanocubes were successfully obtained on rGO using chitosan as reductant, employing AgNO3 and GO as starting materials in the presence of HCl. The reduction of GO and the generation of the Ag/AgCl nanocubes occurred simultaneously. The merit of this method lies in its low-cost, non use of toxic agent and easily scalable. Ag/AgCl nanocubes/reduced graphene oxide showed high stability and dispersity in water and large surface area, and had been successfully applied in catalytic performance toward the oxidation of HQ and CT. AgNCs/rGO/p-ATT/CFME showed a wide linear concentration range (0.08–1000 μmol L−1 for HQ and 1.5–900 μmol L−1 for CT) and a low detection limit (0.0094 μmol L−1 for HQ and 0.13 μmol L−1 for CT), indicating that AgNC/rGO/p-ATT/CFME could be utilized as a promising sensing platform for the determination of HQ and CT.
Acknowledgements
This work was supported by the NNSFC (no. 21375045) and NSF of Jilin Province (no. 20130101118JC).
References
- B. Wiley, Y. Sun and Y. Xia, Acc. Chem. Res., 2007, 40, 1067–1076 CrossRef CAS PubMed.
- Z. Xu, Y. Hou and S. Sun, J. Am. Chem. Soc., 2007, 129, 8698–8699 CrossRef CAS PubMed.
- H. Wang, X. Zheng, J. Chen, D. Wang, Q. Wang, T. Xue, C. Liu, Z. Jin, X. Cui and W. Zheng, J. Phys. Chem. C, 2012, 116, 24268–24273 CAS.
- P. Christopher, H. Xin and S. Linic, Nat. Chem., 2011, 3, 467–472 CAS.
- M. Lv, S. Su, Y. He, Q. Huang, W. Hu, D. Li, C. Fan and S. T. Lee, Adv. Mater., 2010, 22, 5463–5467 CrossRef CAS PubMed.
- P. Gunawan, C. Guan, X. Song, Q. Zhang, S. S. J. Leong, C. Tang, Y. Chen, M. B. Chan-Park, M. W. Chang, K. Wang and R. Xu, ACS Nano, 2011, 5, 10033–10040 CrossRef CAS PubMed.
- J. Cui, C. Hu, Y. Yang, Y. Wu, L. Yang, Y. Wang, Y. Liu and Z. Jiang, J. Mater. Chem., 2012, 22, 8121–8126 RSC.
- J. D. Kim, T. Palani, M. R. Kumar, S. Lee and H. C. Choi, J. Mater. Chem., 2012, 22, 20665–20670 RSC.
- A. K. Geim, Science, 2009, 324, 1530–1534 CrossRef CAS PubMed.
- F. Schedin, A. Geim, S. Morozov, E. Hill, P. Blake, M. Katsnelson and K. Novoselov, Nat. Mater., 2007, 6, 652–655 CrossRef CAS PubMed.
- M. I. Katsnelson, Mater. Today, 2007, 10, 20–27 CrossRef CAS.
- K. S. Kim, Y. Zhao, H. Jang, S. Y. Lee, J. M. Kim, K. S. Kim, J. H. Ahn, P. Kim, J. Y. Choi and B. H. Hong, Nature, 2009, 457, 706–710 CrossRef CAS PubMed.
- R. Pasricha, S. Gupta and A. K. Srivastava, Small, 2009, 5, 2253–2259 CrossRef CAS PubMed.
- J. Liu, S. Fu, B. Yuan, Y. Li and Z. Deng, J. Am. Chem. Soc., 2010, 132, 7279–7281 CrossRef CAS PubMed.
- X. Qin, Y. Luo, W. Lu, G. Chang, A. M. Asiri, A. O. Al-Youbi and X. Sun, Electrochim. Acta, 2012, 79, 46–51 CrossRef CAS PubMed.
- S. Liu, L. Wang, J. Tian, Y. Luo, X. Zhang and X. Sun, J. Colloid Interface Sci., 2011, 363, 615–619 CrossRef CAS PubMed.
- L. Huang, K. Hou, X. Jia, H. Pan and M. Du, Mater. Sci. Eng. C, 2014, 38, 39–45 CrossRef CAS PubMed.
- Q. Liu, X. Zhang, G. Wen, Y. Luo, A. Liang and Z. Jiang, Plasmonics, 2015, 10, 285–295 CrossRef CAS.
- J. Tang, D. Tang, B. Su, Q. Li, B. Qiu and G. Chen, Electrochim. Acta, 2011, 56, 8168–8175 CrossRef CAS PubMed.
- S. Qi, X. Shen, Z. Lin, G. Tian, D. Wu and R. Jin, Nanoscale, 2013, 5, 12132–12135 RSC.
- P. Yang, L. Wang, Q. Wu, Z. Chen and X. Lin, Sens. Actuators, B, 2014, 194, 71–78 CrossRef CAS PubMed.
- J. Yang, W. D. Zhang and S. Gunasekaran, Biosens. Bioelectron., 2010, 26, 279–284 CrossRef CAS PubMed.
- J. Geng, Y. Bi and G. Lu, Electrochem. Commun., 2009, 11, 1255–1258 CrossRef CAS PubMed.
- M. Zhu, P. Chen and M. Liu, Langmuir, 2013, 29, 9259–9268 CrossRef CAS PubMed.
- H. V. Tran, L. D. Tran, C. T. Ba, H. D. Vu, T. N. Nguyen, D. G. Pham and P. X. Nguyen, Colloids Surf., A, 2010, 360, 32–40 CrossRef CAS PubMed.
- Y. Guo, X. Sun, Y. Liu, W. Wang, H. Qiu and J. Gao, Carbon, 2012, 50, 2513–2523 CrossRef CAS PubMed.
- X. Zhang, S. Duan, X. Xu, S. Xu and C. Zhou, Electrochim. Acta, 2011, 56, 1981–1987 CrossRef CAS PubMed.
- Y. G. Sun, H. Cui, Y. H. Li and X. Q. Lin, Talanta, 2000, 53, 661–666 CrossRef CAS.
- H. Cui, C. X. He and G. W. Zhao, J. Chromatogr. A, 1999, 855, 171–179 CrossRef CAS.
- P. Nagaraja, R. A. Vasantha and K. R. Sunitha, J. Pharm. Biomed. Anal., 2001, 25, 417–424 CrossRef CAS.
- M. F. Pistonesi, M. S. Di Nezio, M. Centurion, M. E. Palomeque, A. G. Lista and B. S. F. Band, Talanta, 2006, 69, 1265–1268 CrossRef CAS PubMed.
- C. Wang, Y. Chen, K. Zhuo and J. Wang, Chem. Commun., 2013, 49, 3336–3338 RSC.
- F. Hu, S. Chen, C. Wang, R. Yuan, D. Yuan and C. Wang, Anal. Chim. Acta, 2012, 724, 40–46 CrossRef CAS PubMed.
- X. Feng, Y. Shi and Z. Hu, Mater. Chem. Phys., 2011, 131, 72–76 CrossRef CAS PubMed.
- X. Hu, J. Li and J. Wang, Electrochem. Commun., 2012, 21, 73–76 CrossRef CAS PubMed.
- Y. Zhang, R. Sun, B. Luo and L. Wang, Electrochim. Acta, 2015, 156, 228–234 CrossRef CAS PubMed.
- D. Song, J. Xia, F. Zhang, S. Bi, W. Xiang, Z. Wang, L. Xia, Y. Xia, Y. Li and L. Xia, Sens. Actuators, B, 2015, 206, 111–118 CrossRef CAS PubMed.
- M. Nazari, S. Kashanian and R. Rafipour, Spectrochim. Acta, Part A, 2015, 145, 130–138 CrossRef CAS PubMed.
- L. Yang, S. Fan, G. Deng, Y. Li, X. Ran, H. Zhao and C. P. Li, Biosens. Bioelectron., 2015, 68, 617–625 CrossRef CAS PubMed.
- B. Kaur, T. Pandiyan, B. Satpati and R. Srivastava, Colloids Surf., B, 2013, 111, 97–106 CrossRef CAS PubMed.
- D. C. Marcano, D. V. Kosynkin, J. M. Berlin, A. Sinitskii, Z. Sun, A. Slesarev, L. B. Alemany, W. Lu and J. M. Tour, ACS Nano, 2010, 4, 4806–4814 CrossRef CAS PubMed.
- M. Kathiwala, A. O. Affum, J. Perry and A. Brajter-Toth, Analyst, 2008, 133, 810–816 RSC.
- T. M. Oliveira, H. Becker, E. Longhinotti, D. De Souza, P. de Lima-Neto and A. N. Correia, Microchem. J., 2012, 109, 84–92 CrossRef PubMed.
- P. Kalimuthu and S. A. John, Electrochem. Commun., 2009, 11, 367–370 CrossRef CAS PubMed.
- D. Li, M. B. Muller, S. Gilje, R. B. Kaner and G. G. Wallace, Nat. Nanotechnol., 2008, 3, 101–105 CrossRef CAS PubMed.
- M. Fang, L. A. Long, W. F. Zhao, L. W. Wang and G. H. Chen, Langmuir, 2010, 26, 16771–16774 CrossRef CAS PubMed.
- M. R. C. Massaroppi, S. A. S. Machado and L. A. Avaca, J. Braz. Chem. Soc., 2003, 14, 113–119 CrossRef CAS PubMed.
- P. Kalimuthu and S. A. John, Bioelectrochemistry, 2009, 77, 13–18 CrossRef CAS PubMed.
- E. Laviron, J. Electroanal. Chem. Interfacial Electrochem., 1974, 52, 355–393 CrossRef CAS.
- B. J. Sanghavi and A. K. Srivastava, Electrochim. Acta, 2010, 55, 8638–8648 CrossRef CAS PubMed.
- P. N. Bartlett, E. Ghoneim, G. El-Hefnawy and I. El-Hallag, Talanta, 2005, 66, 869–874 CrossRef CAS PubMed.
- M. U. Anu Prathap, B. Satpati and R. Srivastava, Sens. Actuators, B, 2013, 186, 67–77 CrossRef CAS PubMed.
- W. Si, W. Lei, Y. Zhang, M. Xia, F. Wang and Q. Hao, Electrochim. Acta, 2012, 85, 295–301 CrossRef CAS PubMed.
- J. Qu, Y. Wang, J. Guo, Y. Dong and T. Lou, J. Electrochem. Soc., 2014, 161, B220–B224 CrossRef CAS PubMed.
- L. Zheng, L. Xiong, Y. Li, J. Xu, X. Kang, Z. Zou, S. Yang and J. Xia, Sens. Actuators, B, 2012, 177, 344–349 CrossRef PubMed.
- H. L. Guo, S. Peng, J. H. Xu, Y. Q. Zhao and X. Kang, Sens. Actuators, B, 2014, 193, 623–629 CrossRef CAS PubMed.
- S. Hu, W. Zhang, J. Zheng, J. Shi, Z. Lin, L. Zhong, G. Cai, C. Wei, H. Zhang and A. Hao, RSC Adv., 2015, 5, 18615–18621 RSC.
|
This journal is © The Royal Society of Chemistry 2015 |